-
PDF
- Split View
-
Views
-
Cite
Cite
Anet Režek Jambrak, Marinela Nutrizio, Josipa Dukić, Ilija Djekić, Marko Vinceković, Slaven Jurić, Gianpiero Pataro, Brijesh Tiwari, Gulden Goksen, Mojca Čakić Semenčić, Anita Slavica, Iva Sabljak, Janko Diminić, Ena Pecina, Andrija Sabol, Anne-Sylvie Fabiano Tixier, Francesco Donsi, Digitalisation, bioinformatics, and delivery systems in sustainable nonthermal extraction of proteins, International Journal of Food Science and Technology, Volume 60, Issue 1, January 2025, vvae038, https://doi.org/10.1093/ijfood/vvae038
- Share Icon Share
Abstract
Sustainable development integrates social, economic, technological, scientific, and environmental challenges through the framework of 17 Sustainable Development Goals. Industry 4.0, digitalisation, plant-based processing, environmental challenges, and alternative protein sources are vastly researched and present the foundation of innovative processing in the food processing industry. The exploitation of agroindustrial discards and plant-based by-products as an alternative source of valuable nutrients, including proteins, highlights the potential of upcycling (new economic value) while addressing sustainability challenges. Within the idea of nonthermal extraction of proteins and its process digitalisation, it is crucial to innovate, connect elements of Industry 4.0 and valorise the term sustainability. Innovative approaches in extractions such as ultrasound, plasma, pulsed electric fields, high-intensity pulses, and others, have an energy-saving effect with limited use of toxic chemicals and/or solvents. Usage of software for solvent selection, incorporating digitalisation, and development of protein and peptide databases can help in the prediction, optimisation, and efficiency of nonthermal extractions of proteins from agroindustrial discards. It is also important to select optimal delivery systems like electrospinning, electrospraying, or encapsulation as useful and efficient processes. The environmental, economic, and societal impact of innovative processing needs to be monitored and valorised using specific tools. Life cycle assessment methodology assesses the environmental impact throughout the life cycle of a commercial product, service, or process. The current review shows that extracting protein from agroindustrial discards and by-products of plant and animal origins is a complex matter. The need for a comprehensive approach that considers various viewpoints on extraction is suggested.
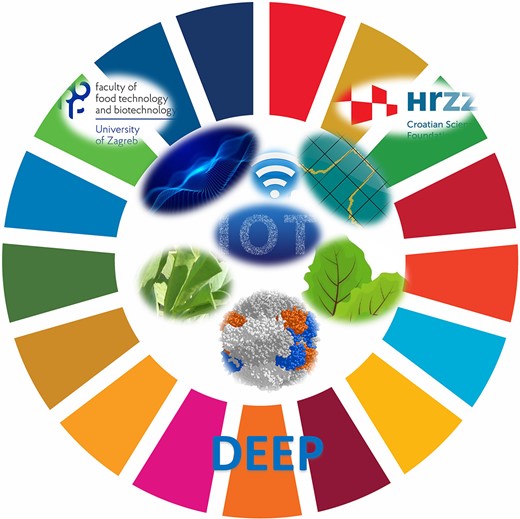
Introduction
The global demand for sustainable protein sources is steadily rising due to factors like population growth, environmental concerns in protein production, and health considerations regarding proteins of animal origin (Henchion et al., 2017). Proteins are increasingly used in developing new food items like vegan foods and protein beverages (Pojić et al., 2018), and they are extracted from biomass using alternative solvents (Kopilovic et al., 2023).
It is also important to address future protein supply and demands and overview possible technologies in the extraction and processing of natural source–derived proteins through eco-innovative approaches (Franca-Oliveira et al., 2021). Beyond nutrition, proteins significantly impact food qualities during preparation, processing, and storage through their techno-functional properties such as solubility, gelling, emulsifying, and foaming abilities (Taha et al., 2022). These properties are influenced by the structural characteristics of proteins, including secondary, tertiary, and quaternary structures, which are affected by factors like denaturation or aggregation due to intrinsic (primary structure, hydrophilic/hydrophobic nature, net charge) and extrinsic factors (potential of Hydrogen (pH), ionic strength, temperature, interactions with other food components). Food processing can also affect protein functionality by altering the native protein structure reversibly (unfolding) or irreversibly (denaturation), depending on the processing technology and conditions used (Yan et al., 2024).
Sustainable development conveys a profound message to humanity, underscoring the imperative of forging new foundational principles essential for fostering enduring and sustainable progress towards a shared future. This necessitates a paradigm shift away from the predominant emphasis on economic and financial considerations as the primary, if not exclusive, drivers, objectives, and benchmarks of development thus far. Instead, it calls for prioritising the ecological and social dimensions, along with their respective goals and criteria, alongside economic considerations. It is of utmost importance to address and valorise sustainability parameters in the food supply chain and food-related sustainable dimensions (Hassoun et al., 2023). As a very important aspect of current research and future application in the food industry is sustainability, it is necessary to implement Sustainable Development Goals (SDGs) in research activities. Waste, energy, economy, and environment are major pillars that need to be managed in terms of Agenda 2030 and SDGs. Energy conservation is vital for the sustainable development of the food industry.
A crucial aspect is the development of Internet of Things (IoT) platforms, integrating workforce practices into manufacturing activities (Martindale et al., 2023), usage of nonthermal extraction from plant waste (Nutrizio et al., 2022), and alternative protein sources (Rivero Meza et al., 2023) for a sustainable food system of plant origin (Munialo, 2024a).
Application of Industry 4.0 is having a strong merge into the food production industry. Nonthermal processing (NTP) technologies have been researched and investigated for more than 20 years, as promising and emerging technologies (Jambrak, 2022). NTP has been applied in food product stabilisation and extraction of valuable compounds. Several nonthermal techniques can be used as alternative preservation processes to the conventional application of thermal treatments and/or pretreatments. Due to oxidative activity like in plasma usage, nonthermal processing can be used in wastewater treatments and different applications (e.g., plasma-activated water). Nonthermal technologies include electro technologies (plasma processing, high-voltage electrical discharges [HVEDs], pulsed electric fields [PEFs], pulsed light [PL], e-beam); pressure-based technologies (high-pressure processing [HPP], high-pressure homogenisation [HPH]); and mechanical technologies (high-power ultrasound [US], hydrodynamic cavitation). There is a need to reach a technology readiness level (TRL), to be applied in the food industry. Two technologies already in use in the food industry are PEF and HPP. Those technologies have a TRL level more than 7 (Ebert & Aganovic, 2022).
Nonthermal extraction methods have gained significant attention in recent years due to their ability to preserve the integrity of proteins and other bioactive compounds during extraction (Singh & Sharma, 2023). These methods enhance mass transfer rates, cell permeability, and secondary metabolite diffusion, leading to higher extraction yields.
With the current state of the art for Industry 4.0 and smart factories accordingly, there are new opportunities to implement elements of Industry 4.0 in NTP. Industry 4.0 includes automation and data exchange in manufacturing technologies and includes cyber-physical systems, IoT, advanced connectivity, and cloud computing to transform production units into “smart factories” (Jambrak et al., 2021).
The demand for plant-based proteins continues to grow, and there is a corresponding increase in research and development efforts to optimise processing techniques and ensure compliance with regulatory requirements (Ivanov & Munialo, 2023; Munialo, 2024b; Rout et al., 2024). This involves not only improving the efficiency of protein extraction but also addressing safety concerns and meeting consumer expectations regarding taste, texture, and nutritional quality. Although nonthermal green extraction techniques are important for food safety and sustainability, consumers are often hesitant to accept them.
In performing green extraction of proteins from agroindustrial discards and plant-based by-products, a great opportunity is in the extraction of proteins from residues, from leaves, as they are usually discarded in the factory. The focus is on reducing, recycling, and reusing, towards zero-waste processing. RuBisCO (Ribulose-1,5-bisphosphate carboxylase/oxygenase) is the most abundant protein found on earth, as it can comprise up to 50% of the total soluble protein found in leaf tissue or within specific microbes (Dukić et al., 2023). Due to the increasing demand for highly nutritious foods, the global tendency is to replace animal proteins with plant proteins, which are more sustainable and profitable. The focus is on soluble protein RuBisCO, which has high nutritional values and in vitro digestibility.
Due to the similarity of the amino acid composition in humans, animal proteins have a significantly higher biological value (egg protein—gold standard/reference 100%) than plant proteins. In terms of digestibility, plant proteins are not significantly inferior to animal proteins. According to the available studies, RuBisCO isolated from alfalfa has a sequence coverage of 77%–99% in vitro in the gastric phase, regardless of the type of processing and the degree of purity (Tanambell et al., 2024).
Upon extraction from agroindustrial discards and plant-based by-products, extracts can be “packed” or processed using different procedures like electrospinning and electrospraying (to obtain nanofibres), or encapsulated, as the usual procedure of delivering extracted bioactive compounds. Electrospinning is an efficient technique for nanofibre production, from polymeric solutions that produce continuous jets that elongate forming the nanomaterial, under the action of the electric field (Jurić et al., 2024). In obtaining nanofibres by the electrospinning process, the application of high temperatures is not necessary since electrostatic forces are used to produce the fibres. This is the most important segment, as a complete extraction procedure will be performed below 35 °C, to avoid protein denaturation.
Application of NTP is usually focused on specific and target usage while assuring preservation (food safety). Application of the Internet of nonthermal food processing technologies (IoNTP) can be focused on food quality monitoring and total quality assessment with the lowest energy and economic resources. The idea is to use smart and sustainable technologies with the least impact on the environment and a positive impact on society. The three pillars of sustainability refer to environmental, economic, and social impacts of nonthermal technologies (i.e., energy balances, life cycle assessment [LCA], waste production/reduction, cost of production, and impact on society) as well as a set novel approach to introduce nonthermal technologies as smart systems in food industries. To determine sustainability, quantitative data must be acquired, with the most common method of LCA. LCA is the primary and most essential tool to support decision-making in sustainability (Schottroff et al., 2022).
Biological databases play a crucial role in the field of biological research. They serve as repositories for a vast amount of biological data generated from scientific experiments, most importantly DNA and protein sequences and protein structures (Christensen & de Vries, 2023). The importance of these databases lies in their ability to store, organise, and make these enormous data readily accessible for researchers, facilitating easy retrieval of information, and thus saving time and resources (Selvakumar et al., 2024). By providing a platform for storing and organising the vast amount of biological data, and facilitating the analysis and interpretation of this data, bioinformatics databases significantly support multiscale and multidisciplinary studies. This cross-disciplinary application further underscores the importance and versatility of these databases in advancing scientific research (Selvakumar et al., 2024).
Upon extraction of proteins using nonthermal technologies, several peptides and intermediaries can occur (Hemker et al., 2020).
While there are numerous databases available for protein and peptide data, specific databases dedicated to the nonthermal extraction of proteins are currently limited. Most researchers in the field rely on general protein databases, such as UniProt and Protein Data Bank (PDB), and apply the data to their specific nonthermal extraction studies (Bateman et al., 2023; Zardecki et al., 2022). The data available in these databases typically include protein sequences, structures, and functional information. For nonthermal extraction studies, the data can provide valuable insights into the properties of the extracted proteins, such as their stability under nonthermal conditions, their interactions with other compounds, and their potential bioactivities (Franca-Oliveira et al., 2021). The available protein databases provide a wealth of data that can be applied to nonthermal extraction studies. The development of more specialised databases would further enhance the field by providing more targeted data for researchers.
To our knowledge, there is no information on NTP in combination with in silico prediction of the intermediate pathway of peptide occurrence/production, during or after nonthermal protein extraction. Therefore, the aim of this review paper is to overview the state of the art of digitalisation, bioinformatics, and delivery systems in sustainable nonthermal extraction of proteins.
Digitalisation
Industry 4.0 includes automation and data exchange in manufacturing technologies and includes cyber-physical systems, IoT, advanced connectivity, and cloud computing to transform production units into “smart factories.” Digitalisation means the transition from an industrial age characterised by analogue technologies to an age of knowledge and creativity characterised by digital technologies. The process of making digital everything that can be digitised. The idea of future nonthermal protein extraction is to use digitalisation in the NTP set-up (with sensors and IoT), to monitor and optimise the extraction process (Jambrak et al., 2021). Moreover, with the application of IoT, collection of in-line data, smart control of the process, and big data optimisation, as well as sustainable production and monitoring, there is the possibility to develop a new era of IoNTP.
The IoT is a system of interrelated computing devices, mechanical and digital machines, objects, or people who are provided with unique identifiers and the ability to transfer data over a network without requiring human-to-human or human-to-computer interaction. An IoT ecosystem consists of web-enabled smart devices that use embedded systems, such as processors, sensors, and communication hardware, to collect, send, and act on data they acquire from their environments (Hassoun et al., 2022a). Extraction by NTP technologies is a very researched area. There are several research articles about applying NTP and the usage of green solvents, in improving the extraction yield of desired compounds (Chemat et al., 2020). Previously, conventional technologies were using nonenvironmentally friendly solvents, which are high energy demanding and use very long duration time for extraction. Green extraction has many advantages such as increasing the yield of bio-active products, extraction and solubilisation of substances in water or green solvents at room temperature instead of using organic solvents, reducing extraction time, and simplifying purification steps. NTP has been used for the extraction of bioactive compounds from plant-based materials because of their potential to facilitate the mass transfer process during conventional solid–liquid extraction (Nutrizio et al., 2020).
Role of digitalisation in data analysis
Digitalisation has significantly transformed the landscape of data analysis. The advent of digital tools, artificial intelligence, and big data has led to a new era of data-driven innovation (Sundu et al., 2022). The digitisation of the economy and society has led to greater data availability, which has, in turn, increased the importance of automated learning and the analysis of data for organisations (Calderon-Monge et al., 2023). The ability to collect, store, and analyse large volumes of data has become more cost-effective and efficient, leading to transformative changes within firms and their immediate business environment (Calderon-Monge et al., 2023).
Digitalisation has enabled the development of advanced data analysis tools and techniques. For instance, machine learning algorithms can now process and analyse large datasets to extract meaningful insights and patterns that were previously unattainable. These advancements have opened up new opportunities for innovation and have had a profound impact on various fields, including bioinformatics (Calderon-Monge et al., 2023).
Looking ahead, the role of digitalisation in data analysis is expected to continue to grow. The ongoing advancements in digital technologies, coupled with the increasing availability of data, suggest that the future of data analysis will be heavily influenced by digitalisation (Nadkarni & Prügl, 2021). One key area of future research is the development of new digital technologies and data analysis techniques. As digital technologies continue to evolve, so will the tools and techniques for data analysis. This will likely lead to the emergence of new methodologies for data mining and predictive modelling, among others. Another important future perspective is the integration of digitalisation with other technological trends. For instance, the convergence of digitalisation with technologies like artificial intelligence and blockchain could lead to the development of new data analysis paradigms (Nadkarni & Prügl, 2021).
IoT and sensors
The IoT is a system of interconnected computing devices that are tagged with unique identifiers and can transmit data over a network. The most popular languages in IoT are Java, C, C++, Python, JavaScript, Node.js, Assembler, PHP, C#, Lua, R, Go, Ruby, Swift, Rust, etc. Therefore, the choice of programming language depends on the capabilities and purpose of the device (sensor, central processing unit, etc.) (Arslan et al., 2023). For the application of NTP technologies, specifically, US and PEF equipment can be equipped with different sensors and transformed into IoT devices. Optimisation can be monitored by an experimental design and digitalisation of green extraction procedure can be, in the future, implemented in the “smart processing” line. Monitoring of extractions can be obtained through the protein yield (RuBisCO) and antioxidant potential (leaves—plant protein sources). In the extraction procedure by NTP, it is possible to obtain peptides, and, for that, the data collection is the ultimate step leading to big data analysis. Data collection is managed through IoT equipped with sensors, computers, and software that are incorporated in the NTP sequence. Each experimental setup can be equipped with additional state-of-the-art sensors for accurate real-time process measurements and data acquisition. Sensor data can be transmitted in real-time via local area network (LAN) resources. Different types of data can be collected like temperature values, pH, conductivity, oxido-reduction potential, pressure, energy consumption, gas emissions, etc. The acquired sensor data will be first read by a central controller, which will forward the collected data to a database or cloud. By fully monitoring the process in real time, the test data are globally available for further analysis and adjustment of test parameters. In addition, some experimental units (PEF devices) already have integrated process sensors connected to a central processing unit. These central processing units can be upgraded and connected to the IoT system.
Communication technologies for IoT deployment include short-range and long-range communication technology standards. Examples of short-range communication technologies include ZigBee, Bluetooth, and ultra-wideband systems, while long-range communication can use wireless local-area network WLAN/LAN, short for long-range wide area networking (LoRaWAN), fibre optics, and wireless cellular networks. LoRaWAN is a low-power, wide-area networking protocol designed to connect battery-operated “things” to the internet in regional, national, or global networks (Pons et al., 2023). Depending on the local communication resources, some type of long-range communication can be used to establish the connection between the sensors and the IoT system.
Nonthermal processing extraction: focus on proteins
Proteins
Proteins are large biomolecules and macromolecules that comprise one or more long chains of amino acid residues. Proteins perform a vast array of functions within organisms. Nine essential amino acids cannot be synthesised in the body and must be taken from food. Proteins can be obtained from plant and animal sources. Animal-based foods have a higher carbon footprint than plant-based, so the focus is on replacing animal proteins with plant-based proteins (Chandran et al., 2023). Human diets high in plant protein are linked to many benefits. Previous research studies have observed that plant-based proteins (leguminous, cereal, oilseed) are incorporated as protein supplements (de Jong et al., 2024). Proteins in plants are strongly tied in the cell wall, so efficient technology must disrupt plant cells and enable extraction.
Plant-based proteins have been gaining popularity as alternatives to animal-derived proteins due to various reasons, such as health benefits, environmental sustainability, and ethical considerations. However, introducing these novel sources of protein into the market often involves navigating regulatory frameworks, particularly in regions like the European Union (EU) where there are strict regulations regarding novel foods. Plant-based proteins are important for food production and the production of biodegradable and edible food packaging. Scientists are targeting to improve nonthermal green extraction techniques (US, microwave, enzyme assisted, PEF, etc.) in the field of protein extraction efficiency and reducing protein denaturation (Tang et al., 2024).
Novel food regulations are designed to ensure the safety of consumers while encouraging innovation in the food industry. When it comes to plant-based proteins, they may fall under the category of novel foods if they have not been widely consumed in the EU before May 1997. This means that companies seeking to market plant-based proteins need to undergo thorough safety assessments and obtain approval from the relevant regulatory authorities before they can be sold to consumers.
Ribulose-1,5-bisphosphate carboxylase-oxygenase
RuBisCO and Rubisco are just some of the names for the enzyme ribulose-1,5-bisphosphate carboxylase-oxygenase, the most abundant enzyme on Earth. It is estimated to have originated 3 billion years ago when the atmosphere had a higher concentration of carbon dioxide and a lower concentration of oxygen (Erb & Zarzycki, 2018). During the gradual adaptation to the changed atmospheric conditions to the current enzyme structure, complex reaction mechanisms were retained that arose under different, historical conditions. It has a very large molecular weight of 560 kDa, making RuBisCO one of the largest enzymes in nature. It is found in most autotrophic organisms, including terrestrial and aquatic plants, eukaryotic algae, cyanobacteria, and photosynthetic bacteria (Zhao et al., 2024). There are four organisational structures of RuBisCO (I, II, III, and IV), and all of them are multimeric and consist of several subunits. RuBisCO form I consists of eight large subunits (L) and eight small subunits (S), often represented as L8S8. The large subunit (50–55 kDa) contains most of the enzyme’s active sites and is also known as the catalytic subunit. The smaller subunit has a molecular weight of 12–18 kDa and is not essential for the catalytic activity of the enzyme (Martin et al., 2019).
The enzyme itself belongs to the group of water-soluble proteins, more precisely to the proteins of fraction 1 (F-1-p). In plants, it accumulates in the chloroplast and is involved in the processes of photosynthesis and photorespiration. It is a key enzyme that catalyses the first and limiting step of photosynthesis, in which the carboxylation of ribulose-1,5-bisphosphate (RuBP) takes place, i.e., the assimilation of carbon dioxide, forming two molecules of 3-phosphoglycerate. RuBisCO catalyses not only the RuBP carboxylation reaction but also the photorespiration reaction, i.e., the oxygenation of RuBP, making it an extremely inefficient catalytic enzyme of photosynthesis (Waheeda et al., 2023). To compensate for its low efficiency, plants accumulate a considerable amount of the enzyme in their leaves, making RuBisCO the most abundant protein on Earth.
Very good amino acid composition and functional properties make RuBisCO an interesting protein for the development of new and the enrichment of existing food products. It has good solubility, which varies depending on the plant species from which it was isolated. In addition, its emulsifying capacity increases with increasing pH and high-temperature pretreatments. The foaming ability increases as the isoelectric value (pI) of the protein is approached. Since the electrostatic interactions between the proteins are minimal at pI, and the layer of protein molecules at the water–air interface has the highest density, RuBisCO’s highest foaming ability is around pI 4.5 (Zhao et al., 2024).
Furthermore, due to the low denaturation temperature and the low critical gelling concentration compared to proteins of animal origin (proteins from egg white and whey) and vegetable proteins, such as soy and pea protein, RuBisCO gels quickly and at low concentrations. Such gel has a unique brittle and delicate structure that dissolves and has a larger surface area of fragments that bind to taste receptors, resulting in better taste perception. In addition to the good functional properties, the nutritional value and digestibility of the proteins are very good and have no significant allergenic potential and can have great potential to be applied in the food industry (Grácio et al., 2023).
Due to positive functional and nutritional properties and to optimise the yield of the RuBisCO, research is increasingly being conducted into new extraction methods. In general, the classic methods of protein extraction include phenol extraction and isoelectric precipitation extraction. Even though these extraction methods represent the gold standard in protein extraction, nonthermal, environmentally friendly extraction methods are also increasingly being researched (Dukić et al., 2023). Some of the RuBisCO extraction methods already investigated include EAE, UAE, and HVED-assisted extraction (Akyüz & Ersus 2021; Dukić et al., 2023). The yield of these methods is lower than the yield of classical methods, but the fact that these methods are more environmentally friendly and therefore more sustainable should be taken into consideration. Although the development of these methods for the extraction of RuBisCO is still in its infancy, their rapid development in the coming years is undisputed.
Extraction methods of proteins
Conventional extraction methods may reduce extraction yields due to protein degradation. The yield of extraction depends on pH, extraction time, solvents, and temperature (Kumar et al., 2021b). Scientists are investigating how to improve extraction efficiency and reduce protein degradation using nonthermal green extraction techniques (US, microwave, enzyme-assisted, PEF, etc.) to replace or complement conventional extraction methods. Nonthermal green extraction techniques have no harmful impact on the environment. Proteins extracted with those techniques are safe for humans (Chandran et al., 2023). Other eco-innovative technologies such as electrostatic separation, subcritical water extraction, and reverse micelles extraction are also recognised as environmentally friendly (Pojić et al., 2018).
Ultrasound-assisted extraction is not so novel technology in food science. Ultrasound-assisted extraction is based on the principle of acoustic cavitation due to the propagation of mechanical waves formed by a set of cycles of high and low pressures called compressions and rarefactions. It can be used as pretreatment or in combination with conventional and other nonthermal green extraction techniques (Kumar et al., 2021a). Ultrasound waves in the range of 10–1,000 W/cm2 are applied to improve extraction efficiency (Sengar et al., 2022). In comparison to conventional extractions, UAE is increasing extraction yield by approximately 20%. Some studies showed the influence of UAE on isolated proteins. Ultrasound cavitation is impacting hydrophobic interactions and hydrogen bonds in protein structure, so techno-functional characteristics are improved. Ultrasound-assisted extraction reduces processing times. Protein denaturation is less due to low operating temperature (Aghababaei et al., 2024).
Disadvantages of UAE are high energy consumption, protein denaturation, and functional effect change if high power is applied, and extraction time is prolonged. Too-long use of the probe can cause erosion of the metal tip; therefore, dissolved metals can be present in the protein solution (Hadidi et al., 2023). Fatima et al. (2023) studied protein extraction from Moringa oleifera seeds. A protein yield of 39.12% was obtained in UAE at the optimised conditions (75% amplitude, pH 11, 1:20 solute-to-solvent ratio). At optimised conditions, a sonication time of 20 min resulted in a 150% higher yield compared to conventional extraction (Fatima et al., 2023).
Enzyme-assisted extraction (EAE) is based on disrupting cell wall integrity by the enzymatic breaking of components of the cellular wall (cellulose, hemicellulose, and pectin). Breakdown of the cellular wall improves the extraction yield of proteins. One of the most used enzymes is protease. The advantages of EAE are lower energy consumption, reduced solvent use, nontoxic treatment, and increased protein content and extraction yield by 2.2%–12% compared to conventional alkaline extraction. Disadvantages of EAE are expensive enzymes, inactivation of enzymes, different enzyme activities between batches, and product transformation as a result of enzyme activity (Hadidi et al., 2023).
Microwave-assisted extraction (MAE) is a technology based on using microwave energy to heat solvents in contact with a sample to partition analytes from the sample matrix into the solvent. The ability to rapidly heat the sample solvent mixture is inherent to MAE and the main advantage of this technique. Microwaves are electromagnetic radiation with frequencies in the range of 300 MHz–300 GHz (Kumar et al., 2021b). Scientists recommend using MAE for the extraction of proteins from samples with hard structures that are difficult to extract with UAE and EAE.
Pulsed electric field–assisted extraction can efficiently optimise the extraction of proteins within a shorter time while minimising solvent and energy consumption. The basic principle of the PEF technology is the application of short pulses of high electric fields with a duration of microseconds to milliseconds and intensity in the order of 0.1–80 kV/cm. The processing time is calculated by multiplying the number of pulse times with effective pulse duration. The advantages of this technology are enhancing extraction yield, less protein denaturation, and a very short time of PEF treatment. The main disadvantages of PEF are the high cost of equipment and the mild approach leading to the formation of small pores on the cell membranes, thus limiting the release of high-molecular-weight intracellular compounds (Bou et al., 2022; Taha et al., 2022).
Nonthermal extraction methods
Traditional extraction methods often involve harsh conditions like high temperatures and chemical solvents, leading to uncontrolled protein denaturation and reduced yields. Consequently, several sustainable extraction techniques have emerged in recent years to address these limitations.
Although it has been known for many years, the USA has been increasingly used as an extraction method in the food industry since the beginning of the 21st century. As a clean, environmentally friendly technology and an excellent alternative to conventional extraction methods, this nonthermal technique has been used for the extraction of various bioactive compounds from a range of natural matrices, such as plant, animal, and marine sources (Kerboua et al., 2022). Compared to classical thermal extraction, the use of UAE shortens the extraction time, consumes less solvent, is more energy efficient and increases the yield of the extracts and makes their quality better, i.e., their biological activity and functionality are preserved. Extraction with ultrasonic waves, mainly in the frequency range of 20–100 kHz, is based on the phenomenon of cavitation, which is caused by the propagation of sound waves in a liquid medium. The pressure fluctuations in the liquid caused by the movement of the sound waves create bubbles that expand and contract. When the bubbles expand, gases from the surrounding liquid diffuse into the cavities, and, when they contract, they leave them. If the pressure oscillation is large enough, the bubbles grow until they implode. This collapse of the cavitation bubbles leads to local temperature and pressure extremes that affect the neighbouring molecules and destroy matrices. The basic principle of UAE of plant tissues is that the plant material is exposed to high-intensity US waves, which generate acoustic cavitation and consequently cause cell disruption and the release of intracellular contents into the extraction solvent (Chemat et al., 2020). Key UAE parameters required to optimise the extraction of proteins from plant tissues include the extraction solvent, treatment time and temperature, ultrasonic power and frequency, and the type of ultrasonic equipment used (Kumar et al., 2021a). When selecting the optimum extraction conditions, it should be considered that the physicochemical properties and bioactivity of proteins and peptides are determined by their structure, which can be disrupted by ultrasonic treatment. At this point, it should be noted that high-power US generates a local temperature increase that can cause the proteins to unfold into an irreversible denatured state (Su & Cavaco-Paulo 2021). In addition, due to the extreme conditions during UAE, highly reactive radicals can be formed that can cause oxidation of the primary aromatic side chains of the amino acids, which can lead to a change in the secondary and tertiary structure of the proteins.
Moreno-Najera et al. found that the optimal extraction of proteins from jackfruit leaves derived from fruit cultivation was achieved by using 1 M NaCl as extraction solvent with the addition of 1% sodium acetate to obtain 94.9 ± 1.2 mg/gd.m. protein (Moreno-Nájera et al., 2020). For comparison, the protein content obtained by conventional isoelectric precipitation of jackfruit leaves was 84.1 mg/gd.m. The best duration of treatment for sonication at 25 °C and 42 kHz was reported to be 20 min. Inspired by the high content of various nutrients in cauliflower by-products, Xu et al. investigated the optimal parameters for the UAE of proteins from cauliflower leaves (Xu et al., 2017). The highest yield of soluble leaf protein (12.1 mg/g) was obtained with the following optimised extraction conditions: 15 min of US treatment at a power of 175 W, a liquid-to-solid ratio of 4 ml/g, and a pH of 11, adjusted with NaOH. A longer extraction time (30 min) and a decrease in ultrasonic power (50 W) and pH resulted in a decrease in extraction yield. When investigating the functional properties of leaf protein concentrates obtained by UAE and thermal extraction (80–90 °C) of Diplazium esculentum leaves, Saha et al. reported a more than threefold higher total protein content (34.28 compared to 9.89%) in protein concentrates obtained by sonication (t = 29 min, T = 56 °C and pH = 8.7) (Saha et al., 2016). By varying different parameters such as power densities (150, 300, and 450 W/L), temperatures (30, 40, and 50 °C), and time (2.5–20 min), Zhao et al. found that the optimal conditions for UAE of mulberry leaf proteins were 50 °C, 450 W/L, and 20 min, resulting in 30.03% of the total protein being extracted (Zhao et al., 2023). Our group dedicated one of its recent research projects to the nonthermal, green US and HVED-plasma-assisted extraction of proteins, in particular the RuBisCO enzyme, from waste sugar beet leaves (Dukić et al., 2023). Under all conditions tested, including variations in temperature, treatment time, and amplitude of the US treatment, as well as voltage, treatment time, and different gases used (to obtain plasma) in the HVED treatment, US was found to be more effective in extracting total soluble proteins using water as the extraction solvent. The highest total protein content was found in the samples treated with US at 100% amplitude for 9 min, 84.60 ± 3.98 mg/gd.m. at 4 °C and 96.75 ± 4.30 mg/gd.m. at 25 °C. As with total proteins, UAE also proved superior to HVED for RuBisCO extraction, with the highest yield observed for samples treated for 9 min at 75% amplitude. To obtain protein isolates from alfalfa leaves, which consist mainly of albumin, Hadidi et al., (2020) developed a US-ultrafiltration-assisted alkaline isoelectric precipitation, which involves sonication of the samples in an ultrasonic bath at a frequency of 20 kHz and a power of 100 W. Using 1 M NaOH as extraction solvent, the highest protein content (91.1 g/100 g) was obtained under the following conditions: pH of 10.1, T = 42.5 °C, extraction time of 102 min, 43.3 ml/g solvent/solid ratio, and flow rate of 9.7 L/h. In addition to extraction time and solvent/solid ratio, pH and temperature are emphasised as factors that significantly affect extraction yield and protein content. An increase in pH from 9 to 10 led to a significant increase in extraction yield. A further increase in pH (from 10 to 10.5) did not affect the yield, which started to decrease after pH = 10.5. With an increase in extraction temperature from 20 to 45 °C, an increase in protein content was also observed.
Although UAE is an ecologically innovative process that has demonstrated its effectiveness in numerous examples of protein extractions in the laboratory, further research is needed to optimise the parameters required for industrial protein extraction, such as time, temperature, equipment, frequency/energy intensity, and sample/solvent ratio. Advanced US techniques that could enable effective protein extraction on an industrial scale in the future are manothermosonication and Barbell Horn ultrasonic technology, but these still require further optimisation for industrial application (Ampofo & Ngadi, 2022).
Pulsed electric field–assisted extraction has garnered considerable attention as a gentle yet targeted processing method. It can intensify the extraction of valuable intracellular compounds, including proteins, from microorganisms, microalgae, and plant tissues, while also inducing targeted structural modifications of proteins (Carpentieri et al., 2022; Taha et al., 2022). This capability could facilitate the production of various formulated foods with desired functional properties (Taha et al., 2022).
The PEF process involves the application of repetitive (Hz–kHz) electric field (0.5–40 kV/cm) pulses of very short (μs–ms) duration and relatively low energy input (WT = 0.1–150 kJ/kg) to a biomaterial of plant or animal origin, or suspension of microorganisms (e.g., microbes or microalgae) placed in direct contact with the electrodes of a treatment chamber, operated either in batch-wise or in continuous (Gómez-López et al., 2022). The pulses commonly used in PEF treatments are unipolar or bipolar, with either exponential or square-wave shapes (Misra et al., 2017). The main effect of the PEF application is the permeabilisation of cell membranes by pores formation, referred to as electroporation or electropermeabilisation (López-Gámez et al., 2020). The extent of electroporation of membranes of biological cells and PEF treatment efficiency mainly depends on electric field strength (in kV/cm) and total specific energy input (in kJ/kg) (or treatment time, in μs), which are the main process parameters that define the PEF treatment intensity. In general, increasing the intensity of these parameters enhances electroporation (Gómez-López et al., 2022).
This makes PEF technology suitable for enhancing the selective retrieval of target intracellular compounds from biomaterials. In particular, several authors have investigated PEF’s potential to enhance protein extraction yields from various plant sources, including soybeans, peas, rice bran, nettle leaves, white button mushroom, brewer’s spent grain, papaya peels, tomato processing waste, sesame cake, rapeseeds stems, wheat, and algae, while reducing energy costs, solvent consumption, and treatment time (Kashyap et al., 2023; Sarswat et al., 2024). Additionally, PEF offers the advantage of reducing energy costs, solvent consumption, and treatment time.
For example, it was found that as compared to the conventional alkaline extraction, a PEF treatment at 2.3 kV/cm for 25 min (250 pulses/min) significantly increased the extraction yield of proteins from rice bran by 21.8% (Thongkong et al., 2023). Similarly, PEF pretreatment at various field strengths (1–5 kV/cm) and energy inputs (1–28 kJ/kg) led to a 21%–63% increase in protein extraction from tomato processing waste compared to untreated samples. Additionally, the release of intracellular protein from tomato waste tissues positively correlated with both the electric field magnitude and energy input (Andreou et al., 2020). In another work, it has been also observed that the application of PEF as a pretreatment of sesame cake for the extraction of proteins resulted in the reduced amount of organic solvents, time, and temperature needed for the extraction, as compared with untreated samples. Interestingly, proteins were extracted at 40 °C in the first 20 min of the process, indicating the relevance of this technique for industrial use (Sarkis et al., 2015). A higher soluble protein yield and shortened processing time were also observed when PEF pretreatment was applied to nettle leaves (Urtica dioica L.) before solid–liquid extraction (Kronbauer et al., 2023). Furthermore, the effectiveness of PEF processing to extract proteins from plant tissues also depends on the complex interaction between electrical parameters and extrinsic factors, such as temperature and pH.
It is known that the combination of PEF with moderate processing temperature can increase the sensitivity of cell membranes to electric pulses, thereby significantly enhancing treatment effectiveness (Gómez-López et al., 2022). To this regard, a study found that subjecting a suspension of white button mushrooms (9% wt/wt) to PEF treatment at a field intensity of 38.4 kV/cm for 272 μs, at a treatment temperature of 85 °C resulted in a protein extraction yield of 49% ± 0.09 (2.7 mg/g of mushroom) proteins. In contrast, the protein yield from conventional extraction at the same temperature and for a few minutes was negligible, suggesting a synergistic effect of electric field and temperature on extraction performance (Xue & Farid, 2015). Similarly, the increase in PEF intensity and temperature has been found to cause higher protein yields from nettle leaves, suggesting a potential synergistic interaction between these variables (Kronbauer et al., 2023). However, it is worth mentioning that an increase in temperature during PEF treatment could facilitate the denaturation of protein molecules (Taha et al., 2022). Thus, further study of the effects of PEF on protein structures under controlled temperatures is recommended.
On the other hand, pH is a critical parameter in protein extraction processes, influencing the efficiency and quality of protein recovery from plant-based materials, such as solubility, stability, enzymatic activity, and selectivity. Optimising the pH of the extraction medium based on the properties of target proteins and the extraction method employed is essential for maximising protein yield and quality. Nevertheless, few studies have explored the combined impact of PEF and pH on protein extraction from plant material. In this context, it has been revealed that both PEF and pH play pivotal roles in fractionating red seaweed (Gracilaria sp.) biomass, enhancing protein release compared to water-mediated extraction, PEF pretreatment, or pH adjustment alone. Specifically, the combination of PEF and sequential pH adjustments (pH 1–12) yielded a protein release of 28.02 ± 0.2%, an essential amino acid content of 63.6 ± 5.3%, and a branched-chain amino acid content of 3.47 ± 0.06%. This underscores the cooperative effect of combining PEF and pH-shift treatments, especially when gradually increasing pH from 1 to 12, highlighting their synergistic impact in augmenting protein release yield, particularly essential amino acids and branched amino acids, in Gracilaria sp. biomass extracts (Kashyap et al., 2023).
A more intricate interaction has been observed when examining the simultaneous effects of PEF, temperature (20–60 °C), and pH (7–11) on protein extraction yields from papaya peels. Specifically, increasing pH led to a rise in protein concentration. However, protein concentrations at pH = 7 and pH = 11 were notably lower at 60 °C compared to those measured at 50 °C. Remarkably, combining PEF treatment with subsequent aqueous extraction at 50 °C resulted in enhanced protein yields from papaya peels, even under neutral pH conditions (Parniakov et al., 2015). Despite the potential of PEF technology to enhance protein extractability from various plant sources, there have been few studies exploring the effects of PEF, alone or in a hurdle approach, on the structure and techno-functional properties of plant-based proteins. There is limited evidence indicating that PEF treatment can induce changes in the primary structure, while the effects on the secondary structure are relatively clear and to a lesser extent on the tertiary and quaternary structures (Fan et al., 2022).
This suggests that structural alterations induced by PEF are likely responsible for the functional changes observed in proteins. However, the extent of these changes depends on the PEF treatment intensity and type of proteins (Taha et al., 2022).
The secondary structure of soy protein isolate changed after PEF treatment at 30–50 kV/cm. Additionally, PEF caused denaturation and aggregation of soy protein isolate, probably due to the formation of hydrophobic interactions and S–S bonds (Liu et al., 2011). Similarly, PEF treatment changed the secondary structure of canola protein in the proportions of α-helices and β-sheets. Furthermore, an increased amount of free sulphydryl groups and surface hydrophobicity and the formation of new protein aggregates suggested alterations in tertiary and quaternary structure as well. As a result, it was found that PEF pretreatment significantly improved the functional properties of canola protein, such as solubility, water-holding capacity, emulsibility, emulsion stability, oil-holding capacity, foamability, and foam stability (Zhang et al., 2017). Similar results were also reported for rice bran proteins (Thongkong et al., 2023).
The main mechanism behind the effects of electric fields on proteins is not very clear. However, based on the few published studies, it has been hypothesised that during PEF treatment, polar groups within proteins absorb energy and generate free radicals. These radicals can disrupt various intramolecular interactions within protein molecules, such as hydrophobic and electrostatic interactions, disulphide bridges, hydrogen bonds, salt bridges, and van der Waals forces. Additionally, PEF treatments may alter the apparent charge of proteins by affecting their ionic interactions. Consequently, these changes can lead to alterations in the structural and functional properties of proteins (Taha et al., 2022). However, further research, including the use of molecular dynamics simulation tools (Marracino et al., 2019), is necessary concerning molecular perception to elucidate the alterations in protein levels within the plant matrix under various PEF processing conditions and environmental factors.
Green-solvent selection for extraction of proteins
Solvent extraction is a widely used technique for separating and purifying natural products from complex matrices. Choosing the right solvent is crucial to the success of this process. Traditionally, solvent selection has been based on experience and intuition, which can be tedious and time-consuming. Recently, computational approaches have been developed to predict the extraction efficiency of different solvents. These methods make it possible to explore a wide range of solvents and predict their performance before costly and time-consuming experimental testing. One of the most popular models for predicting solvent extraction is COSMO-RS (Conductor-like Screening Model for Real Solvents). This model is based on continuous solvation theory and considers electrostatic interactions between solute and solvent. The COSMO-RS model uses a statistical thermodynamic approach based on the results of quantum chemical calculations to understand the dissolution mechanism. COSMO-RS combines considerations from quantum chemistry (COSMO) and statistical thermodynamics (RS) to determine and predict the thermodynamic properties without experimental data. First published by Klamt (Klamt & Schüürmann 1993), the conductor-like screening model, generally referred to as COSMO, has become very popular in computational chemistry as it is an efficient variant of apparent surface charge dielectric continuum solvation models (Klamt, 2011).
COSMO-RS is used to predict a wide range of thermodynamic properties, such as the solubility of gases in liquids, activity coefficients of solutes in mixtures, enthalpies of mixing, phase equilibria, and phase diagrams. The model has also been used to develop calculation methods for solvent design, chemical process simulation, and reactor performance optimisation.
COSMO-RS is a two-step procedure comprising microscopic and macroscopic steps (Figure 1). In the first step, the solute is treated as if it were embedded in a dielectric medium via a molecular surface built around the molecule. This is a macroscopic approach using the dielectric constant of the solvent. COSMO-RS, with the extension RS for “real solvent,” is a combination of an electrostatic theory, COSMO, with the statistical thermodynamic treatment of interacting surfaces (Klamt, 2011). It describes liquid-phase interactions such as molecular surface interaction contacts. This tool generates a charge density surface that reflects the most stable state of the molecule in a perfect conductor. The solute molecule is immersed in a continuous medium of dielectric constant ε, which can be represented by a virtual perfect conductor (Dupeux et al., 2022).
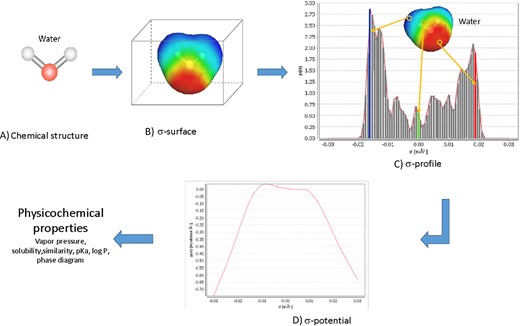
Different calculation steps of Conductor-like Screening Model for Real Solvents.
In this environment, a surface is built around the molecule that generates many electrostatic charges. The structure and distribution of charges are optimised to find the minimum energy of the system (molecule in its most stable state), thanks to calculations based on algorithms, called density functional theory. This charge density is called σ-surface. The colour coding of this σ-surface provides information on the charge density at each point of the molecule. The green zone corresponds to zero charge density, blue characterises positive charge density δ+, and red signifies negative charge densities δ−. In other words, green zones are generally linked to the carbon skeleton, red zones to oxygen or nitrogen groups, and blue zones to hydrogen atoms. COSMO-RS is a powerful tool for predicting the solvent extraction of natural products. The model helps accelerate and rationalise the development of new solvents and optimise existing extraction processes.
Analytics for the characterisation of RuBisCO and peptides
In terms of carboxylase activity, Rubisco enables the addition of inorganic carbon (CO2) and water to an enediol of ribulose-1,5-bisphosphate (RuBP), an organic intermediate of the Calvin–Benson–Bassham pathway (Antonovsky et al., 2017). In this preferred reaction, two molecules of 3-phospho-D-glycerate-a are used to rebuild RuBP generating 0.5 O2. The carboxylase efficiency of the RuBisCO is arbitrated by its oxygenase activity and affected by environmental conditions (Amaral et al., 2024). Besides the 3-phospho-D-glycerate, the oxygenase reaction of the RuBisCO produces a 2-phosphoglycolate, nowadays a less desirable compound recycled via the photorespiratory pathway, and CO2 (and NH4) (Bauwe, 2023).
A fast search in the protein database of the National Center for Biotechnology Information (NCBI; https://www.ncbi.nlm.nih.gov/) results in more than 380,000 items counting the L and the S subunits of the RuBisCO, mainly detected in chloroplasts. There is also a comfortable number of three-dimensional (3D) structures of the RuBisCO including mutants (>200) in the PDB (https://www.rcsb.org/). Large amounts of the RuBisCO are available in algae, cyanobacteria, and leaves of C3 and C4 plants, and, currently, this nonallergenic protein with a high content of essential amino acids and bioactive peptides represents a highly valuable protein source (Dukić et al., 2023; Grácio et al., 2023; Karasawa et al., 2023). It appears that sustainable, efficient, and cost-effective isolation and purification of the RuBisCO on a large scale, predominantly by fractionation methods, suitable for its use in food and feed has not yet been achieved.
The isolation of the RuBisCO slightly evolved from the well-known procedure described by Spreitzer and Mets (1980), and in general, includes (i) preparation of a cell-free extract of (micro)organisms or an extract of pulverised (frozen) leaves (solid–liquid extraction); then, (ii) selective precipitation of soluble proteins from the extract, a partition of resulting pellets (by centrifugation), and solubilisation of the pellets in a suitable aqueous buffer; after that, (iii) separation of the RuBisCO by liquid chromatography; and (iv) a concentration and further characterisation of purified RuBisCO (Matsushima & Matsuo 2024; Wilson et al., 2019).
Bioinformatic—tools, protein and peptide databases
RuBisCO protein is found in various organisms. The UniProt database contains data on RuBisCO protein from beet under the name “Ribulose bisphosphate carboxylase large chain,” gene name “rbcL,” the taxonomic designation “Beta vulgaris (species)” taxonomic number ID 161934. RuBisCO protein from the broad bean plant (lat. Vicia faba) is found in the UniProt database under the name “Ribulose bisphosphate carboxylase large chain,” gene name “rbcL,” taxonomic designation “V. faba (broad bean) (Faba vulgaris),” under the taxonomic ID number 3906.
For mass spectrometry (MS) analysis of isolated RuBisCO proteins from plant leaves, it is necessary to find the protein sequences of the same or most similar proteins from existing databases. For MS/MS identification of proteins during the analysis, the mass spectrometer will first identify the masses of whole peptides (most often cleaved by trypsin, which cleaves after the amino acids arginine and lysine if it is not proline before) of the protein in the MS1 analysis and thus obtain the peptide masses. Then, these peptides enter the MS2 analysis, where the masses of the ion fragments formed by further cleavage of the peptide are obtained. Computer algorithms will compare such mass spectra with the theoretical spectrum of each peptide cleaved in silico by trypsin.
Databases and data analysis
Introduction to bioinformatics databases
There are two main types of bioinformatics databases: primary and secondary (Christensen, 2023; Selvakumar et al., 2024; Swan et al., 2024).
Primary databases are populated with experimentally derived data such as nucleotide sequence, protein sequence, or macromolecular structure. These databases are essentially archival in nature, forming part of the scientific record (Christensen & de Vries, 2023). Examples of primary databases include GenBank (https://www.ncbi.nlm.nih.gov/genbank/) from the NCBI, the European Molecular Biology Laboratory database (https://www.ebi.ac.uk/), and the PDB (https://www.rcsb.org/) (Zardecki et al., 2022).
Secondary databases, on the other hand, comprise data derived from the results of analysing primary data. They often draw upon information from numerous sources, including other databases (primary and secondary), controlled vocabularies, and the scientific literature (Christensen & de Vries, 2023). They are highly curated, often using a complex combination of computational algorithms and manual analysis and interpretation to derive new knowledge from the public record of science. Examples of secondary databases include InterPro (protein families, motifs, and domains, https://www.ebi.ac.uk/interpro/), UniProt Knowledgebase (sequence and functional information on proteins, https://www.uniprot.org/help/uniprotkb), and Ensembl (variation, function, regulation, and more layered onto whole genome sequences, https://www.ensembl.org/index.html) (Christensen & de Vries, 2023).
UniProt (Universal Protein Resource) is a comprehensive, expertly managed, publicly available database of protein sequences, functional information, and variation. It was created as a collaboration between the European Institute for Bioinformatics (EMBL-EBI), the Swiss Institute for Bioinformatics, and the Protein Information Resource. The number of UniProt sequences is over 227 million. The UniProt database (UniProtKB) is divided into two main parts; it consists of a screened set of proteins (UniProtKB/Swiss-Prot), where each protein entry is associated with a summary of experimentally verified or computationally predicted functional information added by our expert biocuration team, and unaudited (UniProtKB/TrEMBL), in which the entries are computer marked by automated systems.
The nonredundant NCBInr database maintained by NCBI contains collected records from several databases, such as the translated coding regions of the GenBank database, as well as the Swiss-Prot database, PDB, Protein Information Resource, and NCBI RefSeq. The NCBInr database is useful for MS/MS analysis because it contains the largest number of nonrepeating (nonredundant) protein sequences, which means that proteins with the same protein sequence have only one record.
Both UniProt and NCBInr databases contain the same taxonomic data related to protein sequences. The main difference between these two databases lies in how the sequences are annotated and maintained. UniProtKB/Swiss-Prot is manually annotated and maintained, while NCBInr is automatically generated or takes data from other databases. NCBInr takes care of protein sequence redundancy. Therefore, NCBInr contains more data, which can be useful for de novo MS analysis of proteins.
Protein and peptide databases
Protein and peptide databases are essential resources for bioinformatics databases, providing comprehensive repositories of sequence and structural data. They also play a crucial role in various areas of research, including protein function prediction, drug discovery, and proteomics (Zardecki et al., 2022). Table 1 presents the main protein and peptide databases.
Database type . | Name . | Description . | Main features . | Reference . |
---|---|---|---|---|
Proteins | Protein Data Bank (PDB) | Single global repository for the three-dimensional structural data of large biological molecules, including proteins and nucleic acids, providing a broad range of tools and resources for the study of the structures of biological macromolecules and their relationships to sequence, function, and disease. | The structures are determined experimentally using techniques such as X-ray crystallography, nuclear magnetic resonance (NMR) spectroscopy, and cryo-electron microscopy | (Berman et al., 2000) (Zardecki et al., 2022) |
Universal Protein Resource (UniProt) | Comprehensive resource for protein sequence and annotation data, consisting of a central repository of protein data that provides the scientific community with a unified view of protein information. | Updated and maintained by the UniProt Consortium, which consists of several leading academic institutions, it contains manually annotated records extracted from literature and curated computational analysis | (Bateman et al., 2023) (Coudert et al., 2023) | |
Peptides | PeptideAtlas | Multi-organism, a publicly accessible compendium of peptides, providing a map of the proteomic observables for each species included, which can be used for the selection of peptide targets suitable for targeted proteomics experiments | Peptides are identified in a large set of tandem mass spectrometry proteomics experiments | (Desiere et al., 2006; Farrah et al., 2011) |
PRoteomics IDEntifications (PRIDE) | The world’s largest data repository of mass spectrometry–based proteomics data. PRIDE is one of the founding members of the global ProteomeXchange (PX) consortium and an ELIXIR core data resource | The database accepts data generated by the proteomics community, making the data freely available to the scientific community | (Deutsch et al., 2023; Perez-Riverol et al., 2022) |
Database type . | Name . | Description . | Main features . | Reference . |
---|---|---|---|---|
Proteins | Protein Data Bank (PDB) | Single global repository for the three-dimensional structural data of large biological molecules, including proteins and nucleic acids, providing a broad range of tools and resources for the study of the structures of biological macromolecules and their relationships to sequence, function, and disease. | The structures are determined experimentally using techniques such as X-ray crystallography, nuclear magnetic resonance (NMR) spectroscopy, and cryo-electron microscopy | (Berman et al., 2000) (Zardecki et al., 2022) |
Universal Protein Resource (UniProt) | Comprehensive resource for protein sequence and annotation data, consisting of a central repository of protein data that provides the scientific community with a unified view of protein information. | Updated and maintained by the UniProt Consortium, which consists of several leading academic institutions, it contains manually annotated records extracted from literature and curated computational analysis | (Bateman et al., 2023) (Coudert et al., 2023) | |
Peptides | PeptideAtlas | Multi-organism, a publicly accessible compendium of peptides, providing a map of the proteomic observables for each species included, which can be used for the selection of peptide targets suitable for targeted proteomics experiments | Peptides are identified in a large set of tandem mass spectrometry proteomics experiments | (Desiere et al., 2006; Farrah et al., 2011) |
PRoteomics IDEntifications (PRIDE) | The world’s largest data repository of mass spectrometry–based proteomics data. PRIDE is one of the founding members of the global ProteomeXchange (PX) consortium and an ELIXIR core data resource | The database accepts data generated by the proteomics community, making the data freely available to the scientific community | (Deutsch et al., 2023; Perez-Riverol et al., 2022) |
Database type . | Name . | Description . | Main features . | Reference . |
---|---|---|---|---|
Proteins | Protein Data Bank (PDB) | Single global repository for the three-dimensional structural data of large biological molecules, including proteins and nucleic acids, providing a broad range of tools and resources for the study of the structures of biological macromolecules and their relationships to sequence, function, and disease. | The structures are determined experimentally using techniques such as X-ray crystallography, nuclear magnetic resonance (NMR) spectroscopy, and cryo-electron microscopy | (Berman et al., 2000) (Zardecki et al., 2022) |
Universal Protein Resource (UniProt) | Comprehensive resource for protein sequence and annotation data, consisting of a central repository of protein data that provides the scientific community with a unified view of protein information. | Updated and maintained by the UniProt Consortium, which consists of several leading academic institutions, it contains manually annotated records extracted from literature and curated computational analysis | (Bateman et al., 2023) (Coudert et al., 2023) | |
Peptides | PeptideAtlas | Multi-organism, a publicly accessible compendium of peptides, providing a map of the proteomic observables for each species included, which can be used for the selection of peptide targets suitable for targeted proteomics experiments | Peptides are identified in a large set of tandem mass spectrometry proteomics experiments | (Desiere et al., 2006; Farrah et al., 2011) |
PRoteomics IDEntifications (PRIDE) | The world’s largest data repository of mass spectrometry–based proteomics data. PRIDE is one of the founding members of the global ProteomeXchange (PX) consortium and an ELIXIR core data resource | The database accepts data generated by the proteomics community, making the data freely available to the scientific community | (Deutsch et al., 2023; Perez-Riverol et al., 2022) |
Database type . | Name . | Description . | Main features . | Reference . |
---|---|---|---|---|
Proteins | Protein Data Bank (PDB) | Single global repository for the three-dimensional structural data of large biological molecules, including proteins and nucleic acids, providing a broad range of tools and resources for the study of the structures of biological macromolecules and their relationships to sequence, function, and disease. | The structures are determined experimentally using techniques such as X-ray crystallography, nuclear magnetic resonance (NMR) spectroscopy, and cryo-electron microscopy | (Berman et al., 2000) (Zardecki et al., 2022) |
Universal Protein Resource (UniProt) | Comprehensive resource for protein sequence and annotation data, consisting of a central repository of protein data that provides the scientific community with a unified view of protein information. | Updated and maintained by the UniProt Consortium, which consists of several leading academic institutions, it contains manually annotated records extracted from literature and curated computational analysis | (Bateman et al., 2023) (Coudert et al., 2023) | |
Peptides | PeptideAtlas | Multi-organism, a publicly accessible compendium of peptides, providing a map of the proteomic observables for each species included, which can be used for the selection of peptide targets suitable for targeted proteomics experiments | Peptides are identified in a large set of tandem mass spectrometry proteomics experiments | (Desiere et al., 2006; Farrah et al., 2011) |
PRoteomics IDEntifications (PRIDE) | The world’s largest data repository of mass spectrometry–based proteomics data. PRIDE is one of the founding members of the global ProteomeXchange (PX) consortium and an ELIXIR core data resource | The database accepts data generated by the proteomics community, making the data freely available to the scientific community | (Deutsch et al., 2023; Perez-Riverol et al., 2022) |
Data analysis and tools
Data mining in bioinformatics involves the application of techniques from machine learning and statistics to extract knowledge from biological databases. It is a crucial component of bioinformatics research, enabling researchers to make sense of the vast amounts of biological data generated by genomic and proteomic experiments. Data mining techniques can be used for a variety of tasks in bioinformatics, including protein structure prediction, gene classification, cancer classification based on microarray data, clustering of gene expression data, and statistical modelling of protein–protein interaction (Mishra et al., 2024).
Some of the tools that can be applied in data analysis include the Basic Local Alignment Search Tool (BLAST, https://blast.ncbi.nlm.nih.gov/Blast.cgi) and Clustal Omega (https://www.ebi.ac.uk/jdispatcher/msa/clustalo). The BLAST is a fundamental tool in bioinformatics, used to find regions of similarity between biological sequences. It compares nucleotide or protein sequences to sequence databases and calculates the statistical significance of matches. The BLAST can be used to infer functional and evolutionary relationships between sequences, as well as help identify members of gene families (Camacho et al., 2023). Clustal Omega is a multiple sequence alignment program that uses seeded guide trees and hidden Markov model profile–profile techniques to generate alignments between three or more sequences. It is particularly useful for aligning large numbers of sequences, making it a valuable tool for bioinformatics research (Sudhasini & Ashadevi 2023).
Data mining techniques and bioinformatics tools like BLAST and Clustal Omega are essential for analysing the vast amounts of data in bioinformatics. They enable researchers to extract meaningful information from biological data and contribute significantly to advancements in the field (Mishra et al., 2024).
Sustainability
Three pillars of sustainability (economy, environment, and society)
Sustainable development is an integrated approach recognising the connection between economic, social, and environmental systems. Economically, it promotes inclusive growth, innovation, and resilience. Socially, it emphasises equity, justice, and access to essential services. Environmentally, it focuses on preserving resources, reducing pollution, and combating climate change for future generations. Achieving sustainable development requires collaboration among all sectors, responsible consumption, and innovative solutions.
The concept, rooted in ethical principles, centres on intra- and intergenerational justice (fairness within and between generations). Sustainable development is key to the United Nation’s Agenda 2030, aiming to meet current needs without compromising future opportunities. It challenges traditional development models, integrating ethics into economic progress and advocating for balanced, multidimensional growth that considers social and ecological impacts. This approach seeks to reconcile conflicting developmental goals, fostering coherence between economic, social, and environmental objectives (Hassoun et al., 2022b; Jambrak et al., 2021; Sabol et al., 2013).
Sustainable development addresses challenges like poverty, inequality, biodiversity loss, and climate change. It requires integrating sustainability into decision-making, promoting global cooperation, and adopting sustainable practices across industries. The key to this is promoting sustainable consumption and production by reducing resource use, minimising waste, and advancing renewable technologies. Sustainable consumption and production aim to decouple economic growth from environmental harm, fostering innovation for a sustainable future. This approach emphasises equity, justice, and responsible business practices, including sustainable supply chains, emissions reduction, and ethical labour. By embracing innovation and responsible practices, societies can achieve sustainable progress and ensure a better future.
One of the key targets of the SDGs is to address food security and sustainability. As the global population continues to expand and industrialisation accelerates, concerns about chronic hunger, nutritional deficiencies, and environmental damage are growing (Kamal et al., 2021).
Nonthermal processing, recognised for its promise, safety, and efficacy in extracting bioactive compounds from food residues, contributes to implementing a circular economy and achieving SDGs (Arshad et al., 2022). The NTP methods such as high-pressure treatment, UAE, PEF, and HVED significantly mitigate environmental footprints, contributing to SDGs related to responsible consumption and production (SDG 12) and climate action (SDG 13). Moreover, by enhancing the efficiency of food preservation processes and minimising resource wastage, nonthermal technologies align with SDGs targeting zero hunger (SDG 2) and clean water and sanitation (SDG 6). Their ability to preserve functional ingredients and antioxidant qualities further bolsters efforts to promote health and well-being (SDG 3) while fostering economic growth (SDG 8). Therefore, through the incorporation of nonthermal technologies into food production systems, stakeholders can promote a comprehensive approach to sustainable development, balancing economic prosperity with environmental management and social equity (Jambrak 2022; Nutrizio et al., 2022; Schottroff et al., 2022).
The imperative to transition towards sustainable methods in protein extraction shows the bigger idea of sustainable development. As industrialisation and population growth place pressure on natural resources and ecosystems, there is a growing demand for innovative and eco-friendly approaches. Eco-innovations, which imply changes in technological processes where agroindustrial residues and by-products of plant and animal origins are transformed into valuable resources (Baiano & Fiore, 2023), appear as a key strategy in the sustainable approach (Hassoun et al., 2023; Katsaros et al., 2022; Wang et al., 2022). By reprocessing and utilising agroindustrial discards and plant-based by-products, rich in antioxidants, dietary fibres, proteins, carbohydrates, and colourants, innovative products can be developed to meet commercial demand within the food chain (Lee et al., 2024). One of the key targets of the SDGs is to address food security and sustainability. As the global population continues to expand and industrialisation accelerates, concerns about chronic hunger, nutritional deficiencies, and environmental damage are growing (Kamal et al., 2021).
The imperative to transition towards sustainable methods in protein extraction shows the bigger idea of sustainable development. As industrialisation and population growth place pressure on natural resources and ecosystems, there is a growing demand for innovative and eco-friendly approaches. Eco-innovations, which imply changes in technological processes where waste and by-products are transformed into valuable resources (Baiano & Fiore 2023), appear as a key strategy in the sustainable approach (Hassoun et al., 2023; Katsaros et al., 2022; Wang et al., 2022). By reprocessing and utilising agroindustrial discards and by-products, rich in antioxidants, dietary fibres, proteins, carbohydrates, and colourants, innovative products can be developed to meet commercial demand within the food chain (Lee et al., 2024).
Environment
As mentioned previously, proteins can be obtained from two main sources—animals and plants, with animal-based protein being in focus due to their potentially higher environmental impact (Chandran et al., 2023). However, speaking about the potential of sustainable nonthermal technologies used for the extraction of proteins, the focus needs to be narrowed only to the extraction process. The energy savings are obtained by decreasing the time the food matrix is exposed to the extraction process, such as in the case of UAE (Chandran et al., 2023) or PEF-assisted extraction where time can be in micro- or milliseconds (Bocker & Silva 2024). Decreased use of chemicals is recorded in MAE and UAE (Chemat et al., 2020). Enzyme-assisted extraction uses water as a preferred solvent elevating its environmental perspective (Kumar et al., 2021b).
To summarise, energy is used for equipment needed for extraction. If needed, energy can be deployed to different types of energy (green, electric, etc.). Water is used: (a) for sample preparation, (b) as a solvent, and (c) for cleaning and sanitising the extraction equipment (Rezek Jambrak et al., 2018). Wastewater because of cleaning and sanitation should be considered since this is part of good hygiene practice (CAC/RCP 1:1969, Rev. 4:2003, Recommended International code of practice—General principles of food hygiene). In some cases, waste can occur and, as such, needs to cascade by type and if needed, treated by applying a waste management hierarchy (Lee et al., 2024). A simplified environmental impact assessment of the nonthermal extraction of proteins is presented in Figure 2.
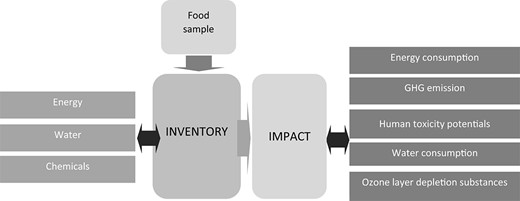
Simplified environmental impact assessment of nonthermal extraction of proteins.
Speaking only about the global warming potential as the main indicator related to climate change, (Berardy et al., 2019) analysed protein values of food with this environmental impact showing that food with the best ratio of the two indicators are peanuts, whey, and soy, while the worst are beef, white rice, and bread. This brings to attention the source of proteins (animals and/or plants) as they can have similar climate change impacts from a nutritional point of view before calculating the impact of extraction. Finally, agroindustrial discards and by-products, although not new, are a promising source for the extraction of proteins as the material (agroindustrial discards and by-products) has a recycling value (Kamal et al., 2021). This can also enhance the achievement of “zero waste” goal, as outlined by Pojić (Pojić et al., 2018).
Sustainable delivery systems: electrospinning, electrospraying, and encapsulation
Electrospinning
In response to changing consumer demands for healthier and more sustainable food choices, the food industry is exploring the use of environmentally friendly materials to develop alternative food structures with potential health advantages. A key focus area involves modifying food microstructures to impart specific functions, enabled by advancements in food science and enhanced comprehension of morphological structures in functional foods (Fasolin et al., 2019; Munialo et al., 2022). Numerous studies underscore the importance of microstructures in influencing sensory attributes and biochemical processes during food digestion. Several microstructures investigated for functional food applications include microcapsules, nanoemulsions, and nanofibrous structures (Gómez-Mascaraque et al., 2018; Mendes et al., 2017). These play a crucial role in the encapsulation, transport, or release of bioactive and aroma compounds. Protein fibrous structure synthesis has garnered significant attention due to the role of resulting electrospun protein scaffolds in accomplishing a whole range of food product textures (and forming essential nutrients). The micro/nanofibrous properties significantly affect food quality features, making it an ideal carrier for bioactive compounds (Dehnad et al., 2024).
While techniques like 3D printing, extrusion, and spinning have been employed to fabricate protein fibrous structures, they often produce fibres that are only a few millimetres or microns in size, which may not faithfully replicate natural microstructures. The exploration of nanoscale protein fibres with precise dimensions and microstructures remains limited due to a shortage of suitable raw materials and a lack of food-grade options (Dehnad et al., 2023; Ghorani & Tucker 2015). Electrospinning emerges as a promising method for producing polymer fibres ranging from submicron to nanoscale sizes, offering precise control over fibre shape, orientation, and dimensions. However, spinnable proteins successfully present challenges due to their complex architectures and strong intermolecular interactions. Adding organic solvents or spinnable polymers to proteins is often needed to make spinning work better, but this can leave behind chemical residues that make purification processes harder (Aghababaei et al., 2024; Chen et al., 2023). Concerns about the greenhouse gas emissions from livestock farming are largely driving the shift in consumer preferences towards balanced diets that include both plant-based and animal-based foods. This trend has fuelled the growth of the plant-based food industry, projected to reach approximately 25 billion tonnes by 2030. While zein, pea, soy, and potato proteins are commonly used in plant-based foods, there is a growing interest in exploring protein alternatives derived from locally sourced agricultural crops (Peydayesh et al., 2022; Sadhukhan et al., 2020).
Electrospinning is commonly regarded as a highly adaptable and effective technique for producing nanofibres due to its straightforwardness and versatility. Nevertheless, many methods of nanofibre manufacturing have constraints, such as being applicable exclusively to noncontinuous nanofibres, generating fibres with specified diameters, and being primarily compatible with certain polymers (Göksen et al., 2020; Nieuwland et al., 2014). The essential elements of the electrospinning process consist of a syringe pump, a high-voltage power source, and a collector. The generation of nanofibres is governed by parameters such as the electrical conductivity and viscosity of the fluid. Electrospinning requires a minimum level of electrical conductivity for nanofibre creation; hence, solutions that are not conductive cannot be used (Dehnad et al., 2023, 2024; Goksen et al., 2022; Jurić et al., 2024). The characteristics of nanofibres are influenced by various parameters, including the kind of polymer and solvent, the concentration of the polymer, and the temperature. The electrical conductivity of solutions containing polymers with ionic functionality is dependent on the concentration. The electrospinning process can be categorised into three main stages: the development of a Taylor cone, the course of a straight jet, and the zone of instability when the jet whips. These stages depend on the Coulombic forces between charges present on the surface of the liquid and the forces exerted by the external electric field (Aghababaei et al., 2024). The creation of the Taylor cone is principally controlled by the electrostatic force resulting from the surface charge caused by the external electric field (Gagaoua et al., 2022; Goksen et al., 2022; Rostami et al., 2023).
Electrospinning has advantages for encapsulating technologies, such as spray drying, especially when it comes to protecting heat-sensitive bioactive components, peptides, and proteins. This is because electrospinning operates at room temperature. In addition, researchers have taken advantage of its extensive surface area to integrate a range of bioactive components, proteins, and biopeptides. The appeal of electrospinning rests in its cost-efficiency, capacity to generate nanofibres, and user-friendly nature. Biopolymer-based nanofibrous mats offer several benefits, including a high surface area-to-volume ratio, a porous structure with linked pores, and the capacity to encapsulate active agents such as bioactive molecules, proteins, and biopeptides (Chen et al., 2023; Rostami et al., 2023). Figure 3 illustrates the electrospinning/electrospray scheme.
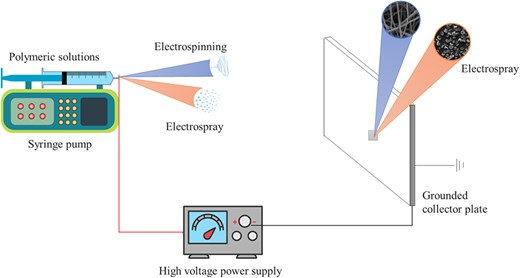
Electrospraying of proteins
Electrospraying, often also referred to as electrohydrodynamic atomisation, is one of the emerging techniques for creating micro and nanoparticles for use in biomedical fields and beyond (Zamani et al., 2014).
Electrospraying involves applying a high voltage (V) to a liquid containing the protein, forcing it to form fine droplets due to the electrostatic forces. The current (I) generated in this process depends on the resistance (R) of the medium, which can vary based on the solvent, protein concentration, and specific electrospray conditions. Ohm’s law, V = R·I, is a fundamental equation used to describe the relationship between the applied voltage (V), the resistance (R), and the resulting current (I) in the system. To determine the intensity per unit mass of protein, one can calculate the current and relate it to the energy expended during the electrospray process.
There are many variables during electrospinning, which affect the energy required for production. Up to now, no study has been conducted in the literature on how much energy is needed for protein electrospun. In addition, the process parameters will vary during the electrospun production of each protein. Therefore, it will vary depending on the type of protein to be studied, its concentration, solution properties, flow rate, voltage, distance between the syringe tip and the collector plate, and environmental conditions (Kang et al., 2020).
Kang et al. (2020) reported the power consumption (P, W) for two systems of electrospinning, for tube-based electrospinning,
and for new spinneret-based electrospinning,
Briefly, a high voltage is applied to a liquid as it passes through a capillary nozzle. By this, the process induces electrical charges within the liquid, which counteract its surface tension, transforming the droplet into a cone-like shape known as a “Taylor cone” (Rosell-Llompart et al., 2018). At a crucial point where, electrostatic forces exceed surface tension, the droplet at the capillary’s tip disintegrates into further droplets. Charged droplets then move towards a grounded collector and while they travel, the solvent evaporates, resulting in the collection of solidified particles (Hartman et al., 2000). The significance of this methodology in augmenting sustainability across various sectors is shown by its pioneering status in domains, such as biodegradable packaging, improved medication delivery systems, and scaffolds for tissue engineering (Jurić et al., 2023; Jurić et al., 2024).
Still, one of the major drawbacks when using proteins as medication delivery systems is immunogenicity. The recent studies underscore the limitations of using protein-based therapeutics as carriers for active drugs, particularly in the treatment of chronic diseases where immunogenicity can severely compromise treatment outcomes (Ebrahimi & Samanta 2023).
Despite many advantages, the use of proteins/peptides as bioactive ingredients in food has disadvantages. They are highly hygroscopic, bitter in taste, with low water solubility and physicochemical instability, and with limited bioaccessibility (Berraquero-García et al., 2023). Solid versions of proteins are being developed to tackle these issues. However, these approaches are not without their limitations, which encompass the possibility of biological activity loss and high energy consumption.
Beyond the pharmaceutical potential, electrospraying extends even deeper, into sustainable food production. This can provide a method to encapsulate proteins that improve food preservation and quality with a reduced environmental footprint. With its ability to precisely control particle size and distribution, electrospraying is an excellent method for the encapsulation of proteins, more efficient than alternatives for processing proteins as deliverable materials, ensuring their stability and functionality (Tanhaei et al., 2021).
Electrospraying produces particles with a narrow size distribution while offering protection against denaturation with precisely developed controlled release and maintained biological activity of proteins, making it suitable for creating solid forms of proteins and enzymes without losing bioactivity. Furthermore, encapsulating proteins into biodegradable carriers has been explored to protect them from degradation, enhance oral delivery, and provide controlled release, with electrospraying being highlighted as a simple and efficient method for creating protein-loaded micro- or nanoparticles (Nguyen et al., 2016).
The utilisation of plant proteins has become increasingly significant in various industries due to a combination of environmental, nutritional, and economic factors. The objectives of plant-protein utilisation span improving human health, enhancing food security, supporting sustainability, and diversifying food products. Many areas in medicine and industry would benefit from the oral delivery of bioactive proteins and peptides. Food-grade colloidal systems can be used in the preparation of functional food (fluid beverage or soft cereal bar), medical food (hard pill), or supplements (soft capsule) (Perry & McClements 2020). Perry and McClements stated that proteins/peptides should be compatible with the product matrix. This makes biopolymers a great candidate for the development of food-grade colloidal systems for the delivery of proteins/peptides. It must be noted that encapsulated ingredients (proteins/peptides) should remain stable throughout the lifetime of the product and be present in a recommended dose. Also, they should be stable when passing through the gastrointestinal system until they reach the required absorption site (Perry & McClements 2020). Another important aspect to mention is that proteins for medical applications (pharmaceutical industry) generally use synthetic polymers, lipids, and surfactants. Usually, these are limited to specific functionalities. On the other hand, in the food industry, only ingredients that are generally recognised as safe can be utilised (Perry & McClements 2020).
Bovine serum albumin–PLGA microspheres are a promising approach in drug delivery and biomedical applications. Bovine serum albumin serves as a model protein, while PLGA, a biodegradable and biocompatible polymer, acts as the carrier matrix. These microspheres are utilised to achieve controlled and sustained release of therapeutic proteins and drugs, improving their stability and bioavailability. The BSA-PLGA system is particularly valuable in vaccine delivery, where it can protect antigens from degradation and provide a steady release, enhancing the immune response. Poly(lactic-co-glycolic acid) is widely used in biomedical applications due to its biodegradability and biocompatibility. However, concerns about its biological toxicity, particularly during degradation, have been raised. As PLGA degrades, it produces lactic acid and glycolic acid, which can lead to local acidity in tissues. This acidic environment may cause inflammation, irritation, or toxicity, especially in sensitive tissues or when used in large quantities. Despite these potential issues, PLGA is generally considered safe, and its toxicity can be managed through careful design of the polymer’s molecular weight, copolymer ratio, and rate of degradation.
Typical morphologies of BSA-loaded PLGA microspheres with various volume ratios of the aqueous drug phase and organic PLGA phase are presented in Figure 4. Microsphere morphology is influenced by the aqueous volume ratio; the more the aqueous phase, the less spherical the PLGA microspheres (Yao et al., 2016). A few examples of protein encapsulation using electrospraying are listed in Table 2.
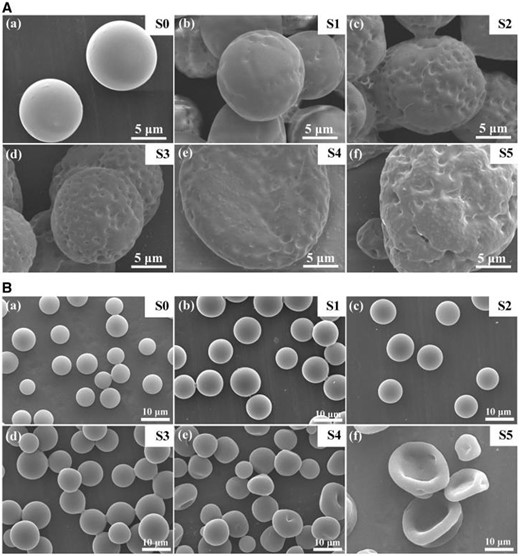
Typical morphologies of bovine serum albumin-loaded poly(lactic-co-glycolic acid) microspheres prepared with different Vw/Vo of 0 (a, S0), 5 (b, S1), 10 (c, S2), 20 (d, S3), 50 (e, S4) and 100 μl/ml (f, S5). The bovine serum albumin concentrations were 0.001 (A) and 0.4 g/ml (B), respectively (Yao et al., 2016).
Examples of various protein encapsulations using the electrospraying technique.
Protein . | Carrier . | Solvent . | Particle size . | Encapsulation efficiency . | Comments . | Reference . |
---|---|---|---|---|---|---|
Bovine serum albumin (BSA) | Not specified | Ethanol/Water | 10–20 μm (droplet relics) | Not specified | No structural change of BSA. Higher protein concentrations showed more stability. Practical processing limits exist as protein concentration increases | (Pareta et al., 2005) |
Bovine serum albumin (BSA) | Polylactide (PLA) | 1,2-Dichloroethane | 0.84–3.95 μm | 22.9%–80.6% | The particle size increased with increasing organic phase ratio while first decreasing and then increasing as the BSA/PLA weight ratio decreased from 1:2 to 1:6 | (Xu & Hanna 2006) |
Bovine serum albumin (BSA) | Alginate and chitosan | Water | 750–2,009 μm | Not specified | Increasing the voltage led to a reduction in both the radius and shell thickness of the capsules. An increase in shell porosity at higher voltages occurred | (Omidi et al., 2010) |
Serum albumin (SA) | Polycaprolactone (PCL), poly(ethylene glycol) (PEG) | Dichloromethane (DCM), chloroform | 3.4–17.9 μm | 7%–40% | PEG content, its molecular weight, and the electrospraying parameters affected the particle size, encapsulation efficiency, and release profiles of the protein | (Bock et al., 2014) |
Bovine serum albumin (BSA) | Poly(D,L-lactide-co-glycolide) (PLGA) | Dichloromethane (DCM), N,N-dimethylformamide (DMF) | 3.0–5.5 μm | 15.7%–25.1% | The molecular weight of PLGA influenced the release. The encapsulation efficiency of BSA was drastically increased by decreasing the concentration of BSA used as the core and by decreasing the flow rate of the core solution | (Zamani et al., 2014) |
Cyclodextrin glucanotransferase (CGTase) | Not specified | Acetate buffer (100 Mm, pH 6) | 75–200 nm | Not specified | Increasing the distance from tip to collector significantly reduces the particle size due to droplet fission | (Saallah et al., 2014) |
Amylase (as a model protein) | Sodium alginate, dextran | Water | Not specified | 30%–47% | All-aqueous electrospray technique generated monodisperse microparticles and microcapsules without using organic solvents Hydrogel particles were fabricated in variable compositions and controlled sizes | (Song et al., 2015) |
Congo red, bovine serum albumin (BSA) | Poly (DL-lactic-co-glycolic acid) (PLGA) | Chloroform | 6.9–9.6 μm | 80%–92% | The morphology and release behaviour of PLGA microspheres were influenced by the volume ratio of the aqueous drug phase and organic PLGA phase and the molecular weight of encapsulated drugs. BSA showed slower release compared to Congo red, indicating that drug release can be controlled by the volume ratio of the aqueous drug phase and organic PLGA phase and drug molecular weight | (Yao et al., 2016) |
Bovine serum albumin (BSA) | Poly-lactic-co-glycolic acid (PLGA) | Acetone for PLGA and ethanol/acetic Acid for BSA | 85–260 nm | Not specified | Decreasing the flow rate and increasing voltage decreased the average size of nanocapsules Capsule size increased with increasing PLGA concentrations in solutions A decrease in polymer concentrations had an important effect on the capsule shape | (Musaei et al., 2017) |
Cytomegalovirus (CMV) pp65 and IE-1 peptides | Poly(D,L-lactide-co-glycolide) (PLGA) | TFE/DMSO with triethylammonium formate (TEAF) for conductivity | 200 nm | 84%–85% | Monodisperse PLGA nanoparticles with high encapsulation efficiency are produced for peptide-based vaccine carriers Peptides were encapsulated without significant structural alteration, retaining their functionality | (Furtmann et al., 2017) |
Alkaline phosphatase (ALP) | Poly(ethylene oxide) | Phosphate-buffered saline | 730–1,290 nm | 85.0%–99.0% | ALP was successfully encapsulated in both monolithic and core-shell structures via electrospinning and electrospraying Coaxial electrospraying of ALP caused a 40% reduction in its bioactivity, attributed to the high voltage used | (Onyekuru et al., 2021) |
Interleukin-1β (pIL-1β) | Alginate, chitosan | Water | 20–60 μm | 40.2%–49.7% | The chitosan layer prevented burst release, enabling a sustained release of pIL-1β for at least 28 days | (Ho et al., 2022) |
Protein . | Carrier . | Solvent . | Particle size . | Encapsulation efficiency . | Comments . | Reference . |
---|---|---|---|---|---|---|
Bovine serum albumin (BSA) | Not specified | Ethanol/Water | 10–20 μm (droplet relics) | Not specified | No structural change of BSA. Higher protein concentrations showed more stability. Practical processing limits exist as protein concentration increases | (Pareta et al., 2005) |
Bovine serum albumin (BSA) | Polylactide (PLA) | 1,2-Dichloroethane | 0.84–3.95 μm | 22.9%–80.6% | The particle size increased with increasing organic phase ratio while first decreasing and then increasing as the BSA/PLA weight ratio decreased from 1:2 to 1:6 | (Xu & Hanna 2006) |
Bovine serum albumin (BSA) | Alginate and chitosan | Water | 750–2,009 μm | Not specified | Increasing the voltage led to a reduction in both the radius and shell thickness of the capsules. An increase in shell porosity at higher voltages occurred | (Omidi et al., 2010) |
Serum albumin (SA) | Polycaprolactone (PCL), poly(ethylene glycol) (PEG) | Dichloromethane (DCM), chloroform | 3.4–17.9 μm | 7%–40% | PEG content, its molecular weight, and the electrospraying parameters affected the particle size, encapsulation efficiency, and release profiles of the protein | (Bock et al., 2014) |
Bovine serum albumin (BSA) | Poly(D,L-lactide-co-glycolide) (PLGA) | Dichloromethane (DCM), N,N-dimethylformamide (DMF) | 3.0–5.5 μm | 15.7%–25.1% | The molecular weight of PLGA influenced the release. The encapsulation efficiency of BSA was drastically increased by decreasing the concentration of BSA used as the core and by decreasing the flow rate of the core solution | (Zamani et al., 2014) |
Cyclodextrin glucanotransferase (CGTase) | Not specified | Acetate buffer (100 Mm, pH 6) | 75–200 nm | Not specified | Increasing the distance from tip to collector significantly reduces the particle size due to droplet fission | (Saallah et al., 2014) |
Amylase (as a model protein) | Sodium alginate, dextran | Water | Not specified | 30%–47% | All-aqueous electrospray technique generated monodisperse microparticles and microcapsules without using organic solvents Hydrogel particles were fabricated in variable compositions and controlled sizes | (Song et al., 2015) |
Congo red, bovine serum albumin (BSA) | Poly (DL-lactic-co-glycolic acid) (PLGA) | Chloroform | 6.9–9.6 μm | 80%–92% | The morphology and release behaviour of PLGA microspheres were influenced by the volume ratio of the aqueous drug phase and organic PLGA phase and the molecular weight of encapsulated drugs. BSA showed slower release compared to Congo red, indicating that drug release can be controlled by the volume ratio of the aqueous drug phase and organic PLGA phase and drug molecular weight | (Yao et al., 2016) |
Bovine serum albumin (BSA) | Poly-lactic-co-glycolic acid (PLGA) | Acetone for PLGA and ethanol/acetic Acid for BSA | 85–260 nm | Not specified | Decreasing the flow rate and increasing voltage decreased the average size of nanocapsules Capsule size increased with increasing PLGA concentrations in solutions A decrease in polymer concentrations had an important effect on the capsule shape | (Musaei et al., 2017) |
Cytomegalovirus (CMV) pp65 and IE-1 peptides | Poly(D,L-lactide-co-glycolide) (PLGA) | TFE/DMSO with triethylammonium formate (TEAF) for conductivity | 200 nm | 84%–85% | Monodisperse PLGA nanoparticles with high encapsulation efficiency are produced for peptide-based vaccine carriers Peptides were encapsulated without significant structural alteration, retaining their functionality | (Furtmann et al., 2017) |
Alkaline phosphatase (ALP) | Poly(ethylene oxide) | Phosphate-buffered saline | 730–1,290 nm | 85.0%–99.0% | ALP was successfully encapsulated in both monolithic and core-shell structures via electrospinning and electrospraying Coaxial electrospraying of ALP caused a 40% reduction in its bioactivity, attributed to the high voltage used | (Onyekuru et al., 2021) |
Interleukin-1β (pIL-1β) | Alginate, chitosan | Water | 20–60 μm | 40.2%–49.7% | The chitosan layer prevented burst release, enabling a sustained release of pIL-1β for at least 28 days | (Ho et al., 2022) |
Examples of various protein encapsulations using the electrospraying technique.
Protein . | Carrier . | Solvent . | Particle size . | Encapsulation efficiency . | Comments . | Reference . |
---|---|---|---|---|---|---|
Bovine serum albumin (BSA) | Not specified | Ethanol/Water | 10–20 μm (droplet relics) | Not specified | No structural change of BSA. Higher protein concentrations showed more stability. Practical processing limits exist as protein concentration increases | (Pareta et al., 2005) |
Bovine serum albumin (BSA) | Polylactide (PLA) | 1,2-Dichloroethane | 0.84–3.95 μm | 22.9%–80.6% | The particle size increased with increasing organic phase ratio while first decreasing and then increasing as the BSA/PLA weight ratio decreased from 1:2 to 1:6 | (Xu & Hanna 2006) |
Bovine serum albumin (BSA) | Alginate and chitosan | Water | 750–2,009 μm | Not specified | Increasing the voltage led to a reduction in both the radius and shell thickness of the capsules. An increase in shell porosity at higher voltages occurred | (Omidi et al., 2010) |
Serum albumin (SA) | Polycaprolactone (PCL), poly(ethylene glycol) (PEG) | Dichloromethane (DCM), chloroform | 3.4–17.9 μm | 7%–40% | PEG content, its molecular weight, and the electrospraying parameters affected the particle size, encapsulation efficiency, and release profiles of the protein | (Bock et al., 2014) |
Bovine serum albumin (BSA) | Poly(D,L-lactide-co-glycolide) (PLGA) | Dichloromethane (DCM), N,N-dimethylformamide (DMF) | 3.0–5.5 μm | 15.7%–25.1% | The molecular weight of PLGA influenced the release. The encapsulation efficiency of BSA was drastically increased by decreasing the concentration of BSA used as the core and by decreasing the flow rate of the core solution | (Zamani et al., 2014) |
Cyclodextrin glucanotransferase (CGTase) | Not specified | Acetate buffer (100 Mm, pH 6) | 75–200 nm | Not specified | Increasing the distance from tip to collector significantly reduces the particle size due to droplet fission | (Saallah et al., 2014) |
Amylase (as a model protein) | Sodium alginate, dextran | Water | Not specified | 30%–47% | All-aqueous electrospray technique generated monodisperse microparticles and microcapsules without using organic solvents Hydrogel particles were fabricated in variable compositions and controlled sizes | (Song et al., 2015) |
Congo red, bovine serum albumin (BSA) | Poly (DL-lactic-co-glycolic acid) (PLGA) | Chloroform | 6.9–9.6 μm | 80%–92% | The morphology and release behaviour of PLGA microspheres were influenced by the volume ratio of the aqueous drug phase and organic PLGA phase and the molecular weight of encapsulated drugs. BSA showed slower release compared to Congo red, indicating that drug release can be controlled by the volume ratio of the aqueous drug phase and organic PLGA phase and drug molecular weight | (Yao et al., 2016) |
Bovine serum albumin (BSA) | Poly-lactic-co-glycolic acid (PLGA) | Acetone for PLGA and ethanol/acetic Acid for BSA | 85–260 nm | Not specified | Decreasing the flow rate and increasing voltage decreased the average size of nanocapsules Capsule size increased with increasing PLGA concentrations in solutions A decrease in polymer concentrations had an important effect on the capsule shape | (Musaei et al., 2017) |
Cytomegalovirus (CMV) pp65 and IE-1 peptides | Poly(D,L-lactide-co-glycolide) (PLGA) | TFE/DMSO with triethylammonium formate (TEAF) for conductivity | 200 nm | 84%–85% | Monodisperse PLGA nanoparticles with high encapsulation efficiency are produced for peptide-based vaccine carriers Peptides were encapsulated without significant structural alteration, retaining their functionality | (Furtmann et al., 2017) |
Alkaline phosphatase (ALP) | Poly(ethylene oxide) | Phosphate-buffered saline | 730–1,290 nm | 85.0%–99.0% | ALP was successfully encapsulated in both monolithic and core-shell structures via electrospinning and electrospraying Coaxial electrospraying of ALP caused a 40% reduction in its bioactivity, attributed to the high voltage used | (Onyekuru et al., 2021) |
Interleukin-1β (pIL-1β) | Alginate, chitosan | Water | 20–60 μm | 40.2%–49.7% | The chitosan layer prevented burst release, enabling a sustained release of pIL-1β for at least 28 days | (Ho et al., 2022) |
Protein . | Carrier . | Solvent . | Particle size . | Encapsulation efficiency . | Comments . | Reference . |
---|---|---|---|---|---|---|
Bovine serum albumin (BSA) | Not specified | Ethanol/Water | 10–20 μm (droplet relics) | Not specified | No structural change of BSA. Higher protein concentrations showed more stability. Practical processing limits exist as protein concentration increases | (Pareta et al., 2005) |
Bovine serum albumin (BSA) | Polylactide (PLA) | 1,2-Dichloroethane | 0.84–3.95 μm | 22.9%–80.6% | The particle size increased with increasing organic phase ratio while first decreasing and then increasing as the BSA/PLA weight ratio decreased from 1:2 to 1:6 | (Xu & Hanna 2006) |
Bovine serum albumin (BSA) | Alginate and chitosan | Water | 750–2,009 μm | Not specified | Increasing the voltage led to a reduction in both the radius and shell thickness of the capsules. An increase in shell porosity at higher voltages occurred | (Omidi et al., 2010) |
Serum albumin (SA) | Polycaprolactone (PCL), poly(ethylene glycol) (PEG) | Dichloromethane (DCM), chloroform | 3.4–17.9 μm | 7%–40% | PEG content, its molecular weight, and the electrospraying parameters affected the particle size, encapsulation efficiency, and release profiles of the protein | (Bock et al., 2014) |
Bovine serum albumin (BSA) | Poly(D,L-lactide-co-glycolide) (PLGA) | Dichloromethane (DCM), N,N-dimethylformamide (DMF) | 3.0–5.5 μm | 15.7%–25.1% | The molecular weight of PLGA influenced the release. The encapsulation efficiency of BSA was drastically increased by decreasing the concentration of BSA used as the core and by decreasing the flow rate of the core solution | (Zamani et al., 2014) |
Cyclodextrin glucanotransferase (CGTase) | Not specified | Acetate buffer (100 Mm, pH 6) | 75–200 nm | Not specified | Increasing the distance from tip to collector significantly reduces the particle size due to droplet fission | (Saallah et al., 2014) |
Amylase (as a model protein) | Sodium alginate, dextran | Water | Not specified | 30%–47% | All-aqueous electrospray technique generated monodisperse microparticles and microcapsules without using organic solvents Hydrogel particles were fabricated in variable compositions and controlled sizes | (Song et al., 2015) |
Congo red, bovine serum albumin (BSA) | Poly (DL-lactic-co-glycolic acid) (PLGA) | Chloroform | 6.9–9.6 μm | 80%–92% | The morphology and release behaviour of PLGA microspheres were influenced by the volume ratio of the aqueous drug phase and organic PLGA phase and the molecular weight of encapsulated drugs. BSA showed slower release compared to Congo red, indicating that drug release can be controlled by the volume ratio of the aqueous drug phase and organic PLGA phase and drug molecular weight | (Yao et al., 2016) |
Bovine serum albumin (BSA) | Poly-lactic-co-glycolic acid (PLGA) | Acetone for PLGA and ethanol/acetic Acid for BSA | 85–260 nm | Not specified | Decreasing the flow rate and increasing voltage decreased the average size of nanocapsules Capsule size increased with increasing PLGA concentrations in solutions A decrease in polymer concentrations had an important effect on the capsule shape | (Musaei et al., 2017) |
Cytomegalovirus (CMV) pp65 and IE-1 peptides | Poly(D,L-lactide-co-glycolide) (PLGA) | TFE/DMSO with triethylammonium formate (TEAF) for conductivity | 200 nm | 84%–85% | Monodisperse PLGA nanoparticles with high encapsulation efficiency are produced for peptide-based vaccine carriers Peptides were encapsulated without significant structural alteration, retaining their functionality | (Furtmann et al., 2017) |
Alkaline phosphatase (ALP) | Poly(ethylene oxide) | Phosphate-buffered saline | 730–1,290 nm | 85.0%–99.0% | ALP was successfully encapsulated in both monolithic and core-shell structures via electrospinning and electrospraying Coaxial electrospraying of ALP caused a 40% reduction in its bioactivity, attributed to the high voltage used | (Onyekuru et al., 2021) |
Interleukin-1β (pIL-1β) | Alginate, chitosan | Water | 20–60 μm | 40.2%–49.7% | The chitosan layer prevented burst release, enabling a sustained release of pIL-1β for at least 28 days | (Ho et al., 2022) |
Solvents, such as chloroform, dichloromethane, and dimethylformamide, are commonly used in the electrospraying technique to dissolve polymers and other materials for the fabrication of micro- and nanoscale particles or fibres. These solvents are favoured due to their volatility, ability to dissolve a wide range of materials, and compatibility with the electrospraying process. However, these solvents pose significant health and environmental risks due to their toxicity and potential for causing respiratory and skin irritation. Careful handling, appropriate ventilation, and solvent recovery systems are essential when using these solvents in electrospraying to minimise exposure and environmental impact.
Usually, commonly used solvents in the process are halogenated (e.g., chloroform, trifluoroethanol) and toxic solvents (e.g., dimethylformamide), which are now restricted by the Chemical Control Regulation in the European Union (Avossa et al., 2022). However, there are examples of successful electrospraying of proteins/peptides using green solvents. For example, deep eutectic solvents can be used as green alternatives to encapsulate proteins/peptides mainly due to their low solvation capacity in conventional solvents via the electrospraying process (Basar et al., 2020). Additionally, BSA has been implemented in alginate and chitosan carriers using a modified method of complex coacervation and electrospray with only water as a solvent. Furthermore, amylase was encapsulated using an all-aqueous electrospray technique within sodium alginate/dextran hydrogels (Song et al., 2015). Another aqueous-based electrospraying setup has been utilised for the preparation of the chitosan-coated alginate microparticles containing pIL-1β (Ho et al., 2022).
Encapsulation
Protein encapsulation is a crucial technique with widespread applications in pharmaceuticals, food science, and biotechnology, facilitating controlled release, improved stability, and targeted delivery. Among the various encapsulation methods, ionic gelation has garnered attention for its simplicity, cost-effectiveness, and biocompatibility. Protein encapsulation may protect proteins from environmental influences, increase bioavailability, and allow for controlled release of medicinal substances; it is highly significant in a variety of industries. Applications include a wide range of products, from the creation of functional food products to medicinal and personal care formulations. Proteins can be encapsulated using a variety of encapsulation processes, including ionic gelation, spray drying, and coacervation. Ionic gelation is the process by which oppositely charged ions interact to generate a gel matrix that contains proteins. With the use of this technique, proteins can be encapsulated in a moderate, regulated environment while maintaining their structural integrity and bioactivity.
Protein encapsulation through ionic gelation finds diverse applications across industries. This technique, known for its simplicity and cost-effectiveness, is particularly valuable in pharmaceuticals, food science, and biotechnology. Ionic gelation has been used in the food industry to encapsulate proteins and improve the stability of vitamins, enzymes, and sensitive bioactive substances. By protecting against deterioration during processing and storage, this application aids in the creation of useful food products and functional food (Jurić et al., 2022).
Ionic gelation is a biotechnology technique to encapsulate and precisely deliver proteins and enzymes. This improves therapeutic and diagnostic uses by protecting bioactive chemicals throughout delivery and facilitating their regulated release at certain places and times.
The encapsulation process is greatly influenced by the characteristics of the protein, including size, charge, and stability. Different interactions between proteins and the gelation matrix can impact the efficacy of encapsulation. The selection and concentration of polymers, like chitosan, alginate, or carrageenan, have a big influence on how the gel matrix forms and stays stable. Protein entrapment and release are influenced by the gelation process, which is influenced by the type and concentration of the polymer. Environmental factors, such as ionic strength and viscosity of the solution, also influence the gelation process. These factors can impact the overall structure, porosity, and stability of the gel matrix, affecting protein encapsulation efficiency.
To achieve high encapsulation effectiveness and customise the properties of the gel matrix in protein delivery systems created using ionic gelation, it is essential to comprehend and optimise these aspects.
The creation of nanoscale delivery systems has been made possible by recent developments, such as the incorporation of nanotechnology into ionic gelation procedures. Modified ionic gelation techniques produce nanoparticles with increased stability, regulated release, and higher bioavailability (Sattayapanich et al., 2022).
LCA and TRL
An LCA assesses the environmental impact throughout the life cycle of a commercial product, service, or process. A product’s LCA is often conducted on the entire process known as cradle-to-grave, which is the more extensive version of an LCA starting from the “cradle,” where the raw material is extracted, until the end of the process, where the waste is disposed. Multiple steps must be considered when performing an LCA and can be split into four distinct stages. Over the last three decades, LCAs have become more standardised with specific requirements and guidelines by the International Organization for Standardization (ISO). This standardisation is essential for straightforward comparisons between different materials.
When it comes to the application of new technologies in the food continuum, the sustainability black box should be considered as an “open design challenge” in line with many aspects that need to be considered (Melles et al., 2022). Djekic and Tomasević (2022) believe that the TRL has an impact on a better understanding of the overall sustainability of new technologies. The European Commission outlines nine TRLs that can be simplified through three stages—proof of concept (from idea to experiment), lab-scale application (including its validation in a lab-scale environment), and full industrial application (Salvador-Carulla et al., 2024). Due to the immaturity of new technologies involved, they usually assess the LCA of a product pro futuro, i.e., when the product becomes mature (Arvidsson et al., 2024). Different authors define different approaches for future-oriented LCA studies such as prospective LCA that has a perspective of future environmental impacts, applying different scenarios (Guinée et al., 2018) or ex-ante LCA focused on up-scaling TRL levels with or without comparisons with existing technologies (van der Giesen et al., 2020). It is obvious that two issues arise: What scenarios were employed, and what type of upscaling models were used? (Arvidsson et al., 2024). It is worth mentioning that novel technologies do not necessarily contribute environmentally, as seen in one of the latest prospective LCA studies, where future scenarios confirmed that only for limited impact categories, a novel application has positive environmental impacts (Eltohamy et al., 2023).
The focus relies on the first step as outlined in ISO 14040 (ISO, 2006)—setting goals and scope in terms of defining the boundaries of the system and its functional unit, as well as in impact assessment depending on future scenario models. There is a need for drafting a consensus guideline on how to validate the sustainability of nonthermal extraction of proteins and later verify its results during exploitation (Djekic & Tomasević 2022). This confirms the conclusion of the 76th LCA Discussion Forum that there is a clear need for developing a common methodology (Adrianto et al., 2021). When it comes to nonthermal extraction of proteins there are no clear guidelines on how to perform LCA depending on TRL level with limited studies found in the literature that have food proteins in focus such as in the case of microbial protein production via power-to-food approaches (Sillman et al., 2020).
Conclusion
The SDGs encompass critical aspects of fostering a more sustainable and equitable world. Food scientists and researchers can contribute to more sustainable processing by digitalisation, optimisation of processing, energy-efficient technological processing, and sustainable use of raw materials, i.e., upcycling and lowering emissions. An LCA assesses the environmental impact throughout the life cycle of a commercial product, service, or process.
Many agroindustrial discards and by-products of plant and animal origins have been researched as sources for the extraction of proteins, such as oil-industrial waste like sesame cake, coconut cake, or flaxseed cake and plant waste from fruits, vegetables, cereals, and legumes processing, including leaves, peels, husks, fungi waste, and dairy waste. Extracting protein from agroindustrial discards is a complex matter, and there is a need for a comprehensive approach that considers various viewpoints on extraction. This approach should prioritise the nutritional value, the amount produced, and the functionality of the extracted protein. In essence, sustainability in protein extraction not only aligns with the broader goals of sustainable development but also offers tangible benefits in terms of food security and environmental and economic benefits. By embracing eco-innovation and leveraging untapped resources such as agroindustrial discards and by-products of plant and animal origins, there is an urgent need to pave the way towards a more resilient and equitable future for generations to come.
In terms of innovation in extraction, there are modern extraction technologies. The conventional extraction methods mainly use different chemicals (alkali, solvents, detergents) that may lead to the degradation of proteins. Nonthermal technologies, like US, plasma, and PEF, are clean and environmentally friendly. Thanks to the innovative power and low cost of these technologies, which meet all the requirements for sustainable development, there are numerous examples of the extraction of biomolecules such as polysaccharides, polyphenols, aromatic compounds, oils, carotenoids, and proteins from different plants and by-products of food processing, which include plant and animal origins. Plant-derived proteins and waste have emerged as sustainable alternatives for food systems, with efficient extraction being crucial for meeting the demand for protein-based products with specific functional properties. The tendency is to replace animal proteins with plant proteins, which are more sustainable and profitable. The focus is on the soluble protein RuBisCO, which has high nutritional values and in vitro digestibility.
These findings highlight the potential of optimised, focused, digitalised NTP in improving protein extraction from plant sources. The idea is to target specific peptides and short proteins that show the bioactivity potential (bioactive peptides). To analyse data, bioinformatics databases are very important tools in the field of food and related biological research. They also provide a valuable contribution to other research fields, including engineering science and technology.
Therefore, it is necessary to monitor and control digitalised nonthermal extraction processes using IoT hardware (sensors and logical unit) and software. During the process, it is necessary to store data, obtain values (energy consumption, processing time, temperature, emissions, etc.), and improve extraction processes towards a higher yield of proteins/peptides with high sustainability parameters.
This is vital for the operationalisation of sustainable development in the sense that it enables the development of innovative new production ideas, new and creative jobs, pollution, ecological footprint reduction, etc. Circular economy is therefore a concept that fully supports the economic, social, and ecological goals and criteria of sustainable development.
Nonthermal processing technology stands out for its low energy usage, simple scalability, and suitability for continuous flow operations. Recent literature underscores the potential of PEF in enhancing protein extraction to meet the growing need for alternative proteins with enhanced functionalities. Nevertheless, further research is needed to fine-tune process parameters. This entails meticulous monitoring and adjustment of key electrical parameters, temperature, and pH to tailor the extraction process to different plant matrices, aiming for improved protein yields and desired techno-functional attributes.
It is also necessary to select optimal solvents, and COSMO-RS is an example of a powerful and versatile model that can be used to predict a wide range of thermodynamic properties. The model is relatively simple to use and can be applied to a wide variety of systems.
After extraction and obtaining proteins and peptides, it is important to pack and deliver the valuable compounds. One can also deliver small molecules like pharmaceutical drugs. Sustainable delivery systems like electrospinning, electrospraying, and encapsulation are promising methods for producing polymer fibres, from submicron to nanoscale sizes, and particles from micro- and nanoparticles, offering precise control over fibre shape, orientation, and dimensions. Encapsulation is a crucial technique with widespread applications facilitating controlled release, improved stability, and targeted delivery of proteins, peptides and small molecules.
It is necessary to keep up with new developments in material science, biopolymer chemistry, and nanotechnology to improve the potential for protein encapsulation; electrospraying or spinning is useful to obtain protein structures to be used as food, like also in the case of 3D printing.
Combining new technologies like 3D printing shall lead to developing intelligent delivery methods for exact control over release kinetics and progressing with nanomaterials.
Innovation for sustainability encompasses a wide range of areas, including renewable energy technologies, sustainable agriculture practices, waste management solutions, water conservation methods, and green transportation systems. By investing in research and development and supporting entrepreneurial initiatives, societies can drive progress towards more sustainable and efficient ways of living and doing business. Fostering innovation requires creating an enabling environment that encourages experimentation, collaboration, and knowledge sharing.
Author contributions
Anet Režek Jambrak (Conceptualization, Drafting, Writing, Editing), Marinela Nutrizio (Writing, Editing), Josipa Dukić (Writing, Editing), Ilija Djekić (Writing, Editing), Marko Vinceković (Writing, Editing), Slaven Jurić (Writing, Editing), Gianpiero Pataro (Writing, Editing), Brijesh Tiwari (Writing, Editing), Gulden Goksen (Writing, Editing), Mojca Čakić Semenčić (Writing, Editing), Anita Slavica (Writing, Editing), Iva Sabljak (Writing, Editing), Janko Diminić (Writing, Editing), Ena Pecina (Writing, Editing), Andrija Sabol (Writing, Editing), Anne-Sylvie Fabiano Tixier (Writing, Editing), and Francesco Donsi (Writing, Editing)
Funding
This research was funded by the Croatian Science Foundation project, IP-2022-10-2207: DEEP - Digitalisation of nonthermal Extraction of proteins from plant by-products and Electroforming as output Product. The work of doctoral student Josipa Dukić has been fully supported by the ``Young researchers' career development project-training of doctoral students'' of the Croatian Science Foundation (DOK-2021-02).
Conflicts of interest
None declared.
Acknowledgements
Bolded references annotate the most important references for this review, as they show engineering aspects of Rubisco to enhance CO2 utilisation, nonthermal technologies potential (like pulsed electric field technology) for recovery of proteins from waste plant resources and application of i.e., electrospun plant protein-based nanofibres. Also, the cited references show the importance of bioinformatics and digitalisation in data mining and big data analysis, and potential of future-oriented life cycle assessment in sustainability valorisation and calculations.
References
Saha, J., & Deka, S. C. (
Sarkis, J. R., Boussetta, N., Blouet, C., Tessaro, I. C., Marczak, L. D. F. & Vorobiev, E. (