-
PDF
- Split View
-
Views
-
Cite
Cite
Yanli Wang, Yuxiang Bai, Jingjing Dong, Hangyan Ji, Jialin Liu, Zhengyu Jin, Partial hydrolysis of waxy rice starch by maltogenic α-amylase to regulate its structures, rheological properties and digestibility, International Journal of Food Science and Technology, Volume 58, Issue 9, September 2023, Pages 4881–4890, https://doi.org/10.1111/ijfs.15920
- Share Icon Share
Abstract
Although different types of starch have been modified with maltogenic α-amylase (MGA), its behaviour on waxy rice starch (WRS) remains obscure. This study aimed to modify cooked WRS with high concentration using MGA. The molecular weight of WRS decreased from 1.8 × 107 to 4.1 × 106 Da due to the exo–endo activity of MGA, while a high proportion of short branch chain and branching degree were generated as the degrees of hydrolysis (DH) increased. Intriguingly, no retrogradation occurred for the hydrolysates, even at DH 4.65%. Rheology reveal that the hydrolysate systems exhibited better fluidity and thixotropy. Furthermore, the resistant starch content of cooked WRS was elevated by 10.43% after MGA modification. The results indicate that MGA-modified WRS was superior to other starches that have been reported in terms of inhibition of retrogradation and resistant starch content. Our data demonstrate the potential of enzyme-based protocol for WRS modification using MGA alone to acquire clean and functional starch.
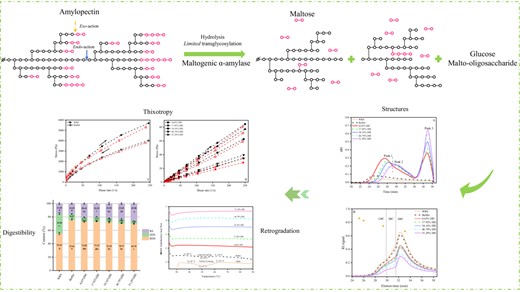
Introduction
Waxy rice, also known as glutinous or sticky rice, is available to produce traditional zongzi, commercial instant food and seaweed rice etc. (Li et al., 2014). Starch, as the major ingredient, accounts for nearly 90% of rice in the dry basis, which plays a decisive role in the quality of rice products (Bao et al., 2004). Waxy rice starch (WRS) is almost entirely composed of amylopectin with low gelatinisation temperature, good water retention and high transparency (Sasaki et al., 2017; Hsieh et al., 2019). However, the texture of cooked waxy rice is cohesive, and waxy rice products are prone to retrograde during storage, which are undesirable in commercial applications. Thus, starch modification is often necessary to overcome the shortcomings of native starch.
Recently, enzymatic modification has attracted considerable attention since it not only meets the demand of clean–label starch ingredients, but endows the customised functional properties to starch products (Deng et al., 2012; Hayashi et al., 2014). Among various types of starch-related enzymes, maltogenic α-amylase (MGA; EC 3.2.1.133) from GH13 has received growing interest due to its versatile catalytic performance. In addition to the primary exo-α-1,4-hydrolysis activity liberating maltose, the enzyme also shows endo-hydrolysis activity towards accessible intramolecular bonds (Leman et al., 2005; Goesaert et al., 2009; Keeratiburana et al., 2020). Furthermore, transglycosylation activity of MGA at moderate substrate concentration has been reported owing to its structural similarity to cyclodextrinases (Park et al., 2000; Lee et al., 2008). At present, several studies have confirmed the anti-staling effect of MGA alone, that is shorting the length of amylopectin side chains to slow down retrogradation, on different kinds of starch, such as pulse starch (Li et al., 2021), potato starch (Chen et al., 2020), waxy maize starch (Grewal et al., 2015) and normal rice starch and rice meal (Li et al., 2014). Besides, MGA has also been employed to reduce the amylolytic digestibility of normal corn starch and pulse starch (Miao et al., 2014; Li et al., 2021). Above all, MGA is an effective tool for regulating the physiochemical and functional properties of starch. Although MGA has been utilised to modify different types of starch, its role in WRS is unclear.
This study, therefore, was conducted to elucidate the influence of commercial MGA from Bacillus subtilis on WRS. Specifically, the structure, morphology, thermal and rheological properties, as well as in vitro digestibility of hydrolysates with different degrees of hydrolysis, were comprehensively characterised. The new knowledge will be of great significance for WRS to develop clean–label starch products with enhanced functional attributes, which would contribute to expanding the new markets of WRS in the processing industry.
Materials and methods
Materials
Waxy rice starch (about 97% amylopectin, moisture of 11.44%) was supplied from Jiangsu Baobao Group Co. (Suqian, China). MGA from Bacillus subtilis (10 000 MANU/g product) was acquired from Novozymes (Beijing, China). Debranching pullulanase (700 U mL−1) and isoamylase from Pseudomonas sp. (200 U mL−1) were obtained from Megazyme (Wicklow, Ireland) and Novozymes, respectively. Amyloglucosidase (EC3.2.1.3, 260 U mL−1) from Aspergillus niger and α-amylase from hog pancreas (EC3.2.1.1, 50 U mg−1) were procured from Sigma-Aldrich (St. Louis, MO, USA). The glucose assay kit was acquired from Megazyme (Wicklow, Ireland). All other reagents and chemicals were of analytical grade.
Starch amylolysis assay
Waxy rice starch slurry (25%, w/v) was prepared by mixing 10 g of WRS and 100 mm sodium acetate buffer containing 5 mm CaCl2 (pH 5.0) in a final volume of 40 mL, followed by stirring in a boiling water bath for 40 min to fully gelatinisation. After cooling to 60 °C, the amylolysis process was triggered by adding MGA (8 U g−1) to the slurry. The incubation was carried out for 15, 30, 60, 120 and 180 min, and the corresponding degrees of hydrolysis (DH) were 4.65%, 17.92%, 34.16%, 46.79% and 51.29%, respectively. Percentage DH was calculated according to the total sugar content released at any given time dividing by the initial starch weight. The enzyme was terminated in a boiling water bath for 20 min. The hydrolysates were cooled to ambient temperature (25 °C) and then lyophilised at −55 °C for 48 h at 1 Pa. The lyophilised equipment used was purchased from Ningbo Scientz Biotechnology Co., Ltd. (SCIENTZ-10 N, Zhejiang, China). The obtained samples were ground, passed through a 100-mesh sieve (150 μm), and kept in a desiccator for subsequent analysis. Two control samples were used: (i) native WRS; (ii) WRS in the sodium acetate buffer through the same procedure without adding MGA (Buffer).
Size exclusion chromatography (SEC)
Molecular weight distributions of the samples before and after debranching were analysed using a high-performance size exclusion chromatography (HPSEC). For debranched samples, 50 mg of starch powder was dispersed in 5 mL of 90% DMSO aqueous solution and boiled in a water bath for 1 h with gentle stirring. Then, 25 mL of anhydrous ethanol was added to precipitate starch molecules. After centrifugation at 8000 g for 20 min, the sediments were carefully collected. The liquid residue was removed through inverting the centrifuge tube on clean paper for 15 min. The obtained starch pellets were re-dissolved in 5 mL of 50 mm sodium acetate buffer (pH 4.5) and boiled for 10 min. Subsequently, the samples were debranched with isoamylase (2 U) and pullulanase (7 U) at 42 °C for 24 h, and the enzymes were terminated by a boiling water bath for 15 min. The fully debranched starch fractions were precipitated by adding twenty volumes of anhydrous ethanol. After centrifugation at 8000 g for 20 min, the collected pellets were re-dispersed in 10 mL of ultra-pure water, boiled for 30 min and then filtered through a 0.45 μm disc filter. A 100-μL aliquot of debranched sample was automatically injected into a HPSEC system. Shodex OHpak 804 HQ and 802.5 HQ (Showa Denko K·K.) were connected in tandem and equilibrated at 60 °C. Elution was carried out at a flow rate of 0.5 mL min−1 using 100 mm NaNO3 as mobile phase. A series of dextran standards (1.0 × 103, 5.0 × 103, 2.5 × 104, 5.0 × 104, 2.7 × 105 and 6.7 × 105 Da) were employed to calibrate the HPSEC system.
The unbranched samples were dissolved in ultra-pure water (5 mg mL−1), and boiled for 30 min, followed by filtering through a 0.45-μm disc filter. Shodex OHpak 806 HQ and 804 HQ (Showa Denko K·K.) were connected in series to examine the molecular weight distributions.
High-performance anion exchange chromatography-pulsed amperometric detection (HPAEC-PAD)
Samples (2 mg mL−1) were boiled for 30 min and then debranched with isoamylase (2 U) at 42 °C for 24 h. The obtained supernatant was injected onto a CarboPac PA-200 column equipped with an HPAEC-PAD (Dionex, Sunnyvale, CA, USA) system after passing through a 0.45 μm membrane. The chain length distributions were computed by the peak integration method using Chromeleon Console 7.2.5 program package.
Proton nuclear magnetic resonance (1H NMR) spectroscopy
One-dimensional 1H NMR spectra of all samples were recorded using a Bruker Avance III 400 MHz NMR spectrometer (Bruker Biospin, Rheinstetten, Germany). Sample (10 mg mL−1) was dispersed in deuterium oxide (D2O), boiled for 1 h and lyophilised. After repeating the procedure twice, the freeze-dried samples were suspended in 0.5 mL of D2O (containing 1.0 mg mL−1 3–(Trimethylsilyl) propionic-2,2,3,3-d4 acid sodium salt (TSP)) by boiling for 1 h. Then, the solution was transferred into a 5-mm NMR glass tube and analysed immediately with 64 scans at 60 °C. The regions of signal representing anomeric protons were integrated by MestReNova software to calculate the branching degree of starch samples (α-1,4: δ 5.24–5.55; α-1,6: δ 4.85–5.03; α- and β-anomeric reducing end protons: δ 5.03–5.16 and δ 4.58–4.69, respectively).
Scanning electron microscopy (SEM)
The morphology of each sample was visualised by SEM (mode JSM–7600 F, JEOL Techniques, Tokyo, Japan). All samples were sprinkled on double-sided adhesive tape and sputtered with gold. Morphological images were captured under vacuum with an accelerated voltage of 5 kV at 500-fold magnification. (See in the Supporting Material).
Retrogradation
Retrogradation profiles of all samples were recorded using a differential scanning calorimeter (DSC 7000X, HITACHI, NSK, Tokyo, Japan). Each sample (3 mg) with 4.5 μL of distilled water was sealed in a DSC aluminium pan and gelatinised by scanning at 120 °C. An empty pan was employed as a reference. Before testing, the heat flow curve of the instrument was calibrated with an indium standard. After 7 days of storage at 4 °C, the second scan was run at a rate of 10 °C min−1 ranging from 20 to 120 °C to determine the retrogradation.
Rheological measurements
The dynamic and steady rheological analyses were performed using a rheometer (DHR-3, TA Instruments Ltd., Crawley, UK) equipped with a 40 mm diameter plate geometry sensor. Suspensions of all samples (30%, w/v) were completely gelatinised and then stored at 25 °C overnight. Frequency sweeps were measured in the range of 0.1–200 rad s−1 at 25 °C. Profiles of storage modulus (G′), loss modulus (G′′) and complex viscosity (η*) were collected at 1% strain (in the linear viscoelastic region; Wang et al., 2022).
Steady-state flow behaviours were carried out ranging from 0.1 to 600 s−1 at 25 °C. The experimental data were fitted with the Power Law model:
where σ is the shear stress (Pa), γ is the shear rate (s−1), K is the consistency constant (Pa·sn), and n is the flow behaviour exponent (Wang et al., 2021). The model fitting was evaluated according to the coefficient R2. In addition, thixotropy of all samples was conducted following the method described previously (Wang et al., 2022).
In vitro digestibility
In vitro digestibility of all cooked samples was determined as described by Englyst et al. with minor alternations (Ji et al., 2021). Briefly, 600 mg of starch was suspended in 15 mL of sodium acetate buffer (0.2 M, pH 5.2, containing 1 mm CaCl2) and then boiled for 30 min to cook the starch. After equilibration at 37 °C for 15 min, fresh enzyme solution (5 mL) containing α-amylase (290 U mL−1) and amyloglucosidase (3 U mL−1) was added to initiate the assay. After 0, 20 and 120 min, aliquots (100 μL) were taken and mixed with 900 μL of anhydrous ethanol to stop the enzymes. The mixture was centrifuged at 10 000 g for 5 min. Megazyme D-Glucose Assay Kit was applied to quantify the amount of glucose liberated from the amylolysis in the supernatant at 20 and 120 min. The content of starch fractions (RDS, SDS and RS) was calculated using the following equations:
where G0, G20 and G120 are the total mass of glucose released by amylolysis at 0, 20 and 120 min, respectively.
Statistical analysis
Differences between samples were analysed by one-way Anova. Data were evaluated using Tukey's multiple comparison (P < 0.05). All experiments were performed in triplicate except for the NMR result. Software IBM SPSS version 23.0 (IBM Corp., Armonk, NY, USA) was employed for statistical analysis.
Results and discussion
Molecular size distribution
Molecular weight (Mw) distribution of WRS with high concentrations (25%, w/v) was investigated during MGA hydrolysis (Fig. 1a). For the control WRS, only one peak was detected by HPSEC, since the raw material only contains amylopectin. The hydrolysates exhibited unique Mw distribution. As shown in Fig. 1a, the area of original amylopectin in WRS (peak 1, at ~28 min) decreased, while the response of a fraction with lowered Mw (peak 2, at ~30.5 min) and low Mw saccharides (peak 3, at ~37 min) rose with increasing DH (Fig. 1a). This phenomenon is related to the exo–endo action of the enzyme. At DH < 46.79%, the exo-α-1,4-glucanase activity was dominant, which shortened the side chains of WRS. However, the response of the hydrolysates at peak 2 was gradually higher than that of the original amylopectin (peak 1) after DH ≥46.79%, indicating the endo-glucanase activity of the enzyme. Notably, the endo-action of MGA was inclined to split the long polymer chains of starch connecting the clusters since low Mw dextrins (between peak 2 and peak 3) were almost absent. Detailed Mw information and z-average radius of gyration (Rz) of all samples are listed in Tables 1 and S1. MGA treatment substantially diminished the Mw of WRS from 1.81 × 107 to 1.90 × 106 Da, which is positively correlated with DH. The Mw distributions are similar to previous study (Grewal et al., 2015). Particularly, although the hydrolysis pattern of the enzyme was not affected by the high substrate concentration (25%) in the present study, the DH value for the transition from exo to endo increased to 46.79%, compared with the study by Grewal et al. (2015; 2% of waxy maize starch, 10% DH).
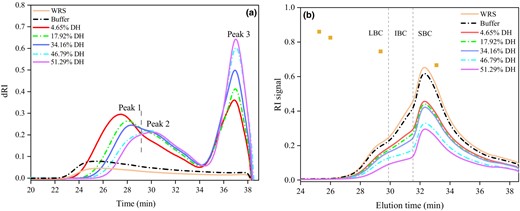
Normalised high-performance size exclusion chromatograms (HPSEC) of non-debranched (a) and debranched (b) WRS after different DH. The dash lines separate long (LBC), intermediate (IBC) and short (SBC) branch chains of amylopectin (B).
Molecular-weight distributions of non-debranched and debranched WRS at different DH
Samples %DH . | Peak 1 . | Peak 2 . | Peak 3 . | Amylopectin branch-chain-length distribution (%)* . | ||
---|---|---|---|---|---|---|
Mw × 106 (Da) . | Mw × 105 (Da) . | Mw × 105 (Da) . | LBC . | IBC . | SBC . | |
WRS | 18.14 ± 0.09a | – | – | 13.3 ± 0.2Ca | 17.6 ± 0.1Ba | 68.9 ± 0.3Ad |
Buffer | 16.03 ± 0.08b | – | – | 13.1 ± 0.1Ca | 17.1 ± 0.4Ba | 69.9 ± 0.5Ad |
4.65 | 3.73 ± 0.04c | 10.97 ± 0.07a | 3.53 ± 0.10a | 12.7 ± 0.2Cab | 16.7 ± 0.3Ba | 70.7 ± 0.4Ad |
17.92 | 3.29 ± 0.02d | 9.07 ± 0.07b | 2.42 ± 0.09b | 12.4 ± 0.2Cbc | 16.3 ± 0.3Bab | 71.4 ± 0.4Acd |
34.16 | 2.31 ± 0.01e | 5.64 ± 0.05c | 1.34 ± 0.03c | 12.0 ± 0.1Ccd | 15.5 ± 0.3Bcd | 72.7 ± 0.4Abc |
46.79 | 1.90 ± 0.01f | 3.83 ± 0.06d | 0.88 ± 0.03d | 11.8 ± 0.2Cd | 14.9 ± 0.2Bd | 73.1 ± 0.4Ab |
51.29 | 1.85 ± 0.02f | 3.34 ± 0.06e | 0.86 ± 0.03d | 9.6 ± 0.0Ce | 13.4 ± 0.2Be | 77.1 ± 0.3Aa |
Samples %DH . | Peak 1 . | Peak 2 . | Peak 3 . | Amylopectin branch-chain-length distribution (%)* . | ||
---|---|---|---|---|---|---|
Mw × 106 (Da) . | Mw × 105 (Da) . | Mw × 105 (Da) . | LBC . | IBC . | SBC . | |
WRS | 18.14 ± 0.09a | – | – | 13.3 ± 0.2Ca | 17.6 ± 0.1Ba | 68.9 ± 0.3Ad |
Buffer | 16.03 ± 0.08b | – | – | 13.1 ± 0.1Ca | 17.1 ± 0.4Ba | 69.9 ± 0.5Ad |
4.65 | 3.73 ± 0.04c | 10.97 ± 0.07a | 3.53 ± 0.10a | 12.7 ± 0.2Cab | 16.7 ± 0.3Ba | 70.7 ± 0.4Ad |
17.92 | 3.29 ± 0.02d | 9.07 ± 0.07b | 2.42 ± 0.09b | 12.4 ± 0.2Cbc | 16.3 ± 0.3Bab | 71.4 ± 0.4Acd |
34.16 | 2.31 ± 0.01e | 5.64 ± 0.05c | 1.34 ± 0.03c | 12.0 ± 0.1Ccd | 15.5 ± 0.3Bcd | 72.7 ± 0.4Abc |
46.79 | 1.90 ± 0.01f | 3.83 ± 0.06d | 0.88 ± 0.03d | 11.8 ± 0.2Cd | 14.9 ± 0.2Bd | 73.1 ± 0.4Ab |
51.29 | 1.85 ± 0.02f | 3.34 ± 0.06e | 0.86 ± 0.03d | 9.6 ± 0.0Ce | 13.4 ± 0.2Be | 77.1 ± 0.3Aa |
Percentages of long, intermediate and short branch chains were calculated as the integral value of the respective fractions peak area of amylopectin branch chains as shown in Fig. 1; in the same column, the numbers with different small letter are significantly different at P < 0.05; in the same row, the numbers with different capital letter are significantly different at P < 0.05.
Molecular-weight distributions of non-debranched and debranched WRS at different DH
Samples %DH . | Peak 1 . | Peak 2 . | Peak 3 . | Amylopectin branch-chain-length distribution (%)* . | ||
---|---|---|---|---|---|---|
Mw × 106 (Da) . | Mw × 105 (Da) . | Mw × 105 (Da) . | LBC . | IBC . | SBC . | |
WRS | 18.14 ± 0.09a | – | – | 13.3 ± 0.2Ca | 17.6 ± 0.1Ba | 68.9 ± 0.3Ad |
Buffer | 16.03 ± 0.08b | – | – | 13.1 ± 0.1Ca | 17.1 ± 0.4Ba | 69.9 ± 0.5Ad |
4.65 | 3.73 ± 0.04c | 10.97 ± 0.07a | 3.53 ± 0.10a | 12.7 ± 0.2Cab | 16.7 ± 0.3Ba | 70.7 ± 0.4Ad |
17.92 | 3.29 ± 0.02d | 9.07 ± 0.07b | 2.42 ± 0.09b | 12.4 ± 0.2Cbc | 16.3 ± 0.3Bab | 71.4 ± 0.4Acd |
34.16 | 2.31 ± 0.01e | 5.64 ± 0.05c | 1.34 ± 0.03c | 12.0 ± 0.1Ccd | 15.5 ± 0.3Bcd | 72.7 ± 0.4Abc |
46.79 | 1.90 ± 0.01f | 3.83 ± 0.06d | 0.88 ± 0.03d | 11.8 ± 0.2Cd | 14.9 ± 0.2Bd | 73.1 ± 0.4Ab |
51.29 | 1.85 ± 0.02f | 3.34 ± 0.06e | 0.86 ± 0.03d | 9.6 ± 0.0Ce | 13.4 ± 0.2Be | 77.1 ± 0.3Aa |
Samples %DH . | Peak 1 . | Peak 2 . | Peak 3 . | Amylopectin branch-chain-length distribution (%)* . | ||
---|---|---|---|---|---|---|
Mw × 106 (Da) . | Mw × 105 (Da) . | Mw × 105 (Da) . | LBC . | IBC . | SBC . | |
WRS | 18.14 ± 0.09a | – | – | 13.3 ± 0.2Ca | 17.6 ± 0.1Ba | 68.9 ± 0.3Ad |
Buffer | 16.03 ± 0.08b | – | – | 13.1 ± 0.1Ca | 17.1 ± 0.4Ba | 69.9 ± 0.5Ad |
4.65 | 3.73 ± 0.04c | 10.97 ± 0.07a | 3.53 ± 0.10a | 12.7 ± 0.2Cab | 16.7 ± 0.3Ba | 70.7 ± 0.4Ad |
17.92 | 3.29 ± 0.02d | 9.07 ± 0.07b | 2.42 ± 0.09b | 12.4 ± 0.2Cbc | 16.3 ± 0.3Bab | 71.4 ± 0.4Acd |
34.16 | 2.31 ± 0.01e | 5.64 ± 0.05c | 1.34 ± 0.03c | 12.0 ± 0.1Ccd | 15.5 ± 0.3Bcd | 72.7 ± 0.4Abc |
46.79 | 1.90 ± 0.01f | 3.83 ± 0.06d | 0.88 ± 0.03d | 11.8 ± 0.2Cd | 14.9 ± 0.2Bd | 73.1 ± 0.4Ab |
51.29 | 1.85 ± 0.02f | 3.34 ± 0.06e | 0.86 ± 0.03d | 9.6 ± 0.0Ce | 13.4 ± 0.2Be | 77.1 ± 0.3Aa |
Percentages of long, intermediate and short branch chains were calculated as the integral value of the respective fractions peak area of amylopectin branch chains as shown in Fig. 1; in the same column, the numbers with different small letter are significantly different at P < 0.05; in the same row, the numbers with different capital letter are significantly different at P < 0.05.
The HPSEC profiles of debranched samples are illustrated in Fig. 1b. The curves are split into three areas: Area 1 (~DP 26–308), Area 2 (~DP 13–26) and Area 3 (~DP ≤12), corresponding to long branch chains, intermediate branch chains and short branch chains (LBC, IBC and SBC) respectively. As listed in Table 1, the proportions of LBC, IBC and SBC in the control were 13.3%, 17.6% and 68.9%, respectively. After MGA modification, the levels of the respective LBC and IBC in the hydrolysates reduced to 9.6% and 13.4% respectively, whereas the proportion of SBC enhanced significantly from 68.9% to 77.1% with an increase in DH. Changes in the CLD profiles resulted from the exo-activity by MGA. The result concurs with previous findings, showing a high proportion of SBC (Li et al., 2021; Zhong et al., 2021a, 2021b).
High-performance anion exchange chromatography-pulsed amperometric detection (HPAEC-PAD)
A higher resolution of branched short amylopectin chains in the debranched samples were accurately detected by HPAEC-PAD. The chain length distribution exhibited conspicuous effects of MGA on branch chains of WRS (Fig. S1c and d), supporting the data from SEC (Fig. 1b). As expected, the incubation with MGA shifted the chain distribution towards shorter chains. As a result, the levels of chains with DP < 8 suffered a substantial increase, especially DP 2, whereas chains in the DP 8-32 significantly lowered (Fig. S1D).
Proportion of α-1,6 branch linkages of starch
Four peaks ranging from 4.5 to 5.6 ppm are displayed in the 1H NMR spectra (Fig. 2a). The peaks at 4.95 and 5.35 ppm are associated with anomeric protons of α-1,6 and α-1,4 linkages, respectively (Bai et al., 2011). The percentage of α-1,6 glycosidic bonds in WRS was 5.10%. The result is in good accordance with previous study (Hizukuri, 1986). After MGA modification, the relative ratios of α-1,6 to α-1,4 linkages increased by 0.80%, 1.17%, 1.19%, 1.54% and 1.67%, respectively (Fig. 2b), resulting from the cleavage of α-1,4 glycosidic bonds by MGA. The variation tendency in the proportion of α-1,6 linkage is in agreement with other reported results (Li et al., 2021; Zhong et al., 2021a, 2021b). Besides, MGA also exerts the transglycosylation activity at high substrate concentrations. Hence, in the present study, the limited transglycosylation reaction of MGA under the applied conditions also caused an increase in the α-1,6 branch linkages of the hydrolysates. Moreover, the signals of α- and β-anomeric reducing end protons were identified, corresponding to 5.21 and 4.63 ppm, respectively. Generally, the hydrolysis level of starch is reflected by the intensities of the two signals (Zhong et al., 2021a, 2021b). As shown in Fig. 2a, the intensities of the two signals were enhanced by MGA with increasing DH value.
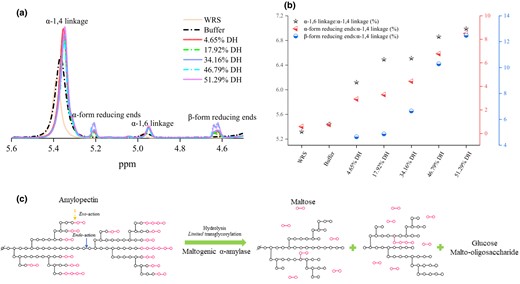
1H NMR spectra of WRS and its hydrolysates (a and b); schematic presentation of the action of MGA on WRS with high concentration (c).
Combining the SEC (Table 1; Fig. 1a) and HPAEC (Fig. S1C and D) results, MGA exhibited exo- and endo-action on the amylopectin of WRS, that is shortening the outer chain to release maltose and cutting off the long inner chain that links the clusters of starch. It is similar to the studies by Grewal et al. (2015) and Villas-Boas et al. (2019). In particular, under high concentration of WRS in our work, the enzyme also exhibited limited transglycosylation, as evidenced by the increased ratio of α-1,6 bonds (Fig. 2a, b). This unique effect of MGA on WRS is schematically depicted in Fig. 2c.
Retrogradation
Waxy rice products such as rice cakes tend to retrogradate during storage, resulting in poor sensory quality and texture, reducing consumer acceptability. As shown in Fig. S3, the endothermic peaks were detected for native (5.02 mJ mg−1) and buffer-treated WRS (1.66 mJ mg−1) from 7-day storage because of the reassociation of amylopectin chains. The onset (To), peak (Tp) and conclusion (Tc) temperatures for native WRS were 45, 51 and 61 °C, respectively. For the buffer-treated WRS, To, Tp, and Tc were 40, 54 and 58 °C, respectively. However, no endothermic heat flow appeared for MGA-modified starch under the same conditions. It is well known that MGA is widely used as an anti-ageing agent in food industry. However, the retrogradation in other reported starches, such as corn starch and pulse starch, tended to decrease gradually after MGA modification, and finally disappeared completely (Grewal et al., 2015; Li et al., 2021). Intriguingly, all of the modified WRS had no retrogradation in our study, even at a DH of 4.65%. According to Hsieh et al. (2019), WRS possesses the lowest retrogradation tendency compared with other commercial waxy starches. In addition, the phenomenon is directly related to the proportion of branch chains with DP 6-24, especially the levels of external chains with DP 6-8, which interfered with the alignment of intra- and inter-molecular chains (Vamadevan & Bertoft, 2018). After MGA modification, the ratio of short chains with DP 6-8 was increased from 7.46% to 12.73%, while the proportion of long chains with DP 12-24 was substantially reduced from 54.53% to 15.07% (Fig. S1C and D). Taken together, the short and long chains in the hydrolysates failed to entwine to form partial crystallites, thus inhibiting the retrogradation.
Frequency sweep
Frequency sweeps (0.1–200 rad s−1) of WRS with different DH were determined at 1.0% strain (within the LVR area). As can be seen from Fig. 3a and b, the native and buffer-treated WRS exhibited viscoelastic solid-like behaviour as the storage modulus (G′) was superior to the loss modulus (G″). For all hydrolysates, G′ was larger than G″ at low frequency region (<1 rad s−1). With increasing frequency (>1 rad s−1), G′′ exceeded G′, and both of them showed a relatively strong frequency dependence. In addition, as the DH value enhanced, the G′ value suffered from attenuation, while the G″ value increased. On the one hand, the breakdown of the amylopectin matrix in swollen starch granules appears to be the determinant for G′ (Keetels et al., 1996). The breakdown ratio is negatively correlation with the degree of swelling of starch granules (Singh et al., 2012). After MGA modification, Mw of WRS decreased significantly (Table 1; Fig. 1a), resulting in the breakdown of amylopectin molecules, as evidenced by SEM result (Fig. S2). Owing to the degradation of WRS, the swelling of amylopectin in the dispersed phase was inhibited during gelatinisation. The volume fraction of the swollen particles became smaller, which hindered the intermolecular interactions, rendering G′ reduce during cooling. On the other hand, G′ may be affected by the aggregation between amylopectin chains, and long amylopectin chains (DP > 50) facilitate the association of starch molecules during cooling (Jane et al., 1999). As previously mentioned, the LBC and IBC of WRS were trimmed by the exo-activity of MGA (Table 1; Figs 1b and S1D), reducing the rearrangement and entanglement effects of the amylopectin chains during cooling. Correspondingly, the complex viscosity (η⁎) declined with increasing frequency and DH (Fig. 3c), indicating an improvement in system fluidity. This result coincides with the results from steady shear flow measurements (Section 3.7).
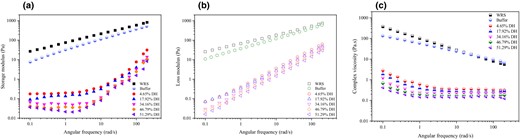
Storage modulus (a), loss modulus (b) and complex viscosity (c) as a function of angular frequency (ω) of WRS and its hydrolysates.
Steady shear flow behaviour
Steady shear flow behaviours of WRS pastes are illustrated in Fig. S4A and B. As shown in Fig. S4A, obviously, the apparent viscosity of all samples dropped with increasing shear rate, showing the shear-thinning behaviour. The apparent viscosity of native WRS reached maximum, followed by the buffer-treated WRS. Comparatively, the viscosity of the hydrolysates diminished slightly along with shear rate increasing. At a given shear rate, the apparent viscosity of the hydrolysate systems tended to decrease with the increase of DH. For the profiles of stress (Fig. S4B), a growing trend in shear rate was observed for all samples. The stress values of the hydrolysates were significantly inferior to that of the control. The result means that MGA treatment weakened the interaction between starch molecules, and disrupted the entanglement of molecular chains, thus achieving the flow of the samples by applying small stress.
The Power Law model was employed to fit the stress curves of the samples, and the parameters are summarised in Table S2. The results reveal that the model adequately fitted the stress–shear rate data well (R2 = 0.9931–0.9998). A greater slope (K value) of the stress–shear rate curve implies a stronger shear thinning behaviour. The K value of WRS paste was the largest (267.915 Pa·sn), suggesting its strong shear thinning behaviour. In comparison, the hydrolysate suspensions showed very low consistency (K values: 0.161–0.478). It reveals that the suspensions treated with MGA were less viscous, and displayed weaker shear-thinning behaviour. However, there was no significant difference in K between the hydrolysates owing to their similar Mw (Table 1). In addition, after MGA modification, the flow behaviour exponent (n) of the samples was elevated remarkably (80%), implying better plasticity of the systems. Similar results were reported for alcohol–alkali-treated WRS suspensions (Chen et al., 2019).
Thixotropy
Thixotropy is one of the important rheological properties. Under shearing force, a hysteresis loop is generated by the area between the upward and downward curves of shear stress vs. shear rate. The area of the hysteresis loop reflects the extent of structural breakdown caused by the shear stress in the destruction and rebuilding of the inner structure in a sample, which remains the shear-thinning behaviour in subsequent shear sweep (Viturawong et al., 2008; Wang et al., 2022). As can be seen from Fig. 4a and b, all samples displayed legible hysteretic loops, indicating thixotropic behaviour. At the same shear rate, the hysteresis loop areas of the hydrolysates (Fig. 4b) were significantly smaller than those of native starch and buffer-treated starch (Fig. 4a). The marked decreases in the hysteresis loop of the hydrolysates resulted from better fluidity of the system owing to the degradation of the molecular structures and the shortening of the molecular chains (Table 1; Figs 1, S1). The flexibility enabled the molecular structures of MGA-modified WRS to be restored rapidly under the same applied shearing (Wang et al., 2010; Fang et al., 2019). Additionally, the hysteresis loop areas of the hydrolysates decreased slightly with increasing DH. It may be attribute to that higher DH value led to stronger fluidity of the system. The result is in agreement with the tendency of the apparent viscosity curves (Fig. S4A).
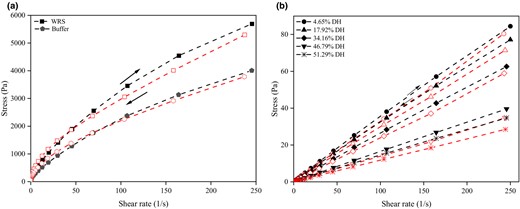
In vitro digestibility
Figure 5 displays the effect of MGA hydrolysis on the digestibility of WRS. In native WRS, the contents of RDS, SDS and RS were 55.24%, 29.83% and 14.93%, respectively. After cooking, the RDS level increased to 75.62%, whereas the content of SDS decreased to 8.83%, and the RS level did not change (P > 0.05), which is in accordance with the study by Zhang et al. (2016). They investigated the digestibility of native WRS, cooked WRS and residual starch during the preparation process of waxy rice vinasse, pointing that the RDS level of WRS increased to 78.88%, while the SDS level decreased to 6.84%, and the RS level did not change (P > 0.05) after cooking. However, modification of MGA led to lower levels of RDS and SDS in the hydrolysates, but different extent. Compared with cooked WRS, the amount of RDS in the hydrolysates decreased by 2.77%, 2.40%, 3.27%, 5.25% and 5.86%, respectively, as the DH increased. The SDS content reduced by 2.10%, 1.60%, 3.01%, 2.82% and 4.57%, respectively. It has been reported that large amounts of SDS could be produced by high level of long chains (DP > 13; Zhang et al., 2008). According to the chain length distribution derived from HPSEC (Fig. 1b, Table 1), the levels of IBC and LBC significantly reduced owing to MGA hydrolysis, leading to a decrease in SDS content. However, compared with the control, the levels of RS after MGA treatment was considerably enhanced by 10.43%, which was higher than that of previously reported pulse starch (RS: ~6%; Li et al., 2021). The phenomenon is associated with the branching degree of starch molecules (Miao et al., 2014; Zhong et al., 2021a, 2021b). As previously mentioned by the 1H NMR result (Fig. 2), a high proportion of α-1,6 branch linkages of the hydrolysates (5.76%–6.55%) was achieved by the enzyme, which could resist the hydrolysis of amylolytic enzymes.
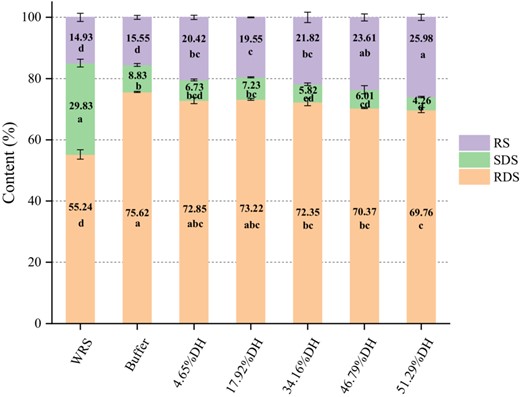
In vitro digestibility of cooked WRS and its hydrolysates. In the same nutrition component, the column with different letter are significantly different at P < 0.05.
Conclusions
In this study, cooked WRS with high concentration was treated using MGA. MGA hydrolysis significantly reduced the molecular weight of WRS and severely disrupted the morphology of WRS. In particular, the inhibitory effect of retrogradation and functional properties of the hydrolysates were superior to other types of starch that have been reported. These phenomena are attributed to the increase in short-chain levels and α-1,6 glycosidic linkage ratio in the hydrolysates. Therefore, no retrogradation occurred in all the hydrolysates, and the RS content of the hydrolysates increased from 15.55% to 25.98%. Furthermore, the frequency dependence and thixotropy of the hydrolysates enhanced, while the viscosity of the systems reduced. In summary, our work provides new insights into tailoring structural and physicochemical features of starch by utilising readily available enzyme.
Acknowledgments
This work was supported by the National Natural Science Foundation of China (grant number 32130084, 32072268), the Science & Technology Pillar Program of Jiangsu Province (BE 2018304) and the Special Funds for Taishan Industry Leading Talent of Shandong Province, China (LJNY 201706).
Author contributions
Yanli Wang: Data curation (lead); formal analysis (lead); investigation (lead); methodology (lead); software (lead); writing – original draft (lead); writing – review and editing (lead). Yuxiang Bai: Conceptualization (lead); project administration (equal); resources (equal). Jingjing Dong: Data curation (supporting); methodology (supporting); validation (supporting); writing – review and editing (supporting). Hangyan Ji: Investigation (supporting); methodology (supporting); software (supporting); validation (supporting). Jialin Liu: Methodology (supporting); visualization (supporting). Zhengyu Jin: Project administration (equal); resources (equal); supervision (lead).
Conflict of interest
The authors declare that they have no conflicts of interest regarding the publication of this paper.
Ethical approval
Ethics approval was not required for this research.
Peer review
The peer review history for this article is available at https://publons.com/publon/10.1111/ijfs.15920.
Data availability statement
The data that support the findings of this study are available from the corresponding author upon reasonable request.
References
In this study, based on the hydrolysis and transglycosylation activity of novel maltogenic amylase CoMA, the relationship between its modification on the structure and retrogradation properties of potato starch was evaluated. This study is helpful for reviewing the research status of maltogenic α-amylase modified starch in our manuscript, and allows us to understand the changes in the structure and properties of potato starch modified by maltogenic α-amylase.
The objective of this study was to determine the degree of hydrolysis (DH) and starch structure after maltogenic α-amylase treatments in relation to its retrogradation. On the one hand, this study could be as a literature review in the introduction, on the other hand, we cited the calculation method of the degree of hydrolysis in this study, and used the degree of hydrolysis to measure the degree of starch modification. Furthermore, we compared the retrogradation properties of the waxy rice starch in our work and waxy maize starch in this study after maltogenic α-amylase modification.
This study was conducted to elucidate structural and functional properties of waxy starches, including tapioca, potato, maize, wheat, and rice. It sheds light on the differences in structure and properties among common waxy starches, as well as the advantages of waxy rice starches. Moreover, it helps us to explain why all the hydrolysates were not retrograded when waxy rice starch is modified with maltogenic α-amylase.
This study aimed to modify granular lentil (LS), faba bean (FBS) and pea (PS) starches with maltogenic α-amylase (MGA) from Bacillus stearothermophilus, and the MGA-modified starches were then characterised and compared with that produced from native normal maize starch (NMS). On the one hand, this study could be as a literature review in the introduction. On the other hand, we referred to the method for the determination of SEC of debranched samples in this study. It gave us a more comprehensive understanding of the product structure after modification of waxy rice starch. In addition, we compared with in vitro digestibility in our paper.
In this research, the structural and thermodynamic properties of an extremely thermostable maltogenic amylase from Thermofilumpendens (TfMA) treated rice starch were investigated firstly. Then, the effect of TfMA used in cooking condition on the retrogradation of gelatinized rice starch and rice meal under low temperature storage was further evaluated. On the one hand, this study could be as a literature review in the introduction. On the other hand, we referred to the assay for in vitro digestion in this study, and compared with the digestibility in our paper.