-
PDF
- Split View
-
Views
-
Cite
Cite
Fangfang Zhang, Wangrui Yi, Jun Cao, Kunming He, Yawen Liu, Xinpeng Bai, Microstructure characteristics of tea seed dietary fibre and its effect on cholesterol, glucose and nitrite ion adsorption capacities in vitro: a comparison study among different modifications, International Journal of Food Science and Technology, Volume 55, Issue 4, April 2020, Pages 1781–1791, https://doi.org/10.1111/ijfs.14465
- Share Icon Share
Abstract
The effects of xylanase–cellulase hydrolysis, ultrasonic modification and enzymatic-ultrasonic treatment on the physicochemical properties, morphological structures and adsorption capacities in vitro of purified fibre (PF) from tea seed were determined. The results showed the ultrasonically treated fibre (UTF) had a higher water-holding capacity (60.15 g g−1), oil-binding capacity (30.42 g g−1), swelling capacity (29.93 mL g−1), emulsification activity (381.36 m2 g−1) and emulsification stability (20.20) than PF, enzymatically hydrolysed fibre (EHF) and enzymatic-ultrasonic treated fibre (EUF). The structures of PF, UTF, EHF and EUF were characterised by scanning electron microscope, Laser particle size analyzer, X-ray diffraction, thermogravimetric analysis and Fourier transform infrared spectra. Furthermore, compared with PF, the adsorption capacity of UTF, EHF and EUF for cholesterol, glucose and nitrite ions during simulative gastrointestinal tract was improved to different degrees. This study can provide guidance for the comprehensive utilisation of byproduct of tea seed and designing novel functional dietary fibre.
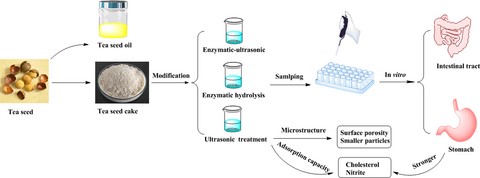
Introduction
Dietary fibre (DF), a natural macromolecular polysaccharide with a complex structure, is not easily digested and absorbed by enzymes produced by the human body, but fermented by bacteria in the colon. Depending on the solubility, DF can be categorised as insoluble dietary fibre (IDF) and soluble dietary fibre (SDF) (Fuentes-Alventosa et al., 2009). Recent studies have shown that an adequate intake of DF can help reduce the risk of diabetes, diarrhoea, cardiovascular disease, obesity and certain cancers (Elleuch et al., 2011). In the food industry, DF is often added to improve nutrition, texture, colour, flavour and sensation. DF can be added to beverages as a thickener, to pharmaceutical products as an excipient or to fermented food to improve the fermentation capacity and product flavour (López-Marcos et al., 2015).
Increasing attention is being paid to DF obtained from agricultural byproducts owing to its good antioxidant capacity, fermentation capacity, and oil adsorption and water retention characteristics (Elleuch et al., 2011). Water-soluble DF can adjust and improve the physiological functions of intestinal microbiota, as well as preventing metabolic syndrome and cardiovascular disease (Galisteo et al., 2008). DF with a higher oil-binding capacity (OBC) and water-holding capacity (WHC) can increase the volume of excrement and provide mechanical stimulation of colonic mucosa. In addition, the intestinal microbiota ferments DF to produce short-chain fatty acids, thereby reducing the risk of obesity, diarrhoea and ulcerative colitis (Peerajit et al., 2012). Furthermore, DF with a strong binding ability effectively promotes the excretion of cholesterol, bile acid and nitrite ions in the gastrointestinal tract, thus reducing the serum concentrations of these compounds (Ou et al., 2001).
Dietary fibre contains various characteristic functional groups, such as aldehydes, ketones, ethers, carboxylic acids and ether linkages, which have strong adsorption abilities for water, oil or toxic metal ions. Typically, DF is modified using physical, chemical or enzymatic methods to increase the exposure of these characteristic groups (Santala et al., 2014). However, the microstructure and physicochemical properties of DF may vary depending on the modification method used (Luo et al., 2018). Studies have shown that xylanase-assisted modification of rice bran fibre effectively reduces the particle size of the DF and improves the WHC, OBC and adsorption capacity for heavy metal ions (Ma & Mu, 2016). Ultrasonic technology is a new modification technology with green environmental protection and high efficiency. It can achieve the effect of depolymerization of biological tissue and entangled complex macromolecules through the cavitation effect and mechanical effect on the substrate generated by ultrasonic wave in the liquid (Liu et al., 2016).
The tea seed that the fruit of tea tree (the leaf is used as tea drinks), from camellia plants, which are widely distributed in East and Southeast Asia, is rich in nutrients and DF. The rapid development of tea seed products has resulted in a large amount of tea seed cake, which is currently discarded as waste. Therefore, tea seed has potential for use as a new type of raw material for DF with good nutritional value and functional characteristics. However, there are been few studies on the extraction, purification and modification of tea seed fibre.
The choice of an appropriate modification method for DF derived from tea seed can enhance the physicochemical properties and physiological functions. The main aims of this study were as follows: (i) to extract and purify the tea seed fibre, (ii) to determine the effects of ultrasonic treatment and complex enzymatic hydrolysis on the microstructure and physicochemical properties of purified tea seed fibre, and (iii) to elucidate the effects of modified tea seed fibre on the functional properties of cholesterol, nitrite ions and glucose adsorption in simulated gastrointestinal digestion in vitro.
Materials and methods
Materials
The tea seed raw material was purchased from a market in Bijie city, Guizhou province, China. α-Amylase (4000 U g−1), amyloglucosidase (100 000 U mL−1), acidic protease (50 U mg−1), xylanase (50 U mg−1) and cellulose (50 U mg−1) were purchased from Aladdin Reagent Co., Ltd. (Shanghai, China). Cholesterol (>99%), trypsin (30 000 U g−1), pancreatic lipase (30 000 U g−1), pepsin (30 000 U g−1), pancreatin (250 U mg−1) and bile salts were purchased from Yuanye Biotechnology Co., Ltd. (Shanghai, China). All the chemical reagents were of analytical grade.
Preparation of PF
The process flow for preparing the PF is shown in Figure S1. Tea seeds were picked, dried and peeled to obtain the sarcocarp. The sarcocarps were pressed at low temperature to obtain tea seed oil using a previously reported method (Yang et al., 2019) with slight modifications. After filtration, the tea seed oil was collected and the remaining solids were dried and ground (60 mesh sieve) to obtain the tea seed cake. The tea seed cake was dried at 50 °C for 5 h in a hot-air drying oven, ground using a grinding machine, and then defatted by Soxhlet extraction with petroleum ether for 4 h. The defatted cake was ground again until it passed through a 60 mesh sieve, and 20 g of this product was added to 400 mL of distilled water in a 1000 mL beaker. Under uniform stirring, the pH of the mixture was adjusted to 6.0 with a 0.1 mol L−1 sodium hydroxide solution. Then, high-temperature α-amylase (iodine solution test of the mixture in the beaker did not produce a blue colour to detect that the starch was completely hydrolysed) was added to the mixture at 95 °C in a water bath shaker, and hydrolysis was allowed to proceed for 30 min at 120 r.p.m. The pH of the hydrolysed product was adjusted to 4.4 using a 0.1 mol L−1 HCl solution. Amyloglucosidase (1 g per 100 g) was added to the sample at a water bath temperature of 60 °C, and the mixture was stirred for 3 h. The water bath temperature was increased to 100 °C for 10 min to destroy enzyme activity. Then, the enzyme solution was adjusted to pH 7.8, alkaline protease (0.5 g per 100 g) was added at a water bath temperature of 55 °C, and the mixture was allowed to stand for 3 h. Finally, the water bath temperature was increased to 100 °C for 10 min to destroy enzyme activity. To obtain the PF, the mixture was filtered three times under vacuum conditions and then the solids were freeze-dried at −80 °C.
Fibre modification
Enzymatic hydrolysis of PF
Enzymatic hydrolysis of PF was conducted using a literature method (Daou & Zhang, 2014) with slight modifications. PF (60 g) suspended in 600 mL of phosphate buffer (0.1 m, pH 5.0) was mixed with 0.3 g of a composite enzyme (xylanase amount: 0.3 g per 100 g, cellulase amount: 0.4 g per 100 g) in a water bath at 50 °C, and the mixture was allowed to hydrolyse for 36 h. Subsequently, the mixture was heated in boiling water for 10 min to destroy enzyme activity and then cooled to room temperature (30 °C). The mixture was filtered three times under vacuum conditions, and then the solids were freeze-dried (−80 °C) to obtain the enzymatically hydrolysed fibre (EHF).
Ultrasonic treatment of PF
A high-power CNC ultrasonic cleaner (KQ-800kde; Kunshan Ultrasonic Instrument Co., Kunshan, China) was used to modify the fibre according to a reported literature method (Moczkowska et al., 2019) with slight modifications. The PF was evenly dispersed in distilled water (1:100, w/w) for 20 min at a temperature of 40 °C and an ultrasonic power of 640 W. After the ultrasonic treatment, the mixture was filtered three times under vacuum conditions, and the solids were freeze-dried at −80 °C to obtain the ultrasonically treated fibre (UTF).
Enzymatic-ultrasonic treatment of PF
The PF was evenly dispersed in distilled water (1:100 w/w) for 20 min at a temperature of 40 °C and an ultrasonic power of 640 W. Then, the sample was treated using the method for preparing the EHF to obtain the enzymatic-ultrasonic treated fibre (EUF).
Analysis of the physicochemical properties of the fibres
Chemical and monosaccharide composition analysis
The moisture content was tested using an HB43-S infrared rapid humidity tester (Mettler Toledo, International Trading Co., Ltd, Shanghai, China). The contents of fat, protein, ash, total dietary fibre (TDF), SDF and IDF were measured according to the American Association of Cereal Chemists official methods (AACC, 1986, and 1995).
The monosaccharide compositions of the PF, EHF, UTF and EUF were measured according to a recently reported method (Chau & Huang, 2003). The fibre (approximately 10 mg) was added to 5 mL of trichloroacetic acid aqueous solution (2 mol L−1). The mixture was hydrolysed at 100 °C for 2 h and then diluted with deionized water. The diluted samples were analysed using an Agilent 1100 HPLC with a (DVD) detector (Agilent Technologies Co., Colorado Springs, CO, USA).
WHC, OBC and swelling capacity (SC) analysis
The pre-dried fibre (1.0 g) was mixed with 70 mL of distilled water. The mixture was stirred and then centrifuged at 5000 g for 10 min. The WHC, OBC and SC of PF, EHF, UTF and EUF were determined using the reported method (Luo et al., 2017).
Emulsifying activity and stability analysis
Using the turbidimetric method described in the literature (Thaiphanit & Anprung, 2016), the emulsifying activity of the fibre (EAF) and the emulsifying stability of the fibre (ESF) were determined. First, 45 mL of a fibre suspension (1 g per 100 mL) was mixed with 15 mL of soybean oil and stirred at 200 g using a homogenizer (T10, Albany, USA) at room temperature for 1 min. After 100-fold dilution with a 0.1% (w/v) sodium dodecylbenzenesulfonate (SDBS) solution, the absorbance at 500 nm was measured (TU-1810; Shanghai APL Instrument Co., Ltd., Shanghai, China), which was considered the initial absorbance value at 0 min. After stirring the suspension for a further 5 min, the sample was again diluted with SDBS, and the measured absorbance was considered as the absorbance value at 5 min. The SDBS solution was used as a blank.
Characterisation of the fibre microstructure
Scanning electron microscopy (SEM) and particle size analysis
The surface morphologies and fracture patterns of the PF, EHF, UTF and EUF were observed using a JEOL JEM 2100 instrument (JEOL Ltd, Tokyo, Japan) according to a literature method (Luo et al., 2018). The fibre samples were prepared by spattering on a sample stub and then coating with sputtered Pt/Pd for 5 min.
The particle sizes of the PF, EHF, UTF and EUF were determined using a Mastersizer 2000 laser particle size analyzer (Clevedon Organs UK Ltd, Malvern, UK). Distilled water was used as a dispersant (refractive index: 1.33).
X-ray diffraction (XRD) analysis
The XRD patterns of the samples were recorded using an X-ray diffractometer (D8 Advance, Bruker, Rheinstetten, Germany) via a method similar to that described in the literature (Sucaldito & Camacho, 2017). The diffractometer was operated at 40 kV and 40 mA in the 2θ range of 5°–60° with an angular step width of 0.02°.
Fourier transform infrared (FT-IR) spectrometry analysis
To analyse the functional groups present in the PF, EHF, UTF and EUF, FT-IR spectra were collected using a Nicolet 5700 infrared spectrometer (Thermo Scientific, Waltham, MA, USA) in the scanning range of 400–4000 cm−1 at a resolution of 4 cm−1. The pre-dried samples were mixed with KBr (1:100, w/w), ground and sliced. The background spectrum was collected using blank KBr.
Thermogravimetric analysis (TGA)
The thermal properties of the PF, EHF, UTF and EUF were determined using a differential scanning calorimeter (Q100 TA, New Castle, DE, USA). The pre-dried sample (3 mg) was placed in an aluminum pot, using an empty aluminum pot as a reference, and heated from 25 to 700 °C at a rate of 20 °C min−1.
In vitro gastrointestinal simulation
Cholesterol adsorption capacity (CAC) analysis
The adsorption properties of the PF, EHF, UTF and EUF for cholesterol in the gastrointestinal tract were determined using the o-phthalaldehyde method recently described in the literatures (Wang et al., 2015; Wang et al., 2018) with minor modifications. The fibre sample (0.1 g) was mixed with diluted yolk solution (20 mL) and adjusted to pH 2.0 with HCl (6 mol L−1). Then, pepsin solution (15 mg of pepsin dissolved in 0.1 mol L−1 of HCl) was added to simulate the environment of human gastric juice. And the mixture was adjusted to pH 7.0 with NaHCO3 (0.5 mol L−1), then 8 mg mL−1 of pancreatin and 50 mg mL−1 of bile salt mixture solution (5 mL) was added to simulate the environment of the human small intestine. Afterwards, samples were shaken at 37 °C for 120 min, heated in boiling water for 10 min to destroy enzyme activity and cooled to room temperature. After centrifuged at 4000 g for 15 min, the absorbance at 550 nm of samples was measured using a spectrophotometer (TU-1810; Shanghai APL Instrument Co., Ltd.), and the cholesterol content was quantified according to the standard curve (y = 0.425x + 0.01, R2 = 0.9976). The cholesterol adsorption capacity (CAC) in fibre sample was calculated as follows:
where C1 is the concentration of cholesterol without adding fibre sample, C2 is the concentration of cholesterol with adding fibre sample, and m0 is the mass of the added fibre sample.
Adsorption capacity of nitrite ions (ACNI) analysis
The adsorption capacities of the fibre samples for nitrite ions in the gastrointestinal tract were determined using reported methods (Zhu et al., 2015; Wang et al., 2018) with slight modifications. The fibre sample (0.1 g) was mixed with sodium nitrite (20 mL, 100 µmol L−1) and stirred evenly. The pH of the mixture was adjusted to 2.0 with HCl (6 mol L−1), and then, pepsin solution (15 mg of pepsin dissolved in 0.1 mol L−1 of HCl) was added to start the gastric digestion. And the mixture was adjusted to pH 7.0 with NaHCO3 (0.5 mol L−1), and then, 8 mg mL−1 of pancreatin and 50 mg mL−1 of bile salt mixture (5 mL) were added to start the small intestine digestion. Afterwards, samples were shaken at 37 °C for 60, 120, 240, 360 and 480 min, respectively, then destroyed enzyme activity in boiling water for 10 min and centrifuged at 4000 g for 15 min. The absorbance at 538 nm of samples was measured using a spectrophotometer (TU-1810; Shanghai APL Instrument Co., Ltd.). The nitrite ions weight was quantified according to the standard curve (y = 0.435x + 0.006, R2 = 0.9993). The adsorption capacity of nitrite ions (ACNI) in fibre sample was calculated by the following formula:
where w1 is the weight of sodium nitrite in solution without adding fibre sample, w2 is the weight of sodium nitrite in solution with adding fibre sample, and m0 is the weight of added fibre sample.
Glucose adsorption capacity (GAC) analysis
The GAC values of the fibre samples were determined by a literature method (Wang et al., 2015). The fibre sample (0.2 g) was mixed with 100 mL of glucose solution (0.1, 0.2, 0.5 and 1.0 mmol L−1, respectively), and the pH of mixture was adjusted to 7.0 with NaHCO3 (0.5 mol L−1). Then, 8 mg mL−1 of pancreatin and 50 mg mL−1 of bile salts mixture (5 mL) were added, and incubated at 37 °C for 6 h. Afterwards, samples were heated in boiling water for 10 min and centrifuged at 4000 g for 15 min. The absorbance at 620 nm of samples was measured using a spectrophotometer (TU-1810; Shanghai APL Instrument Co., Ltd.). The glucose content was quantified according to the standard curve (y = 1.1255x − 0.0089, R2 = 0.9978). The glucose adsorption capacity (GAC) of the fibre sample was calculated by the following formula:
where Ci is the concentration of glucose solution without adding fibre sample, C2 is the concentration of glucose in the supernatant with adding fibre sample, m0 is the weight of fibre sample, and V is the volume of glucose solution.
Statistical analysis
The experimental data were statistically analysed using SPSS 20.0 (SPSS Inc., Quarry Bay, HK, USA). The data are expressed as average values ± standard deviations (n = 9). The statistical significance was evaluated using Duncan’s multiple range tests. A value of P < 0.05 was considered statistically significant. Plots were drawn using Origin Pro Portable 9.0.
Results and discussion
Physicochemical properties analysis
Chemical and monosaccharide composition
The composition of the tea seed cake is shown in Table S1. The main components of the tea seed cake were moisture (4.98%), fat (10.12%), protein (16.12%), ash (5.16%) and TDF (51.91%; 6.62% SDF and 45.29% IDF). Therefore, the tea seed cake was rich in DF and has great potential for applications in the food processing industry.
The monosaccharide compositions of PF, EHF, UTF and EUF are shown in Table 1. The results indicate that the tea seed fibre was rich in arabinose (219.51–261.12 mg g−1 fibre), xylose (40.87–51.01 mg g−1 fibre), galacturonic acid (45.97–62.66 mg g−1 fibre) and lactose (66.21–75.86 mg g−1 fibre). Meanwhile, the contents of total monosaccharides in EHF (498.30 mg g−1 fibre) and UTF (489.70 mg g−1 fibre) were significantly higher than that in PF (458.67 mg g−1 fibre) (P < 0.05). This difference may be caused by degradation of cellulose and hemicellulose in PF via hydrolysis by complex enzymes or via the mechanical and cavitation effects of ultrasonic waves, resulting in an increase of monosaccharide content (Ma & Mu, 2016). However, the total monosaccharide content of EUF was significantly lower than those of PF, UTF and EHF. During the treatment to prepare EUF, the possible reason was that the hydrogen bonds between lignin and hemicellulose were destroyed by ultrasound (Cheikh Rouhou et al., 2018). With the subsequent addition of the complex enzymes, the SDF chains were hydrolysed into low-molecular-weight fragments, some of which were easily lost during the filtration and washing processes, thus decreasing the DF content (Moczkowska et al., 2019).
Effects of enzymatic hydrolysis, ultrasonic treatment and enzymatic hydrolysis combined with ultrasonic modification on monosaccharide composition of tea seed fibre
Monosaccharide composition (mg g−1 fibre) . | PF . | EHF . | UTF . | EUF . |
---|---|---|---|---|
Guluronic acid | 9.22 ± 0.01c | 9.94 ± 0.04a | 9.57 ± 0.01b | 9.04 ± 0.01d |
Mannose | 9.04 ± 0.03b | 7.76 ± 0.02c | 10.50 ± 0.03a | 7.54 ± 0.01d |
Rhamnose | 10.97 ± 0.01c | 11.17 ± 0.03b | 12.28 ± 0.06a | 10.07 ± 0.04d |
Glucosamine | 6.61 ± 0.01c | 11.05 ± 0.06a | 8.72 ± 0.01b | 6.53 ± 0.01c |
Glucuronic acid | 3.66 ± 0.03c | 4.17 ± 0.04b | 4.35 ± 0.06a | 3.72 ± 0.04c |
Galacturonic acid | 45.97 ± 0.03d | 53.25 ± 0.01b | 62.66 ± 0.02a | 50.22 ± 0.06c |
Glucose | 18.88 ± 0.01b | 16.46 ± 0.05c | 19.42 ± 0.02a | 15.62 ± 0.07d |
Galactose | 71.97 ± 0.02c | 75.86 ± 0.03a | 72.94 ± 0.04b | 66.21 ± 0.05d |
Xylose | 47.92 ± 0.02b | 40.87 ± 0.01c | 51.01 ± 0.03a | 43.80 ± 0.02d |
Arabinose | 227.33 ± 0.02c | 261.12 ± 0.05a | 230.89 ± 0.05b | 219.51 ± 0.02d |
Fucose | 7.12 ± 0.01b | 6.67 ± 0.04c | 7.41 ± 0.02a | 5.85 ± 0.01d |
Total | 458.67 ± 0.11c | 498.30 ± 0.35a | 489.70 ± 0.27b | 438.09 ± 0.08d |
Monosaccharide composition (mg g−1 fibre) . | PF . | EHF . | UTF . | EUF . |
---|---|---|---|---|
Guluronic acid | 9.22 ± 0.01c | 9.94 ± 0.04a | 9.57 ± 0.01b | 9.04 ± 0.01d |
Mannose | 9.04 ± 0.03b | 7.76 ± 0.02c | 10.50 ± 0.03a | 7.54 ± 0.01d |
Rhamnose | 10.97 ± 0.01c | 11.17 ± 0.03b | 12.28 ± 0.06a | 10.07 ± 0.04d |
Glucosamine | 6.61 ± 0.01c | 11.05 ± 0.06a | 8.72 ± 0.01b | 6.53 ± 0.01c |
Glucuronic acid | 3.66 ± 0.03c | 4.17 ± 0.04b | 4.35 ± 0.06a | 3.72 ± 0.04c |
Galacturonic acid | 45.97 ± 0.03d | 53.25 ± 0.01b | 62.66 ± 0.02a | 50.22 ± 0.06c |
Glucose | 18.88 ± 0.01b | 16.46 ± 0.05c | 19.42 ± 0.02a | 15.62 ± 0.07d |
Galactose | 71.97 ± 0.02c | 75.86 ± 0.03a | 72.94 ± 0.04b | 66.21 ± 0.05d |
Xylose | 47.92 ± 0.02b | 40.87 ± 0.01c | 51.01 ± 0.03a | 43.80 ± 0.02d |
Arabinose | 227.33 ± 0.02c | 261.12 ± 0.05a | 230.89 ± 0.05b | 219.51 ± 0.02d |
Fucose | 7.12 ± 0.01b | 6.67 ± 0.04c | 7.41 ± 0.02a | 5.85 ± 0.01d |
Total | 458.67 ± 0.11c | 498.30 ± 0.35a | 489.70 ± 0.27b | 438.09 ± 0.08d |
EHF, enzymatically hydrolysed fibre; EUF, enzymatic-ultrasonic treated fibre; PF, purified fibre; UTF, ultrasonically treated fibre.
Data are expressed as the mean ± SD (n = 9). Different lowercase (a–d) in the same row means significant difference (P < 0.05).
Effects of enzymatic hydrolysis, ultrasonic treatment and enzymatic hydrolysis combined with ultrasonic modification on monosaccharide composition of tea seed fibre
Monosaccharide composition (mg g−1 fibre) . | PF . | EHF . | UTF . | EUF . |
---|---|---|---|---|
Guluronic acid | 9.22 ± 0.01c | 9.94 ± 0.04a | 9.57 ± 0.01b | 9.04 ± 0.01d |
Mannose | 9.04 ± 0.03b | 7.76 ± 0.02c | 10.50 ± 0.03a | 7.54 ± 0.01d |
Rhamnose | 10.97 ± 0.01c | 11.17 ± 0.03b | 12.28 ± 0.06a | 10.07 ± 0.04d |
Glucosamine | 6.61 ± 0.01c | 11.05 ± 0.06a | 8.72 ± 0.01b | 6.53 ± 0.01c |
Glucuronic acid | 3.66 ± 0.03c | 4.17 ± 0.04b | 4.35 ± 0.06a | 3.72 ± 0.04c |
Galacturonic acid | 45.97 ± 0.03d | 53.25 ± 0.01b | 62.66 ± 0.02a | 50.22 ± 0.06c |
Glucose | 18.88 ± 0.01b | 16.46 ± 0.05c | 19.42 ± 0.02a | 15.62 ± 0.07d |
Galactose | 71.97 ± 0.02c | 75.86 ± 0.03a | 72.94 ± 0.04b | 66.21 ± 0.05d |
Xylose | 47.92 ± 0.02b | 40.87 ± 0.01c | 51.01 ± 0.03a | 43.80 ± 0.02d |
Arabinose | 227.33 ± 0.02c | 261.12 ± 0.05a | 230.89 ± 0.05b | 219.51 ± 0.02d |
Fucose | 7.12 ± 0.01b | 6.67 ± 0.04c | 7.41 ± 0.02a | 5.85 ± 0.01d |
Total | 458.67 ± 0.11c | 498.30 ± 0.35a | 489.70 ± 0.27b | 438.09 ± 0.08d |
Monosaccharide composition (mg g−1 fibre) . | PF . | EHF . | UTF . | EUF . |
---|---|---|---|---|
Guluronic acid | 9.22 ± 0.01c | 9.94 ± 0.04a | 9.57 ± 0.01b | 9.04 ± 0.01d |
Mannose | 9.04 ± 0.03b | 7.76 ± 0.02c | 10.50 ± 0.03a | 7.54 ± 0.01d |
Rhamnose | 10.97 ± 0.01c | 11.17 ± 0.03b | 12.28 ± 0.06a | 10.07 ± 0.04d |
Glucosamine | 6.61 ± 0.01c | 11.05 ± 0.06a | 8.72 ± 0.01b | 6.53 ± 0.01c |
Glucuronic acid | 3.66 ± 0.03c | 4.17 ± 0.04b | 4.35 ± 0.06a | 3.72 ± 0.04c |
Galacturonic acid | 45.97 ± 0.03d | 53.25 ± 0.01b | 62.66 ± 0.02a | 50.22 ± 0.06c |
Glucose | 18.88 ± 0.01b | 16.46 ± 0.05c | 19.42 ± 0.02a | 15.62 ± 0.07d |
Galactose | 71.97 ± 0.02c | 75.86 ± 0.03a | 72.94 ± 0.04b | 66.21 ± 0.05d |
Xylose | 47.92 ± 0.02b | 40.87 ± 0.01c | 51.01 ± 0.03a | 43.80 ± 0.02d |
Arabinose | 227.33 ± 0.02c | 261.12 ± 0.05a | 230.89 ± 0.05b | 219.51 ± 0.02d |
Fucose | 7.12 ± 0.01b | 6.67 ± 0.04c | 7.41 ± 0.02a | 5.85 ± 0.01d |
Total | 458.67 ± 0.11c | 498.30 ± 0.35a | 489.70 ± 0.27b | 438.09 ± 0.08d |
EHF, enzymatically hydrolysed fibre; EUF, enzymatic-ultrasonic treated fibre; PF, purified fibre; UTF, ultrasonically treated fibre.
Data are expressed as the mean ± SD (n = 9). Different lowercase (a–d) in the same row means significant difference (P < 0.05).
WHC, OBC, and SC
The WHC, OBC and SC of DF are closely related to its functional characteristics, such as preventing water and fat loss from food and reducing the adsorption of fat during the human intestinal digestion process (Martínez et al., 2012). The WHC, OBC and SC values of UTF were 60.15 g g−1, 30.42 g g−1 and 29.93 mL g−1, respectively, which were significantly higher than those of PF, EHF and EUF ((P < 0.05, Table 2). Moreover, the WHC, OBC and SC of UTF were better than those of bagasse fibre (Sangnark & Noomhorm, 2003), cumin fibre (Ma & Mu, 2016), bamboo shoot fibre (Luo et al., 2018) and coconut fibre (Zheng & Li, 2018). The reason for this improvement may be that ultrasonic cavitation effect, which destroys the hydrogen bonds between cellulose and hemicellulose molecules and improves the efficiency of hydrated hydroxides, carboxyl groups and capillary action (Daou & Zhang, 2014). In addition, the reduction of the particle size of the fibre by the ultrasonic treatment probably increases the porosity of the surface structure of the fibre and increases the adsorption capacity of the fibre (Luo et al., 2018).
Effects of enzymatic hydrolysis and ultrasonic treatment on physicochemical properties of tea seed fibre
Physicochemical properties . | PF . | EHF . | UTF . | EUF . |
---|---|---|---|---|
WHC (g g−1) | 44.29 ± 3.28c | 59.34 ± 0.96a | 60.15 ± 2.52a | 51.89 ± 1.74b |
OBC (g g−1) | 18.37 ± 0.86c | 21.67 ± 0.64b | 30.42 ± 0.81a | 19.58 ± 0.6c |
SC (mL g−1) | 28.60 ± 0.67d | 29.47 ± 0.36b | 29.93 ± 1.16a | 29.07 ± 1.11c |
EAF (m2 g−1) | 267.97 ± 2.51c | 269.54 ± 2.3c | 381.36 ± 1.19a | 284.21 ± 1.4b |
ESF | 10.77 ± 0.03d | 12.11 ± 0.02b | 20.20 ± 0.01a | 11.78 ± 0.02c |
Physicochemical properties . | PF . | EHF . | UTF . | EUF . |
---|---|---|---|---|
WHC (g g−1) | 44.29 ± 3.28c | 59.34 ± 0.96a | 60.15 ± 2.52a | 51.89 ± 1.74b |
OBC (g g−1) | 18.37 ± 0.86c | 21.67 ± 0.64b | 30.42 ± 0.81a | 19.58 ± 0.6c |
SC (mL g−1) | 28.60 ± 0.67d | 29.47 ± 0.36b | 29.93 ± 1.16a | 29.07 ± 1.11c |
EAF (m2 g−1) | 267.97 ± 2.51c | 269.54 ± 2.3c | 381.36 ± 1.19a | 284.21 ± 1.4b |
ESF | 10.77 ± 0.03d | 12.11 ± 0.02b | 20.20 ± 0.01a | 11.78 ± 0.02c |
EAF, emulsifying activity of the fibre; EHF, enzymatically hydrolysed fibre; ESF, emulsifying stability of the fibre; EUF, enzymatic-ultrasonic treated fibre; OBC, oil-binding capacity; PF, purified fibre; SC, swelling capacity; UTF, ultrasonically treated fibre; WHC, water-holding capacity.
Data are expressed as the mean ± SD (n = 9). Different lowercase (a–d) in the same row means significant difference (P < 0.05).
Effects of enzymatic hydrolysis and ultrasonic treatment on physicochemical properties of tea seed fibre
Physicochemical properties . | PF . | EHF . | UTF . | EUF . |
---|---|---|---|---|
WHC (g g−1) | 44.29 ± 3.28c | 59.34 ± 0.96a | 60.15 ± 2.52a | 51.89 ± 1.74b |
OBC (g g−1) | 18.37 ± 0.86c | 21.67 ± 0.64b | 30.42 ± 0.81a | 19.58 ± 0.6c |
SC (mL g−1) | 28.60 ± 0.67d | 29.47 ± 0.36b | 29.93 ± 1.16a | 29.07 ± 1.11c |
EAF (m2 g−1) | 267.97 ± 2.51c | 269.54 ± 2.3c | 381.36 ± 1.19a | 284.21 ± 1.4b |
ESF | 10.77 ± 0.03d | 12.11 ± 0.02b | 20.20 ± 0.01a | 11.78 ± 0.02c |
Physicochemical properties . | PF . | EHF . | UTF . | EUF . |
---|---|---|---|---|
WHC (g g−1) | 44.29 ± 3.28c | 59.34 ± 0.96a | 60.15 ± 2.52a | 51.89 ± 1.74b |
OBC (g g−1) | 18.37 ± 0.86c | 21.67 ± 0.64b | 30.42 ± 0.81a | 19.58 ± 0.6c |
SC (mL g−1) | 28.60 ± 0.67d | 29.47 ± 0.36b | 29.93 ± 1.16a | 29.07 ± 1.11c |
EAF (m2 g−1) | 267.97 ± 2.51c | 269.54 ± 2.3c | 381.36 ± 1.19a | 284.21 ± 1.4b |
ESF | 10.77 ± 0.03d | 12.11 ± 0.02b | 20.20 ± 0.01a | 11.78 ± 0.02c |
EAF, emulsifying activity of the fibre; EHF, enzymatically hydrolysed fibre; ESF, emulsifying stability of the fibre; EUF, enzymatic-ultrasonic treated fibre; OBC, oil-binding capacity; PF, purified fibre; SC, swelling capacity; UTF, ultrasonically treated fibre; WHC, water-holding capacity.
Data are expressed as the mean ± SD (n = 9). Different lowercase (a–d) in the same row means significant difference (P < 0.05).
Emulsifying capacity and emulsion stability
The EAF and ESF values of UTF were 281.36 m2 g−1 and 20.20, respectively (Table 2), which are significantly higher than those of PF, EHF and EUF (P < 0.05). This difference may be caused by the higher oil adsorption capacity of the UTF. The fibre emulsification performance is related to the SDF content, but the emulsifying properties of the fibre are also affected by the water and oil retention characteristics of the fibre (Chen et al., 2015). The reduction of the fibre particle size by ultrasonic treatment probably also has an effect, as smaller fibres could rapidly diffuse to the oil–water interface and easily form a stable emulsion.
Microstructure characterisation
Microstructure and particle size analysis
SEM images of PF, EHF, UTF and EUF are shown in Fig. 1. The surface of unmodified PF was irregular and flaky, with a smooth and fluffy surface. It is likely that residual lipids, proteins and starch impurities were completely removed from the tea seed cake during the purification process (Chen et al., 2013). After the hydrolysis and modification of EHF by complex enzymes, defects were observed on the matrix surface and many internal structures were exposed. The irregular porous surfaces of UTF and EUF were caused by the ultrasonic treatment. The strong mechanical force and cavitation effect of the ultrasonic waves completely destroyed the sample matrix, leading to the degradation of some fibres and semi-fibres, and the formation of a discontinuous loose porous surface of the fibre (Wen et al., 2017). In addition, the fibres EUF and UTF were hydrolysed by complex enzymes and treated by ultrasound, resulting in a decrease in particle size and an increase of the matrix surface area, probably owing to the degradation capacity of some compounds in the matrix (Liu et al., 2016). Therefore, the microstructures of the samples shown in Fig. 1 confirm that complex enzyme modification and ultrasonic modification change the surface structure of the fibre, making it porous and increasing the adsorption capacity of the fibre.
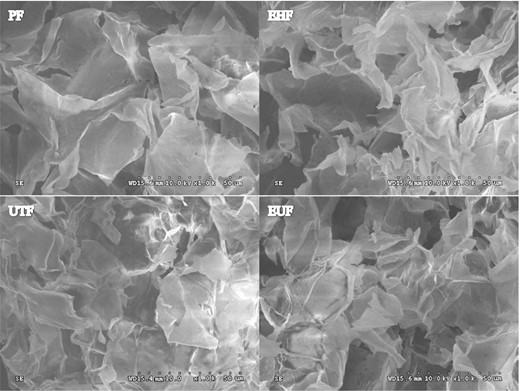
Scanning electron microscopy of tea seed fibre. PF, purified fibre; UTF, ultrasonic treated fibre; EHF, enzymatic hydrolysis fibre; EUF, enzymatic-ultrasonic fibre.
The particle size of the fibre was obviously reduced after modification (Figure S2). The particle sizes of PF, EHF, UTF and EUF were 84.7, 66.2, 39.63 and 61.16 µm, respectively (P < 0.05). The reduction in the particle size of the fibre should improve its ability to absorb glucose, cholesterol and nitrite ions in the gastrointestinal tract (Navarro-González et al., 2011). Complex enzyme modification and ultrasonic modification reduce the particle size of the fibre, thus increasing the porosity of the fibre surface as well as the adsorption capacity and emulsification stability of the fibre.
XRD analysis
The XRD patterns of all the samples showed a regular sharp peak at 21.6° and a weak peak at 15.9°, which are characteristic of cellulose crystals (Fig. 2a). There was no obvious difference in the peak positions for PF, EHF, UTF and EUF, indicating that the crystal structure of the fibre did not change after complex enzymatic hydrolysis modification or ultrasonic modification (Zhao et al., 2013). However, the crystallinity value of UTF (36.87%, Fig. 2b) was significantly higher than those of PF (24.56%), EUF (28.15%) and EHF (29.46%, P < 0.05). This difference may be due to the destruction of amorphous areas of the fibre and the removal of some lignin and hemicellulose during the modification process (Qi et al., 2016). Moreover, change is likely related to the fact that the extremely strong mechanical action and cavitation of ultrasonic treatment reduces the particle size of the fibre, leading to the some degradation of cellulose and hemicellulose.

The X-ray diffraction (a) and crystallinity (b) of tea seed fibre. Different lowercase (a–d) on the bar means significant difference (P < 0.05). EHF, enzymatic hydrolysis fibre; EUF, enzymatic-ultrasonic fibre; PF, purification of fibre; UTF, ultrasonic treated fibre.
FT-IR analysis
Although the FT-IR spectra of the four fibre samples show some similarities, there are obvious differences in the intensity and position of various characteristic absorption peaks (Fig. 3a). The peak at 3411.96 cm−1 corresponds to the stretching of hydroxyl groups, which may be caused by the hydrogen bonds of cellulose and hemicellulose (Cheikh Rouhou et al., 2018). The peak at 2926.15 cm−1 corresponds to a characteristic hydrocarbon vibration of cellulose. The peak near 1741.84 cm−1 is generally considered to be a typical bending or stretching vibration corresponding to various groups on lignin and hemicellulose (Kaushik & Singh, 2011). The peak at 1632 cm−1 is mainly characteristic of carboxyl group bending or stretching, which may be due to interactions between the carboxyl groups and cellulose or hemicellulose chain through intermolecular hydrogen bonds (Popescu et al., 2009). In addition, this peak is affected by the bending vibration of water absorbed by crystalline cellulose and hemicellulose (Cherian et al., 2008). The peak at 1061.02 cm−1 corresponds to the vibration, bending or stretching of the ether bond of lignin or hemicellulose (C–O–C). Overall, the FT-IR spectra reveal that the bonding in PF is obviously different to that in EHF, UTF and EUF bonds. The difference is related to the destruction of hydrogen bonds in cellulose, hemicellulose and lignin by complex enzymatic hydrolysis and ultrasonic treatment (Ma & Mu, 2016), and the formation of a new amorphous cellulose and soluble sugars.
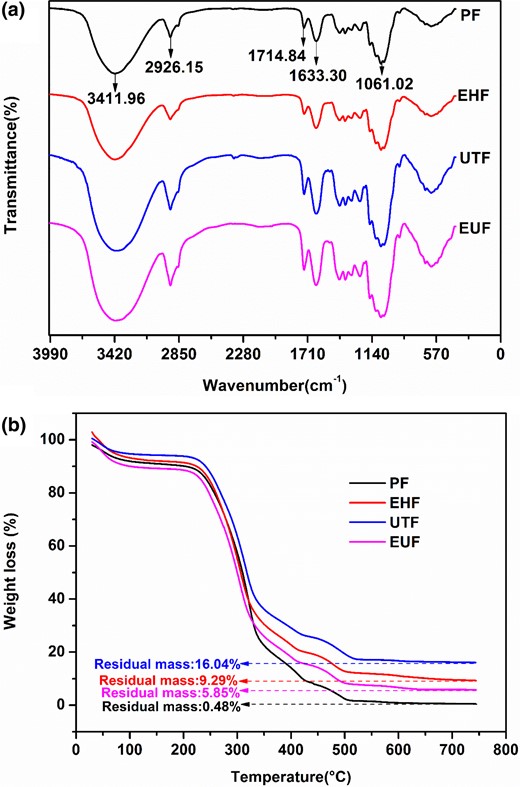
The Fourier-transformed infrared spectroscopy (a) and thermal properties by TGA (b) of tea seed fibre. EHF, enzymatic hydrolysis fibre; EUF, enzymatic-ultrasonic fibre; PF, purified fibre; UTF, ultrasonic treated fibre.
TGA
The TGA results show that UTF, EHF and EUF have higher thermal stabilities than PF (Fig. 3b). PF underwent initial thermal oxidation and decomposition at 304.54 °C, whereas the modified fibres EHF, UTF and EUF underwent initial thermal oxidation and decomposition at 321.8, 315.7 and 318.5 °C, respectively. UTF had a higher initial degradation temperature than PF, EHF and EUF. Meanwhile, the residual mass of UTF (16.04%) was higher than those of the PF (0.48%), EHF (9.29%) and EUF (5.85%). These results show that modification by ultrasonic treatment results in a fibre with good thermal stability, probably due to the higher crystallinity and degradation temperature of UTF.
In vitro gastrointestinal simulation
CAC of fibres
The CAC is one of the important indexes for evaluating the physiological characteristics of DF, and it has been proved that an adequate intake of DF can reduce the risk of digestive tract and cardiovascular diseases (Brouns et al., 2011). The CAC values of PF, EHF, UTF and EUF in the in vitro gastrointestinal tract (pH 2.0 and 7.0) are shown in Fig. 4a. The CAC values of PF, EHF, UTF and EUF are 8.76, 6.56, 12.71 and 7.61 mg g−1, respectively, in the gastric environment (pH 2.0) and 6.18, 5.94, 8.67 and 7.03 mg g−1, respectively, in the intestinal environment (pH 7.0). The CAC values in the gastric environment (pH 2.0) are higher than those in the intestinal environment (pH 7.0). Thus, the CAC of DF is affected by pH, with the adsorption of cholesterol mainly occurring in the stomach. Furthermore, the CAC of UTF is significantly higher than those of EHF, EUF and PF (P < 0.05). This increase in the CAC of UTF is mainly due to the cavitation effect of the ultrasonic wave and the mechanical crushing effect that destroyed the glycosidic bonds inside the fibre, resulting in a porous structure on the fibre surface (Wen et al., 2017). In addition, the ultrasonic modification significantly reduced the particle size of the fibre, which also affected the CAC (Liu et al., 2016). Complex enzyme modification also reduced the size of fibre, but the CAC of EHF was lower than those of PF, UTF and EUF under the in vitro gastrointestinal conditions (pH 2.0 and 7.0). EHF exhibited a poor CAC in the intestines and stomach, likely because enzymatic hydrolysis changed the structural characteristics of the fibre.

The physiological and functional properties of tea seed fibre in vitro. All values represent the mean ± SD (n = 9). EHF, enzymatic hydrolysis fibre; EUF enzymatic-ultrasonic fibre; PF, purified fibre; UTF, ultrasonic treated fibre; CAC (a), cholesterol adsorption capacity; GAC (b), glucose adsorption capacity; ACNI (c and d), adsorption capacity of nitrite ions
ACNI of fibres
As can be seen from Fig. 4c,d, the ACNI values of the four fibres in the simulated gastric environment (pH 2.0) were significantly higher than the ACNI values of the four fibres in the simulated intestinal environment (pH 7.0). Furthermore, the ACNI values of PF, EHF, UTF and EUF in the simulated gastric environment (pH 2.0) showed an upward trend from 60 to 360 min and then gradually from 360 to 480 min. This observation may indicate that the fibre's ability to adsorb nitrite ions reached saturation. In contrast, the ACNI values of the four fibres in the simulated intestinal environment (pH 7.0) showed an upward trend within the range of 60–480 min. Compared with PF, EHF and EUF, UTF showed a significant adsorption capacity for nitrite ions in the gastrointestinal environment (P < 0.05), and the optimal ACNI values of UTF in the gastrointestinal environment (pH 2.0 and 7.0) were 1.10 and 0.27 mg g−1, respectively. The good performance of UTF may be due to the porous structure and smaller particle size of this fibre (Zhu et al., 2015). These results differed from those in other studies on improving the adsorption capacity of fibres for nitrite by physical modification, which may be due to differences in the modification methods used and the action mechanisms (Luo et al., 2018).
GAC of fibres
The GAC values of the four fibres are shown in Fig. 4b. Within the investigated glucose concentration range of 0.1–1.0 (mmol L−1), PF, EHF, UTF and EUF exhibited effective adsorption of glucose. Each fibre exhibited a maximum GAC at a glucose concentration (0.5 mmol L−1), with GAC values of 56.75, 63.13, 72.89 and 61.03 µmol g−1 for PF, EHF, UTF and EUF, respectively (P < 0.05). The decrease of the GAC at higher glucose concentrations may be caused by the saturation of the fibre's ability to adsorb glucose. However, the GAC of UTF was significantly higher than those of PF, EHF and EUF, indicating that UTF exhibited a better in vitro gastrointestinal hypoglycaemic effect, which may be related to the porous surface structure of the modified fibre (Fig. 1). The porous structure of the UTF surface significantly enhances the amount of glucose molecules that can be embedded on the fibre network, thus enhancing the GAC (Peerajit et al., 2012).
Conclusions
In this study, tea seed DF was modified by complex enzymatic hydrolysis, ultrasonic treatment or and enzymatic-assisted ultrasonic treatment. Compared with PF, EHF and EUF, UTF exhibited better physicochemical and physiological properties with higher WHC, OBC, SC, EAF, ESF, CAC, ACNI and GAC. SEM images and particle size analysis confirmed that ultrasonic treatment caused obvious changes in the microstructure and particle size of the fibre. In addition, XRD, TGA and FT-IR spectroscopy showed that the crystallinity and thermal stability of UTF were improved significantly. In conclusion, ultrasound treatment not only increased the adsorption properties of tea seed DF, but also improved its physiological properties.
Acknowledgments
This work was supported by the Key Research and Development Program Project of Hainan province in 2019 (ZDYD201902), the Postgraduate Innovative Scientific Research Project of Hainan University in 2018 (hys2018-190), Hainan North Latitude 18° Food Processing Co., Ltd. (Hainan, China) and Hainan Dabai Kangjian Pharmaceutical Co., Ltd. (Hainan, China).
Conflict of interest
Authors do not declare any conflict of interest.
Ethical approval
Ethics approval was not required for this research.
Data availability statement
The data that support the findings of this study are available from the corresponding author upon request.
References
Author notes
These authors are contributed equally to the work.
The peer review history for this article is available at https://publons.com/publon/10.1111/ijfs.14465