-
PDF
- Split View
-
Views
-
Cite
Cite
Xue-Na Yu, Ye Guo, Qianling Yang, Haiyan Yu, Meng-Jiao Lu, Liang Zhao, Zhuo-Shuai Jin, Xiang-nan Xu, Jia-Yue Feng, Ying-Qiang Wen, Chimeric mutations in grapevine ENHANCED DISEASE RESISTANCE1 improve resistance to powdery mildew without growth penalty, Plant Physiology, Volume 195, Issue 3, July 2024, Pages 1995–2015, https://doi.org/10.1093/plphys/kiae169
- Share Icon Share
Abstract
Grapevine (Vitis vinifera L.) incurs severe quality degradation and yield loss from powdery mildew, a major fungal disease caused by Erysiphe necator. ENHANCED DISEASE RESISTANCE1 (EDR1), a Raf-like mitogen-activated protein kinase kinase kinase, negatively regulates defense responses against powdery mildew in Arabidopsis (Arabidopsis thaliana). However, little is known about the role of the putatively orthologous EDR1 gene in grapevine. In this study, we obtained grapevine VviEDR1-edited lines using CRISPR/Cas9. Plantlets containing homozygous and bi-allelic indels in VviEDR1 developed leaf lesions shortly after transplanting into the soil and died at the seedling stage. Transgenic plants expressing wild-type VviEDR1 and mutant Vviedr1 alleles as chimera (designated as VviEDR1-chi) developed normally and displayed enhanced resistance to powdery mildew. Interestingly, VviEDR1-chi plants maintained a spatiotemporally distinctive pattern of VviEDR1 mutagenesis: while almost no mutations were detected from terminal buds, ensuring normal function of the apical meristem, mutations occurred in young leaves and increased as leaves matured, resulting in resistance to powdery mildew. Further analysis showed that the resistance observed in VviEDR1-chi plants was associated with callose deposition, increased production of salicylic acid and ethylene, H2O2 production and accumulation, and host cell death. Surprisingly, no growth penalty was observed with VviEDR1-chi plants. Hence, this study demonstrated a role of VviEDR1 in the negative regulation of resistance to powdery mildew in grapevine and provided an avenue for engineering powdery mildew resistance in grapevine.
Introduction
Grapevine (Vitis sp.) is one of the oldest and most economically valuable fruit crops worldwide (Jaillon et al. 2007). Currently, most commercially cultivated grapevines originate from European grapevines (Vitis vinifera L.), which are highly susceptible to fungal diseases, especially powdery mildew caused by the fungus Erysiphe necator. Erysiphe necator not only infects green leaves, affecting photosynthesis, but also infects young fruit, resulting in fruit cracking, which induces secondary infection by other saprophytic molds, seriously affecting grapevine production safety (Gadoury et al. 2012).
An integrated approach to crop disease control requires proper cultivation regimes, pesticide application, and breeding of resistant varieties (van Esse et al. 2020). The development of resistant germplasms mainly relies on mining and inserting the resistance (R) genes into elite cultivars through genetic hybrid (Dangl and Jones 2001). However, resistance mediated by a single R gene lacks persistence because it is to be easily overcome by evolving pathogens (Dangl et al. 2013), and although increasing attention has focused on the pyramiding of several R genes, it is too time consuming (Xiao et al. 2019). Meanwhile, recessive mutations in host susceptibility (S) genes have also been used to confer resistance to plants (van Schie and Takken 2014). Resistance mediated by one S gene is more durable than resistance mediated by a single R gene (Wang et al. 2022b), and disrupting a single S gene is faster and simpler than aggregating multiple R genes to achieve broad-spectrum and long-lasting disease resistance in crops. A classic example is the S gene MILDEW RESISTANTCE LOCUS O (MLO) mutation in barley (Hordeum vulgare L), the inactivation of which provides broad and durable resistance against the powdery mildew pathogen Blumeria graminis f. sp. hordei. Notably, mlo-based resistance has been effectively used in European barley agriculture for approximately four decades (Piffanelli et al. 2004). To date, many S genes have been identified and utilized in various crops, including MLO, DOWNY MILDEW RESISTANCE6 (DMR6), SUGARS WILL EVENTUALLY BE EXPORTED TRANSPORTERS (SWEETs), ETHYLENE RESPONSIVE FACTOR922 (ERF922), PUCCINIA STRIIFORMIS-INDUCED PROTEIN KINASE1 (PsIPK1), ENHANCED DISEASE RESISTANCE1 (EDR1), and so on (Zhang et al. 2017; Oliva et al. 2019; Xu et al. 2019; Thomazella et al. 2021; Li et al. 2022; Zhou et al. 2022; Wang et al. 2022a).
However, most S genes have important physiological functions other than disease resistance, and their mutations negatively impact plant growth, greatly limiting their use in plant disease-resistance breeding. For example, the SA 3-HYDROXYLASE (S3H) functioned in the SA to 2,3-DHBA conversion, and its mutant is less susceptible to Pseudomonas syringae but displays accelerating senescence in Arabidopsis (A. thaliana) (Zhang et al. 2013); the Clade III SWEETs, such as SWEET11, SWEET13, and SWEET14, are susceptibility factors for bacterial blight in rice (Oryza sativa L.), but the ossweet11sweet14 mutant has lower yield and grain-filling rate (Fei et al. 2021); and the mlo-associated resistance is also accompanied by growth penalty and yield loss (Büschges et al. 1997; Consonni et al. 2006). Therefore, using the S genes to improve crop disease resistance whilst guaranteeing crop yield and quality is an emerging problem in disease-resistance breeding.
The advent of CRISPR gene editing offered a shortcut for precise crop-resistance breeding (Gao 2021). For example, the CRISPR/Cas9 achieved Tamlo-R32 locus mutation (a recently discovered mlo mutant) and produced four wheat (Triticum aestivum L.) varieties with broad-spectrum powdery mildew resistance, with no impact on growth or yield (Li et al. 2022); the cis-acting components of the SWEET11, SWEET13, and SWEET14 promoters, which are recognized by pathogen transcription activator-like effectors, were edited using CRISPR/Cas9 to generate broad-spectrum resistance through the loss of effector-triggered susceptibility in rice (Oliva et al. 2019; Xu et al. 2019). Therefore, editing the S gene using CRISPR is an efficient method for obtaining resistant varieties.
The edr1 was identified by an Arabidopsis mutant that displayed enhanced disease resistance to the fungus Golovinomyces cichoracearum, a causal agent of powdery mildew (Frye and Innes 1998). It has been reported that the enhanced resistance of edr1 depends on the MITOGEN-ACTIVATED PROTEIN KINASE (MAPK) cascade members MAPK KINASE KINASE5 (MAPKKK5), MAPK KINASE4/5 (MKK4/5), and MAPK3/6. EDR1 negatively regulates the MKK4/5-MAPK3/6 kinase cascade by physically associating with MKK4/5 and repressing KEG (an E3 ligase) phosphorylation to degrade MKK4/MKK5 via the ubiquitin-proteasome system (Zhao et al. 2014; Gao et al. 2021). In the edr1 mutant, activated MKK4/5 and MPK3/6 regulate upstream MAPKKK5 activation by phosphorylation and further enhance MAPK cascade activation and disease resistance via a positive feedback mechanism (Fernández-Milmanda 2023; Wang et al. 2023). EDR1 also interacts with the E3 ubiquitin ligase ARABIDOPSIS TOXICOS EN LEVADURA1 (ATL1) and negatively regulates its ability activity to suppress cell death (Serrano et al. 2014). Additionally, the edr1-associated resistance phenotype is dependent on the salicylic acid (SA) pathway (Tang et al. 2005). EDR1 respectively interacts with two typical resistance-related proteins, ENHANCED DISEASE SUSCEPTIBILITY1 (EDS1) and PEPTIDYLARGININE DEIMINASE4 (PAD4), to inhibit heterodimer formation between EDS1 and PAD4, thereby negatively regulating the defense response and inhibiting cell death (Neubauer et al. 2020). Meanwhile, EDR1 is recruited by ENHANCED DISEASE RESISTANCE4 (EDR4) to the fungal penetration site (Wu et al. 2015). Besides, edr1 phenotypes also require several plasma membrane-localized pattern recognition receptors (PRRs), such as RECEPTOR-LIKE PROTEIN53 (RLP53), BRASSINOSTEROID INSENSITIVE1-ASSOCIATED KINASE1 (BAK1), and SUPPRESSOR OF BAK1-INTERACTING RECEPTOR KINASE1 (SOBIR1) (Chen et al. 2022), indicating that the activation of pattern-triggered immunity (PTI) signaling is crucial for the enhanced resistance of atedr1 mutant.
EDR1 is highly conserved across plant species (Frye et al. 2001). In rice, OsEDR1 also negatively regulated the plant defense responses. The osedr1 showed enhanced resistance to the bacterial pathogen Xanthomonas oryzae pv. oryzae. (Xoo) and Xanthomonas oryzae pv. oryzicola (Shen et al. 2011; Ma et al. 2021). Simultaneous modification of the three homologs of wheat EDR1 using CRISPR/Cas9 enhanced the taedr1 resistance to powdery mildew, accompanied by the highly H2O2 accumulation and callose deposition near the fungi penetration site. In contrast to Arabidopsis, in which the powdery mildew resistance in edr1 mutant is substantially associated with cell death, no mildew-induced cell death has been observed in taedr1 plants (Zhang et al. 2017). The atedr1-mediated disease resistance was SA-dependent and JA/ethylene-independent (Dangl and Jones 2001; Frye et al. 2001); however, osedr1-mediated resistance was associated with activation of SA and JA signaling and suppression of ethylene signaling (Shen et al. 2011). These results suggest that the role of EDR1 in plant species may differ in terms of immune response.
Here, we edited the VviEDR1 of a powdery mildew susceptible grapevine “Thomson Seedless” by CRISPR/Cas9 and obtained VviEDR1-edited lines of various editing types. Both the homozygous and bi-allelic edited lines showed low lignification, dwarfism, and strong spontaneous hypersensitive responses. Transgenic plants expressing both wild-type VviEDR1 and mutant Vviedr1 alleles as chimera (designated as VviEDR1-chi) developed normally. The mature leaves of VviEDR1-chi lines showed strong resistance to powdery mildew, which was accompanied by rapid upregulation of resistance-related gene expression, H2O2 accumulation, callose deposition, and high SA and ethylene levels. This study demonstrated the role of VviEDR1 in the negative regulation of the grapevine resistance to powdery mildew and provided an avenue for engineering powdery mildew resistance in grapevine.
Results
The VviEDR1 expression was down-regulated after the powdery mildew inoculation
We identified a putative ortholog of the Arabidopsis EDR1 gene (AtEDR1) in grapevine, VviEDR1, using multiple sequence alignment and evolutionary tree analysis (Fig. 1A). The VviEDR1 open reading frame was 2,955 bp and encoded a putative protein consisting of 984 amino acids, including two conserved domains, the EDR1 domain and the STKc-MAP3K-like domain in its protein sequence alignment (Fig. 1B). Then, we analyzed the expression patterns of VviEDR1 in the susceptible grapevine “Thompson Seedless” after the infection of powdery mildew. The transcription of VviEDR1 in grapevine leaves was continually downregulated after powdery mildew inoculation, reaching its lowest level at 5 dpi (Fig. 1C). These results indicate that VviEDR1 involved in the response of grapevine leaves to powdery mildew infection.
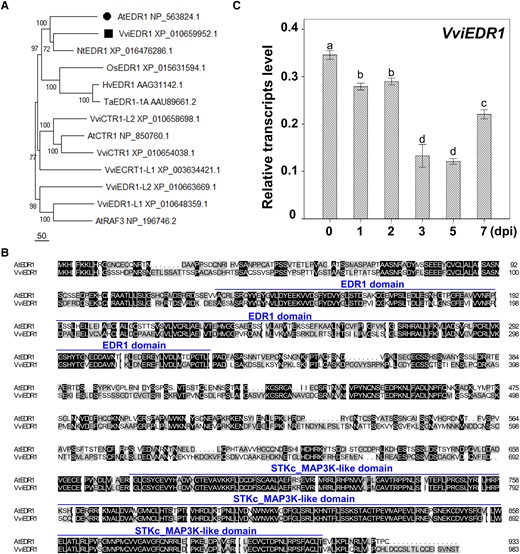
The expression patterns of VviEDR1 post En NAFU1 inoculation. A) Phylogenetic analysis of EDR1 obtained from grapevine (V. vinifera, Vvi), rice (Oryza sativa, Os), tobacco (Nicotiana tabacum, Nt), barley (Hordeum vulgare, Hv), wheat (Triticum aestivum, Ta), and Arabidopsis (Arabidopsis thaliana, At); B) Multiple alignments of amino acid sequences were obtained from AtEDR1 and the putative ortholog VviEDR1; C) Relative transcript levels of VviEDR1 in “Thomson Seedless” mature leavesat 1, 2, 3, 5, 7 post Erysiphe necator NAFU1 inoculation (dpi). Each data point represents the mean ± SD of triple biological replicates. Different letters above the bars indicate the significant differences (P < 0.05), determined by Student's t test.
The selection of sgRNA to targeted VviEDR1 and the obtainment of VviEDR1-edited lines
Three potentially independent sgRNAs were designed to target different sites on the VviEDR1 exon (Fig. 2A and Supplementary Fig. S2A). To quickly verify the mutagenic activity of these sgRNAs, plasmids containing sgRNA1, sgRNA2 or sgRNA2&3 were transformed into grapevine mesophyll protoplasts for transient expression (Supplementary Fig. S2B). After two rounds of PCR/restriction enzyme assay, the mutation fragments not digested by the corresponding restriction enzyme were detected in the protoplasts transformed with sgRNA1, sgRNA2, or sgRNA2&3 (Supplementary Fig. S2, C and D), indicating that the corresponding restriction enzyme site in the target loci of VviEDR1-T1 or T2 was already destroyed by CRISPR/Cas9. The mutated fragments were sequenced, and three and six different mutations were identified in VviEDR1-T1 and VviEDR1-T2, respectively (Supplementary Fig. S2, E and F). Moreover, a large fragment (162 bp) deletion between the T2 and T3 sites was detected in VviEDR1-T2&T3 transgenic protoplasts (Supplementary Fig. S2G). These results proved that the three sgRNAs could recognize their corresponding target sites and guide the Cas9 protein to induce genome editing.
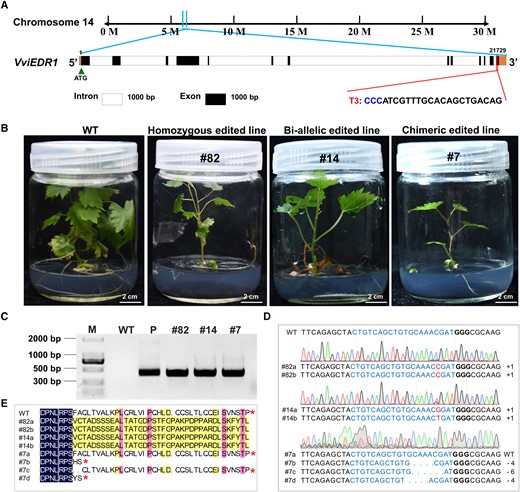
VviEDR1-T3 mutation types detected in the transgenic lines via CRISPR/Cas9-mediated editing. A) The nucleotide positions of VviEDR1-T3 on the chromosome; B) The phenotypes of VviEDR1-T3 edited lines, the images are the same as those in Supplementary Figure S4A; C) Identification of sgRNA sequences in the regenerated plants of VviEDR1-T3, and the amplification in positive lines was expected to give a product of 424 bp, whilst the CRISPR/Cas9 plasmid DNA and the wild type (WT) DNA were used as the positive and negative control, respectively; M, Marker; D) Sequencing chromatograms of the plants with different mutation types inVviEDR1-T3, and the PAM sequences were highlighted in bold, whilst the insertion sites were marked with red, and the deleted bases were indicated by “-”; E) Amino acid sequence alignment between the representative mutant and the WT for the target region of VviEDR1-T3.
Next, the CRISPR/Cas9 vectors containing sgRNA1, sgRNA2, sgRNA3, and sgRNA2&3 were transferred into the PEMs of “Thompson Seedless”: by Agrobacterium tumefaciens-mediated transformation. The process of transforming grapevine PEMs into complete plants is illustrated in Supplementary Fig. S3. In total, 1,546 regenerated plants were obtained. As shown in Table 1, 30 and 1,508 regenerated plants were obtained as targeting for VviEDR1-T2 and VviEDR1-T3, respectively, and eight regenerated plants were obtained as targeting for VviEDR1-T2&T3. Unfortunately, no regenerated plants targeting VviEDR1-T1 have been obtained. Mutations were detected in the regenerated plants. Six of the 30 regenerated plants from the targeting for VviEDR1-T2 transformed stably and were edited; among these six lines, two lines were homozygous for a single-base T insertion at 3 bp upstream of the PAM, and four lines had bi-allelic mutations with both alleles mutated, but the mutations were not identical (Supplementary Fig. S4, C and D). In the targeting for VviEDR1-T3 plantlets, only 54 of the 1,508 regenerated plants were transgenic (Table 1). Among these 54 lines, 46 were edited, 12 (26%) were homozygous for a 1-bp insertion, 29 (63%) were bi-allelic for a 1-bp insertion, and 5 (11%) were chimeric (i.e. more than two mutated alleles were detected in one line) (Fig. 2, C and D). Then, the DNA fragments covering the region of target sites of chimeric lines were cloned into pMD19-T for sequence analysis. Twenty independent clones were analyzed in the VviEDR1-chi line #6, and nine clones were differentially mutated sequences, whereas 11 clones were WT sequences. Twelve independent clones were analyzed in VviEDR1-chi line #7, and four clones were mutated sequences. All mutations in VviEDR1-T2 and -T3 indicated a frameshift mutation or a premature stop codon when the amino acid sequence was deduced from the mutated DNA sequence (Fig. 2E and Supplementary Fig. S4E). Unfortunately, no edited lines were obtained from the eight regenerated plants of VviEDR1-T2&T3. We also detected some potential off-target events caused by the CRISPR/Cas9 system, but no mutations occurred at potential off-target sites in all VviEDR1-edited lines (Supplementary Table S1).
Type . | VviEDR1-T1 . | VviEDR1-T2 . | VviEDR1-T3 . | VviEDR1-T2&T3 . |
---|---|---|---|---|
Regenerated lines | 0 | 30 | 1,508 | 8 |
CRISPR positive lines | 0 | 6 | 54 | 0 |
Homozygousa edited lines | 0 | 2 | 12 | 0 |
Bi-allelicb edited lines | 0 | 4 | 29 | 0 |
Chimericc edited lines | 0 | 0 | 5 | 0 |
Nonedited lines | 0 | 24 | 1,462 | 8 |
Editing efficiency | - | 100% | 85.19% | - |
Type . | VviEDR1-T1 . | VviEDR1-T2 . | VviEDR1-T3 . | VviEDR1-T2&T3 . |
---|---|---|---|---|
Regenerated lines | 0 | 30 | 1,508 | 8 |
CRISPR positive lines | 0 | 6 | 54 | 0 |
Homozygousa edited lines | 0 | 2 | 12 | 0 |
Bi-allelicb edited lines | 0 | 4 | 29 | 0 |
Chimericc edited lines | 0 | 0 | 5 | 0 |
Nonedited lines | 0 | 24 | 1,462 | 8 |
Editing efficiency | - | 100% | 85.19% | - |
aHomozygous = both alleles contain the same mutation.
bBi-allelic = both alleles are mutated, but the mutations are not identical.
cChimeric = presence of more than two mutant alleles, indicative of the presence of different edited cell lines.
Type . | VviEDR1-T1 . | VviEDR1-T2 . | VviEDR1-T3 . | VviEDR1-T2&T3 . |
---|---|---|---|---|
Regenerated lines | 0 | 30 | 1,508 | 8 |
CRISPR positive lines | 0 | 6 | 54 | 0 |
Homozygousa edited lines | 0 | 2 | 12 | 0 |
Bi-allelicb edited lines | 0 | 4 | 29 | 0 |
Chimericc edited lines | 0 | 0 | 5 | 0 |
Nonedited lines | 0 | 24 | 1,462 | 8 |
Editing efficiency | - | 100% | 85.19% | - |
Type . | VviEDR1-T1 . | VviEDR1-T2 . | VviEDR1-T3 . | VviEDR1-T2&T3 . |
---|---|---|---|---|
Regenerated lines | 0 | 30 | 1,508 | 8 |
CRISPR positive lines | 0 | 6 | 54 | 0 |
Homozygousa edited lines | 0 | 2 | 12 | 0 |
Bi-allelicb edited lines | 0 | 4 | 29 | 0 |
Chimericc edited lines | 0 | 0 | 5 | 0 |
Nonedited lines | 0 | 24 | 1,462 | 8 |
Editing efficiency | - | 100% | 85.19% | - |
aHomozygous = both alleles contain the same mutation.
bBi-allelic = both alleles are mutated, but the mutations are not identical.
cChimeric = presence of more than two mutant alleles, indicative of the presence of different edited cell lines.
Phenotypic characterization of VviEDR1-edited lines
The vast majority of edited lines in this study showed abnormal growth and development. When grown under axenic conditions, the VviEDR1-T2 edited lines had slower growth, less lignified stems, and a harder rooting process than the WT (Supplementary Fig. S4A). These plantlets gradually withered and died after growing on the rooting medium for approximately 40 d. The VviEDR1-T3 homozygous and bi-allelic edited plantlets were stronger than the VviEDR1-T2 edited plantlets but still weaker than the WT, and spontaneous necrotic lesions were observed in the leaves of VviEDR1-T3 bi-allelic-edited lines in the culture vessel (Fig. 2B and Supplementary Fig. S5). The VviEDR1-T3 edited lines were transplanted into sterile nutritive soil and cultured in a chamber. After 20 d of growth, the leaves of the homozygous and bi-allelic edited lines gradually withered, and their stems produced massive spontaneous necrotic lesions, which eventually led to death with whole-plant wilting (Fig. 3). Only VviEDR1-chi lines grew rapidly after transplantation, and no substantial difference was found compared to the WT (Fig. 3). Notably, there were no visible necrotic spots on the leaves at the initial stage of growth, but patches of cell death appeared on mature leaves that were not infected after growing in the chamber for 3 months (Supplementary Fig. S6).
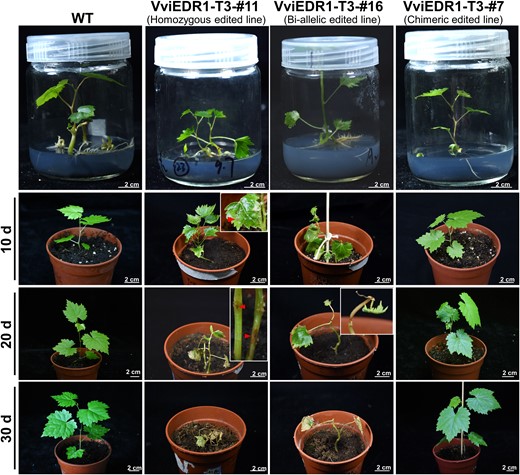
The phenotypes of the different types of VviEDR1-T3 edited lines after transplanting. The VviEDR1-T3 homozygous and bi-allelic edited lines developed visible spontaneous lesions (red arrow indicates) in the stems and leaves after transplanting to the pots; whilst wild type (WT) and VviEDR1-T3 chimeric edited lines grew normally, the image of line #7 in culture vessels is the same as that in Fig. 2B.
VviEDR1-edited enhanced powdery mildew resistance in grapevine
Previous studies have shown that AtEDR1 negatively regulates the resistance of Arabidopsis to powdery mildew (Frye and Innes 1998). To verify the role of VviEDR1 in the grapevine response to powdery mildew, detached leaves of VviEDR1-T3 bi-allelic edited lines #26 and #58, which contained a single base T/G insertion at the 3 bp upstream of PAM and owned a frameshift mutation in VviEDR1, were inoculated with a pure isolate of grapevine powdery mildew En NAFU1 (Supplementary Fig. S7A). The En NAFU1 germinated normally on the leaves of WT and VviEDR1-T3 bi-allelic indels mutant lines at 1 dpi (Supplementary Fig. S7B). A large number of hyphae were observed on the upper epidermis of the leaves of the WT at 3 dpi, and the primary hyphae grew rapidly and produced secondary hyphae of a certain length (Supplementary Fig. S7B). However, the mycelial growth of En NAFU1 was slow, and the average hyphal length per colony decreased by 44% to 61% on the leaves of lines #26 and #58 compared with that on the WT at 3 dpi (Supplementary Fig. S7C). The hyphae of powdery mildew grew vigorously, producing many secondary hyphae on the leaves of WT at 5 dpi, whereas the growth of powdery mildew hyphae was inhibited, and the secondary hyphae were fewer and shorter on the leaves of bi-allelic edited lines. At 7 dpi, fewer powdery mildew haustoria in the leaves of VviEDR1 bi-allelic edited lines, the majority of haustoria grew abnormally, with hypersensitive response-like cell death in the infected epidermal cells (Supplementary Fig. S7, B and D). These results indicated that the VviEDR1-T3 mutation enhanced the resistance of grapevines to powdery mildew at the initial stage of infection by inhibiting hyphal elongation and haustoria formation but not spore germination.
To explore the response of VviEDR1-chi leaves to powdery mildew infection, VviEDR1-chi lines were transplanted into pots and cultivated in a growth chamber. The mature leaves of the rooted plants were inoculated with En NAFU1, and both the WT and VviEDR1-chi lines showed powdery mildew infection symptoms at 14 dpi. However, the leaves of the WT were completely covered by powdery mildew, whereas the leaves of VviEDR1-chi lines #6 and #7 had no obvious whitish mildew colonies, and there were obvious necrotic spots on the leaves under barely visible hyphae, indicating that the leaves had produced a hypersensitive response (Fig. 4A). Trypan blue staining revealed that En NAFU1 grew vigorously, produced a large number of hyphae and conidia on the leaves of WT, and only rare or occasional hypersensitive response-like cell death was observed, whereas slower fungal growth and fewer conidia production were observed on the leaves of VviEDR1-chi lines compared to the WT (Fig. 4, B, E and F). In addition, extensive mildew-induced hypersensitivity responses in the infected epidermal cells and neighboring cells of the mature leaves of VviEDR1-chi lines were observed (Fig. 4B). DAB staining revealed greater H2O2 accumulation in the leaves of VviEDR1-chi lines than that in WT leaves (Fig. 4C). Aniline blue staining showed that more callose was deposited in the epidermal cells of VviEDR1-chi lines attacked by En NAFU1 than in the WT (Fig. 4D). Moreover, we quantified H2O2 and callose accumulation in leaves of WT and VviEDR1-chi lines, and the latter exhibited higher levels of these defense-related metabolites (Fig. 4, G and H).
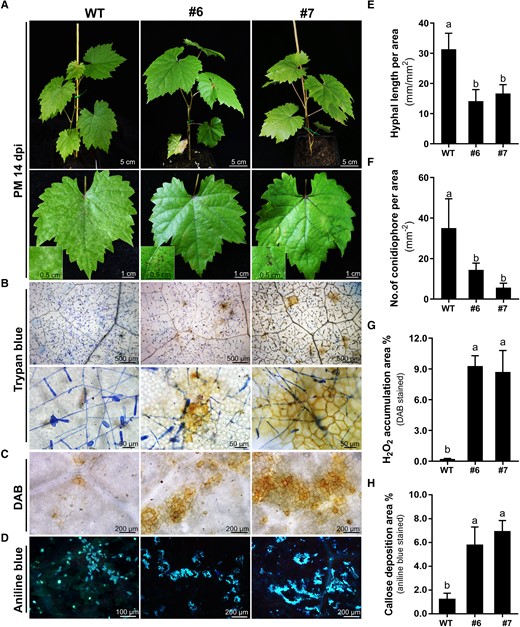
The VviEDR1-T3 chimeric edited lines showed infection-triggered cell death, H2O2 accumulation and callose deposition. A) The wild type (WT) and VviEDR1-chi lines have grown in chamber for 3 months and the leaves showed symptoms at 14 days post-En NAFU1 inoculation (dpi), and the insets showed higher magnification image of powdery mildew (PM) colonies and necrotic lesions on the leaves under the barely visible hypha; B) Representative images showed the trypan blue-stained leaf section of WT and VviEDR1-chi lines #6 and #7 at 14 dpi; C) DAB-stained epidermal cell of the WT and VviEDR1-chi lines at 14 dpi, DAB: diaminobenzidine; D) Representative micrographs showing aniline blue-stained epidermal cells of the WT and VviEDR1-chi lines at 14 dpi; E)–G) Quantification of hyphal length (E), number of conidiophores (F), H2O2 accumulation (G), and callose deposition area (H) on the leaves of chimeric edited lines at 14 dpi. Each data point of all graphs represents the mean ± SD of triple biological replicates. Different letters above the bars indicate the significant differences (P < 0.05), determined by Student's t test.
To investigate whether this resistance could be inherited by clonal propagation, line #7 was propagated by cutting and was grown in a chamber. The mature leaves of the rooting clones were inoculated with En NAFU1 (Supplementary Fig. S8A). When the leaves of the WT were completely covered by powdery mildew, the leaves of line #7-clone 1 and 2 had no obvious whitish mildew colonies at 22 dpi. Similar to the maternal plant, the leaves of line #7 clones showed extensive mildew-induced hypersensitive response-like cell death in the infected epidermal cells and neighboring cells, exhibiting strong resistance to powdery mildew (Supplementary Fig. S8B), indicating that this resistance could be inherited through vegetative propagation. Furthermore, when VviEDR1-chi lines and WT were grown in a greenhouse full of En NAFU1 for more than 2 years, VviEDR1-chi lines grew as vigorously as WT. In every natural outbreak of powdery mildew during these 2 years, most of the leaves of the WT plants were covered with abundant white velvety mycelia; however, no obvious whitish mildew colonies on the leaves of lines #6 and #7 (Fig. 5B and Supplementary Fig. S9).
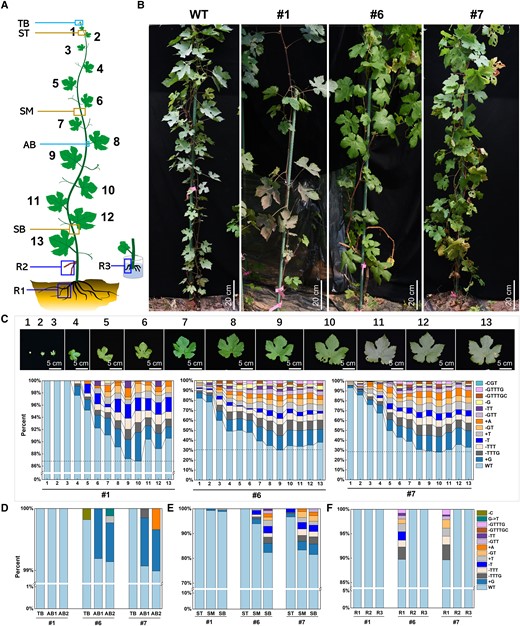
The VviEDR1 mutation types and mutation ratios in different tissues and organs of VviEDR1-chi lines. A) Schematic diagram of sampling sites for the determination of VviEDR1-T3 mutation types and mutation rates by next-generation sequencing, TB, terminal bud; AB, axillary bud; ST, stem-top; stem internode between leaf nos. 2 and nos. 3; SM, stem-middle, stem internode between leaf nos. 6 and nos. 7; SB, stem-bottom, stem internode between leaf nos. 12 and nos. 13; R1, lateral roots of 2-years seedling; R2, aerial roots produced at the base of the stem; R3, adventitious roots produced by stem cutting; B) The wild type (WT) and three VviEDR1-chi lines grown in greenhouse for 2 years; C) The proportions and types of mutated DNA in leaves of VviEDR1-chi lines #1, #6, and #7, and the leaf nos. 1–13 indicated the leaf position from the top bud to the bottom, referring to (A); D) The proportions and types of mutated DNA in the bud of VviEDR1-chi, AB1: the mix of axillary bud of leaf nos. 1–6, AB2: the mix of axillary bud of leaf nos. 7–13; E) The proportions and types of mutated DNA in the stem of VviEDR1-chi lines; F) The proportions and types of mutated DNA in the root of VviEDR1-chi lines.
The regulation of VviEDR1 mutation and resistance in VviEDR1-chi lines by CRISPR/Cas9
To investigate why VviEDR1-chi lines improved the resistance to powdery mildew without affecting the plant growth and development, we analyzed the types and proportions of VviEDR1 edited in the leaves and other tissues of VviEDR1-chi lines #1, #6, and #7 using NGS and Hi-TOM platform, and at least 10,000 reads were analyzed for each sample. As shown in Fig. 5C, the mutation ratios in the lower leaves, which appeared at an earlier stage of plant development, tended to be higher than those in the upper leaves. No mutated VviEDR1 was detected in the VviEDR1-chi line #1 leaf nos.1-3. As the leaf position decreased, the mutation ratio of VviEDR1 increased, and the mutation types were continuously enriched. Below about the leaf nos. 7, the mutation ratio of VviEDR1 tended to be stable between 8.95% and 13.22%. The regulation of VviEDR1 mutations in the leaves of VviEDR1-chi line s#6 and #7 was the same as that in VviEDR1-chi line #1, but the degree of mutation was substantially higher than that in the corresponding leaves of VviEDR1-chi line #1. The proportion of mutated VviEDR1 in the VviEDR1-chi line #6 leaves (Fig. 5A) from upper to lower (leaf nos. 1–13, accordingly), was as follows: 17.92% (nos. 1), 21.66% (nos. 2), 40.27% (nos. 3), 50.99% (nos. 4), 50.11% (nos. 5), 52.30% (nos. 6), 60.91% (nos. 7), 65.53% (nos. 8), 69.88% (nos. 9), 65.61% (nos. 10), 66.75% (nos. 11), 64.96% (nos. 12), and 62.10% (nos. 13). The number of mutation types increased from 9 to 13, and the mutation sites were mainly located at 3 to 5 bp upstream of the PAM sequence, producing single- or several-base indels. Similar to VviEDR1-chi line #6, the proportion of mutated DNA rapidly increased from 6.97% to 61.30% following leaf position nos. 1 to 7 and became stable below the leaf nos. 7 in VviEDR1-chi line #7. Additionally, mutations in the VviEDR1-chi line #7 clones were detected. In the newly unfolded leaves (leaf nos. 1), the ratios of mutated VviEDR1 were lower than 20%, and along with leaf growth, the ratios of mutated VviEDR1 increased, which was approximately 30% in leaf nos. 3, 50% in leaf nos. 5, and 55% in leaf nos. 7 of all three clones of VviEDR1-chi line #7 (Supplementary Fig. S10). The consistent mutation regulation and relatively stable mutation ratio among the different clones indicated that the chimeric trait of VviEDR1-chi could be inherited by clonal propagation.
Surprisingly, there were few mutations in the terminal buds of each VviEDR1-chi line, and the proportion of mutated VviEDR1 was <0.18%. In addition, both the upper axillary buds of leaf nos. 1 to 6 and lower axillary buds of leaf nos. 7 to 13 had a very low mutation ratio of less than 1.01%, indicating that VviEDR1 mutations were absent or rare in the shoot apical meristem (SAM) by CRISPR/Cas9 (Fig. 5D). Similar to the leaves, the proportions of mutated DNA in stem-bottom (17.68% in VviEDR1-chi line #6 and 27.96% in VviEDR1-chi line #7) were higher than those in stem-middle (6.06% in VviEDR1-chi line #6 and 16.7% in VviEDR1-chi line #7) and stem-top (NA in VviEDR1-chi #6 and 3.34% in VviEDR1-chi #7) of VviEDR1-chi lines. The mutation proportion of VviEDR1 in the stem was substantially lower than that in the leaf growing at the same node (Fig. 5E). In addition, approximately 10% of the mutated VviEDR1 contained nine mutation types occurring in the lateral roots of VviEDR1-chi lines #6 and #7 after 2 years of greenhouse growth. Aerial roots produced at the stem base and adventitious roots produced by stem cuttings were also sampled; however, no mutations were detected in all of the VviEDR1-chi lines (Fig. 5F). These results indicated that the ratio of mutated cells was higher in the lower positioned and older senescent tissues than in the newly developing tissue from the apical meristem.
To further confirm whether CRISPR/Cas9 worked continuously during leaf growth and contributed to mutation accumulation as the leaves matured, we tracked changes in the proportion of VviEDR1 mutations during VviEDR1-chi lines leaves growth. As shown in Fig. 6, A and B, when the leaves were just unfolded, the proportions of VviEDR1 mutations were 0, 22.93% and 17.66% in VviEDR1-chi lines #1, #6, and #7, respectively; the mutation ratio increased sharply with the leaves growing that the proportion of VviEDR1 mutations increased to 8.08%, 61.36%, and 67.58% in the leaves of VviEDR1-chi lines #1, #6, and #7, respectively, after 20 d of growth. Because the proportions of VviEDR1 mutations were low in the young leaves and high (>60%) in the mature leaves of VviEDR1-chi lines #6 and #7, we evaluated the powdery mildew resistance of both young (leaf nos. 5) and mature leaves (leaf nos.10) by natural infection in the greenhouse. As shown in Fig. 6C, whitish mildew colonies gleamed on the young leaves of WT and VviEDR1-chi lines. Trypan blue staining showed that the powdery mildew grew normally, producing a large number of hyphae and plump globose haustoria on young leaves of both the WT and VviEDR1-chi lines. The mature leaves of WT and VviEDR1-chi line #1 (the proportion of mutation was approximately 10%) were completely covered by powdery mildew, and most haustoria of powdery mildew were normal, indicating sensitivity to powdery mildew. The mature leaves of VviEDR1-chi lines #6 and #7 (the proportion of mutations was approximately 65%) showed strong resistance to powdery mildew, no obvious whitish mildew colonies and approximately 50% of the haustoria were abnormal (Fig. 6C), which could be attributed to the hypersensitive response of the infected epidermal cells and neighboring cells, which finally arrested fungal development (Fig. 6D).
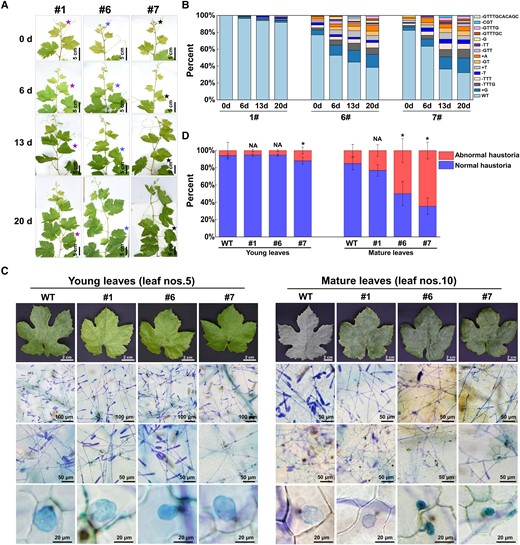
The proportions and types of mutated VviEDR1 increased with the leaves development, resulting in strong powdery mildew resistance in the mature leaves of VviEDR1-chi lines. A) The leaf development process of VviEDR1-chi lines, the stars indicated the morphology of the same leaf at the 0th, 6th, 13th, and 20th day of recording; B) The proportions and types of mutated VviEDR1 changed with the leaves development of VviEDR1-chi lines; C) Representative images showed the trypan blue-stained of young (leaf nos. 5) and mature (leaf nos. 10) leaves of WT and VviEDR1-chi lines, which were infected by the powdery mildew naturally occurred in greenhouse, the yellow arrow points to the normal haustoria of powdery mildew, and the red arrow points to the abnormal haustoria; D) The proportion of normal haustoria and abnormal haustoria in the leaves of WT and VviEDR1-chi lines. Each data point represents the mean ± SD of five biological replicates, asterisks indicate significant differences in comparison with the WT as determined by Student's t-test (*P < 0.05), NA indicate no significant differences (P > 0.05) in comparison with the WT.
VviEDR1 mutation activated multiple defense signaling pathways in the grapevine
To further investigate the mechanism by VviEDR1-mutation confers resistance to powdery mildew, transcriptomic analysis of the mature leaves of WT and VviEDR1-chi lines (#6 and #7) at 2 and 6 dpi was performed, and uninfected leaves were used as a mock. We also detected the expression levels of eight genes by RT-qPCR to validate the RNA-seq data (Supplementary Fig. S11). To identify the genes regulated by EDR1 under pathogen-free conditions, we first selected the common genes that were up- or downregulated more than two-fold in the VviEDR1-chi lines mock relative to the WT mock at 2 and 6 dpi, and the genes only differentially expressed at 2 or 6 dpi were eliminated to exclude the circadian effects on gene expression. There were 602 upregulated and 411 downregulated genes in VviEDR1-chi lines at all time points compared to the WT under pathogen-free conditions (Supplementary Fig. S12, A and B, Supplementary Table S2). The biological functions of these differentially expressed genes (DEGs) were annotated with gene ontology (GO) categories (Supplementary Fig. S12D). There were 584 out of 1,013 annotated genes categorized into multiple functional groups that belong to the biological process, and among these 584 functional genes, 126 genes participated in the protein phosphorylation (GO:0006468), 74 genes were involved in the defense response (GO:0006952), 28 genes worked in cell recognition (GO:0008037), and 16 genes regulated the cellular lipid metabolic process (GO:0044255). The 674 annotated DEGs were categorized according to molecular functions, including 126 genes controlled the protein kinase activity (GO:0004672), 99 genes controlled the oxidoreductase activity (GO:0016491), 94 genes controlled the hydrolase activity (GO:0016787), and 21 genes controlled the signaling receptor activity (GO:0038023). To further identify the functions of VviEDR1-chi lines DEGs under pathogen-free conditions, we mapped the annotated sequences to the reference canonical pathways in the Kyoto Encyclopedia of Genes and Genomes (KEGG) (Supplementary Fig. S12C). These regulated genes in the KEGG pathway were mainly involved in plant hormone signal transduction, the MAPK signaling pathway, and plant–pathogen interactions. Together, these results suggest that protein phosphorylation, defense response, oxidoreductase activity, and hormone signal transduction are altered in VviEDR1-chi lines without pathogen infection.
To identify the genes negatively regulated by EDR1 during powdery mildew infection, genes that were upregulated more than 2-fold in VviEDR1-chi lines or WT compared to uninoculated leaves were selected at 2 or 6 dpi as the gene subsets induced by En NAFU1. Genes with a 1.5-fold higher expression in VviEDR1-chi lines than in WT and in the gene subset induced by En NAFU1 of either VviEDR1-chi lines or WT were selected at 2 dpi or 6 dpi (areas bounded by the red oval in Fig. 7, A and B; Supplementary Table S3). In total, there were 860 and 705 genes at 2 and 6 dpi, respectively, in the VviEDR1-chi lines and powdery mildew-upregulated gene subsets. All genes that were upregulated in the VviEDR1-chi lines and powdery mildew at 2 and 6 dpi were referred to as the VviEDR1-chi&pm-upregulated gene set, and the total number of genes was 1,209 (Fig. 7C). These genes were mainly involved in the biological processes of cell recognition, cell surface receptor signaling pathway, defense response to other organisms, calcium ion transmembrane transport, glutathione metabolic process, and hormone-mediated signaling pathway. They mainly had biological functions as signaling receptor activity, ligand-gated ion channel activity, UDP-glycosyltransferase activity, glutathione transferase activity, and NAD(P)H dehydrogenase activity (Fig. 7D).

VviEDR1 regulates powdery mildew-responsive genes in grapevine. The Venn diagrams showing the overlap between the powdery mildew-induced and the VviEDR1-chi-induced gene sets at 2 dpi (A) and 6 dpi (B), and the red oval indicates the set of VviEDR1-chi&pm-upregulated genes used for the majority of the analyses; C) Venn diagrams showing the VviEDR1-chi&pm-upregulated genes set at 2 and 6 dpi; D) Gene Ontology (GO) enrichment analysis of the genes in (C); E) Heatmap showing the part of genes in VviEDR1-chi&pm-upregulated genes set, the value is normalized by z_score method; the free SA (F) and ethylene (G) content of VviEDR1-chi and WT at 14 dpi. Each data point represents the mean ± SD of triple biological replicates. Different letters above the bars indicate significant differences (P < 0.05), determined by Student's t test.
Many of the genes identified in the VviEDR1-chi&pm-upregulated gene set are involved in plant defense responses (Supplementary Table S3). Substantially, the VviEDR1-chi&pm-upregulated gene set contained at least 149 genes encoding leucine-rich repeat domain (LRR): there were 51 genes encoding LRR-receptor-like protein kinase (LRR-RLK) which usually act as receptors to transmit signaling information during pathogen invasion, and 29 genes encoding a nucleotide-binding site LRR (NLR) disease-resistance protein, among which 8 genes encoding Toll/interleukin-1 receptor (TIR) type NLR as well as 11 genes encoding a coiled-coil (CC) type NLR and 3 resistance powdery mildew 8 (RPW8) type NLR. In addition, EDS1 and SAG101 (senescence-associated carboxylesterase 101), which can form complexes that participate in SA biosynthesis and trigger systemic cell death, were more highly expressed in VviEDR1-chi lines at 2 dpi, and no substantial difference was found between VviEDR1-chi lines and the WT at 6 dpi. Nineteen genes encoding glutathione S-transferase (GST) were present in the VviEDR1-chi&pm-upregulated gene set. The GSTs are involved in regulating the cellular redox state, which often fluctuates during defense responses. Thirteen calcium-binding proteins were observed in the VviEDR1-chi&pm-upregulated gene set, whose synthesis was substantially upregulated at the late stage (6 dpi) of infection in VviEDR1-chi lines compared to that in the WT. Interestingly, seven genes encoding flavin adenine dinucleotide (FAD)-binding domain-containing proteins were also identified as part of the VviEDR1-chi&pm-upregulated gene set. The transcriptional level of FAD was not substantially different in WT with or without powdery mildew infection, but they were highly expressed in VviEDR1-chi lines after infection, especially at 6 dpi. The VviEDR1-chi&pm-upregulated gene set contained at least 56 genes encoding transcription factors, including 11 genes encoding the NAC family, which play an important role in plant senescence and biological stress response; nine genes encoding AP2/ERFs (ethylene-responsive transcription factors) which are participated ethylene-mediated signaling and respond to pathogens; and seven genes encoding WRKYs, including WRKY6 and WRKY51, which were confirmed to regulate the SA and ROS signaling pathways (Fig. 7E). To further understand the resistance mechanisms activated by the VviEDR1 mutation, we measured the levels of free SA and ethylene in WT and VviEDR1-chi lines at 14 dpi, SA and ethylene were detected at higher levels in the leaves of VviEDR1-chi lines than in the WT (Fig. 7, F and G).
Discussion
Genome editing techniques have been developed to achieve precise and predictable plant genome modifications for obtaining desired traits and have given rise to precision breeding techniques, which are defined as the next generation of plant breeding (Gao 2021). The CRISPR/Cas system has become the most widely used system for plant genome editing because of its low cost, simplicity, and high efficiency (Shan et al. 2013; Gao 2021; Li et al. 2022). The long juvenile phase and high heterozygosity of grapevine make it difficult to perform conventional cross-breeding, and the invention of CRISPR genome editing technology undoubtedly added wings to grapevine breeding. At present, a large number of grapevine-edited lines have already been obtained using CRISPR/Cas, such as mlo3, wrky52, epfl9-1, pr4b, and so on (Wang et al. 2018; Li et al. 2020; Wan et al. 2020; Clemens et al. 2022), which provided valuable materials for grapevine gene function research and breeding. In this study, we obtained grapevine VviEDR1-edited lines using CRISPR/Cas9, including three classical genome editing types: homozygous, bi-allelic, and chimeric (Charrier et al. 2019), and both bi-allelic edited lines and VviEDR1-chi lines displayed resistance to powdery mildew.
Consistent with Arabidopsis, grapevine EDR1 plays a negative role in powdery mildew resistance. In our study, sparser hyphae, fewer conidiophores and more abnormal haustoria were observed on the leaves of the VviEDR1-edited lines with powdery mildew inoculation (Fig. 4 and Supplementary Fig. S7). Typical disease-resistant phenotypes of edr1 were observed in the leaves of VviEDR1-chi lines, including H2O2 accumulation, callose deposition, and higher SA content (Figs. 4 and 7F) (Frye and Innes 1998). Similar to Arabidopsis edr1, grapevine edr1 mediates resistance to powdery mildew also partly relies on hypersensitive response-like cell death, whereas powdery mildew resistance in hexaploid wheat edr1 is independent of cell death (Frye and Innes 1998; Zhang et al. 2017). EDR1 is a negative regulator of ethylene-induced senescence. However, ethylene-induced responses are not required for edr1 resistance in Arabidopsis (Frye et al. 2001). In our study, a high ethylene content was detected in VviEDR1-chi lines (Fig. 7G). Our findings agreed with those of Hu et al. (2021), who reported that ethylene enhanced powdery mildew resistance in grapevines. Therefore, ethylene may be involved in the EDR1-mediated resistance to powdery mildew in grapevine. However, further studies are required to confirm this phenomenon. Consistent with a previous study, many well-known disease resistance-related genes were upregulated in VviEDR1-chi lines relative to the WT after inoculation with En NAFU1, including EDS1, SAG101, and NLR disease-resistance proteins. Some genes that participate in plant oxidoreductase reactions and cellular redox state regulation were also induced in VviEDR1-chi lines (Christiansen et al. 2011). These results suggest that the VviEDR1-edited lines regulate grapevine resistance to powdery mildew by activating multiple defense pathways.
In previous studies, the Arabidopsis edr1 mutant was associated with only marginal reductions in growth (Frye and Innes 1998; van Hulten et al. 2006), and the rice edr1 mutant started developing tiny spontaneous lesions on the leaves after the five-leaf stage. Others started developing similar lesions at the tillering stage, and these lesions became obvious at the booting stage (Shen et al. 2011; Ma et al. 2021). In this study, unlike atedr1 and osedr1 mutants, the VviEDR1 homozygous and bi-allelic edited lines, in which VviEDR1 was completely mutated (vviedr1), exhibited severe growth defects. These vviedr1 mutants developed spontaneous disease-like lesions (nonpathogen-induced dead cells) on their stems and leaves, which increased in number and size and finally induced plant death (Fig. 3). Considering that grapevine is a perennial woody plant but Arabidopsis and rice are annual plants, it is possible that the strength of EDR1 differed among plant species during growth.
To our great surprise, the VviEDR1 chimeric edited lines showed no growth defects and exhibited durable, excellent resistance to powdery mildew throughout the years of observation (Figs. 4, 6 and Supplementary Fig. S9). Chimeric mutations created by CRISPR/Cas9 refer to more than two mutated alleles in a single line (Charrier et al. 2019). Usually, the chimeric edited line would be ignored because of its imperfect editing type (Ishizaki 2016). However, we observed that the clones of the VviEDR1-chi line inherited the resistance phenotype of the VviEDR1-chi line (Supplementary Fig. S8), indicating that the chimeric edited lines were stable. This provides an idea for grapevine disease resistance breeding, in that the gentle editing mode was able to balance growth retention and disease resistance.
In this study, the pattern of VviEDR1 mutations created by CRISPR/Cas9 in the VviEDR1-chi lines was analyzed. In the terminal and axillary buds of VviEDR1-chi lines, no or very few mutations (<1.01%) in the VviEDR1-T3 target site (Fig. 5D). This was interesting because the data were acquired from VviEDR1-chi lines grown in a greenhouse for more than 2 years. Additionally, no mutation of VviEDR1 was detected in the aerial roots produced at the base of the stem or in adventitious roots produced by stem cutting (Fig. 5F). That is, the VviEDR1 kept the original sequence almost unchanged over time in the apical or lateral meristems. CRISPR/Cas9-based genome editing technologies take advantage of Cas9 nucleases to induce DNA double-strand breaks (DSBs) at desired locations within the genome. The DSB genotoxic DNA lesions can cause chromosomal rearrangements, genomic instability, and cell death if left unrepaired. The two major pathways in eukaryotes that repair DSBs are error-free-template-dependent homologous recombination (HR) and error-prone-template-independent nonhomologous end joining (NHEJ) (Thacker and Zdzienicka 2004; Xu and Xu 2020). Thus, the efficiency of genome editing is influenced by DSB incidence, DSB repair capability, and DSB repair pathways. Hence, three possible hypotheses were made: (i) The Cas9 may be less efficient in SAMs. Previous studies have shown that gene-editing efficiency is positively associated with Cas9 gene expression (Wang et al. 2022a; Gu et al. 2023). We observed that the transcriptional levels of Cas9 in the terminal and axillary buds were lower than those in the leaves and stems (Supplementary Fig. S13B). We also verified that the editing ratio positively associated with the Cas9 transcript by comparing the relative transcription of Cas9 in the same age leaf of different lines (Supplementary Fig. S13A). (ii) In SAMs, the cell with DSBs may be eliminated by activating apoptosis. Studies have reported that DNA damage activates apoptosis in cells with prolonged replication and differentiation periods. This is probably because it is more efficient and perhaps safer to maintain genome stability and DNA integrity by simply replacing the lost cells (Lee and McKinnon 2007; McKinnon 2013). This mechanism may also be applicable to plant meristem, as SAM cells have a prolonged period of cellular replication and differentiation, and apoptosis may be induced in the cells with DSBs caused by CRISPR/Cas9 (Traas and Vernoux 2002). (iii) In SAMs, the DSB may be repaired by HR. In mammals, embryonic stem cells or proliferating cells display a high frequency of HR; in contrast, NHEJ has been utilized in differentiating cells (Essers et al. 2000; Orii et al. 2006; McKinnon 2013). And the plant SAMs are composed of proliferating embryonic cells (Traas and Doonan 2001). Therefore, in the SAM of VviEDR1-chi lines, VviEDR1 may recover the original sequence suffering from DSB caused by Cas9 through the subsequent repairment by HR. Nevertheless, the VviEDR1 remains almost unmutated in the SAM of VviEDR1-chi lines, contributing to common plant growth. We hypothesized that this phenomenon is widespread in eukaryotes and maintains species stability.
We also observed that the proportion of mutated VviEDR1 in the lower leaves, which appeared at an earlier stage of development in plants, was higher than that in the upper leaves of VviEDR1-chi lines (Fig. 5C). This finding is consistent with the results of a previous study. The ratio of mutated cells was higher in the lower and older leaves than in the newly appearing upper leaves of VviPDS edited lines obtained using the CRISPR/Cas9 system (Nakajima et al. 2017). We also found that the proportion of VviEDR1 mutations increased with the leaves growing. Cas9 remained highly expressed in the leaves of different ages (Supplementary Fig. S13C), indicating that the constant working of the CRISPR/Cas9 system during leaf growth contributes to the accumulation of mutations during leaf maturation. Mature leaves containing mutated cells supported the disease resistance of VviEDR1-chi lines. Interestingly, the chimeric trait was inherited through vegetative propagation, and different clones from the same line exhibited consistent mutation regulation, relatively stable mutation ratio, and strong resistance to powdery mildew (Supplementary Figs. S8 and S10). As an integrated outcome, the VviEDR1-chi lines maintained the dynamic stability of the VviEDR1 mutation, along with the new leaves growing and the senescent leaves defoliating, which endowed VviEDR1-chi lines disease resistance without growth penalty.
Based on the results of this study, we propose a model that describes how VviEDR1-chi lines resist powdery mildew without growth penalty (Fig. 8). The VviEDR1 mutation increases powdery mildew resistance at the expense of severe growth defects. The VviEDR1 homozygous and bi-allelic edited lines died due to the induction of a severe spontaneous hypersensitivity reaction by the VviEDR1 mutant completely. In VviEDR1-chi plants, almost no mutated VviEDR1 existed in the apical meristem, so the cells of the shoot apical meristem could divide and differentiate normally to maintain plant growth. While the VviEDR1 mutation ratio increased with increasing leaf age, abundant mutations accumulated in mature leaves, conferring leaves resistance to powdery mildew by H2O2 accumulation and hypersensitive reaction-like cell death in the impacted epidermal cells and neighboring cells. As an integrated outcome, the chimeric-edited lines maintained the dynamic stability of the VviEDR1 mutation and endowed VviEDR1-chi lines disease resistance without growth penalty.

The model illustrating the VviEDR1-chi lines improve the resistance to powdery mildew without growth penalty. The death of VviEDR1 homozygous and bi-allelic edited lines was induced by the severe spontaneous hypersensitive reaction by VviEDR1 mutant completely. While the transgenic plants expressing both wild-type VviEDR1 and mutant Vviedr1 alleles as chimera (VviEDR1-chi) kept a dynamic stability of the VviEDR1 mutation, because almost no VviEDR1 mutated in the apical meristem, the cells of shoot apical meristem could divide and differentiate normally for plant growth, and the VviEDR1 mutation ratio increased with the leaf age increasing, resulting in abundant mutations accumulation in the mature leaves, conferring leaf resistance to powdery mildew (PM); WT, wild type.
Conclusions
VviEDR1 was a negative regulator of powdery mildew resistance in grapevine. Targeted mutation of VviEDR1 in powdery mildew-susceptible grapevine resulted in solid enhancement resistance. VviEDR1-chi lines used the dynamic stability of the VviEDR1 mutation to balance continuous growth and disease resistance.
Materials and methods
Plant material and cultures
The Vitis vinifera cv. Thompson Seedless was used for protoplast isolation and gene cloning upon powdery mildew infection. The pro-embryonic masses (PEMs) of “Thompson Seedless” for genetic transformation were maintained in the dark at 25°C and transferred to fresh media monthly. The wild-type “Thompson Seedless” and VviEDR1-chi lines used for evaluating the resistance to powdery mildew and transcriptome assay were cultivated in a chamber, with the temperature controlled between 22°C and 26°C under 14 h daylength (10 h dark daily). The WT and VviEDR1-chi lines used to detect the natural occurrence of powdery mildew were grown in a greenhouse at Northwest A&F University, Yangling, Shaanxi, China. The temperatures range from 25°C to 35°C (in summer) or 15°C to 25°C (in winter), with relative humidity ranging from 50% to 75% and natural sunlight.
Cloning and sequence analysis
The VviEDR1 gene was amplified from “Thompson Seedless” leaf cDNA using Planta Max Super-Fidelity DNA Polymerase (Vazyme Bio Co., Nanjing, China). The amplified sequences were integrated into the pMD-19T vector and sequencing. The amplification primers were designed according to the genome database of V. vinifera cv. Pinot Noir in the NCBI database (https://www.ncbi.nlm.nih.gov/). The sequences of amplified primers are shown in Supplementary Table S4.
The coding sequences of VviEDR1 gene obtained by sequencing were translated into protein sequences by DANMAN, and then BLAST-P search was performed in NCBI. The genes with the highest similarity to VviEDR1 in other species were selected, and the phylogenetic tree was constructed by MEGA-X, using the Neighbor-Joining method. The parameters were set as follows: bootstrap value was 1000, P-distance model was selected, partial deletion value was 50, and other default settings. The protein sequences of VviEDR1 and AtEDR1 were analyzed by Jalview, and the parameters were set by default.
Design of sgRNA targets and construction of CRISPR/Cas9 vectors
The CRISPR/Cas9 target sites of VviEDR1 were designed using the online tools CRISPR-P 2.0 (http://crispr.hzau.edu.cn/cgi-bin/CRISPR2/CRISPR) (Liu et al. 2017), and three single guide RNA (sgRNAs) targeting VviEDR1 exons were designed (Supplementary Table S5). The VviEDR1-T1 targeted by sgRNA1 was positioned upstream of the MAP3K-like domain, and a restriction enzyme site (Xap I, A/AATTC) was included at the Cas9 endonuclease cutting site (3-bp upstream 5′-NGG). VviEDR1-T2, targeted by sgRNA2, was located in the MAP3K-like domain, containing a restriction enzyme site (Mva1269 I, GAATGCN/N). These cleavage sites were used to detect the sgRNA activity by PCR/restriction enzyme assay. Additionally, VviEDR1-T3 targeted by sgRNA3 was positioned in the MAP3K-like domain of VviEDR1, which is at approximately 150 bp downstream of the VviEDR1-T2 in the genome. sgRNAs were then cloned into the CRISPR vectors, according to the method described by Xing et al. (2014). Schematic representations of the target sites of one or two sgRNAs and the pKSE401 vector integral gene expression cassettes were shown in Supplementary Fig. S1. Double-stranded inserts of sgRNA1, 2, or 3 were ligated into the pKSE401 vectors, which contained the Cas9 expression cassette, using T4 ligase. The sgRNA2 and sgRNA3 were first linked through pCBC-DT1T2 and then connected to the pKSE401 vector to construct CRISPR/Cas9 vector targeting both VviEDR1-T2 and VviEDR1-T3 (hereafter referred to as VviEDR1-T2&T3).
Detection of sgRNA activities using the grapevine protoplast system
The CRISPR-mediated mutagenesis of the VviEDR1 was evaluated by transient expression in grapevine mesophyll protoplasts. Grapevine mesophyll protoplasts were isolated and transformed as described previously (Zhao et al. 2016). Briefly, the protoplasts were extracted from grapevine young leaves and then transfected with 40 μg CRISPR/Cas9 plasmids containing sgRNA1, sgRNA2 and sgRNA2&3 by PEG-mediated transfection. The transformed protoplasts were incubated at 23°C for 48 h, and two rounds of PCR/restriction enzyme assay were used to detect mutations (Lin et al. 2018). Transformed protoplasts were collected by centrifugation at 12,000 × g for 2 min at room temperature. The genomic DNA was extracted using the CTAB-based method (Doyle and Doyle 1990). PCR primers were designed to amplify a 300 to 600 bp genomic region targeted by the sgRNA that contained the restriction site within or close to the middle of the amplicons. The amplicons were digested with restriction enzyme, Xap I was used to digest the amplicon containing VviEDR1-T1, and Mva1269 I was used to digest the amplicons containing VviEDR1-T2. The enzyme-digested products were used as templates for the second round of amplification and digestion, as described above. The final enzyme-digested products were separated on a 1.2% (mass/volume) agarose gel to detect digested fragments. The final undigested fragments were cloned into the pMD19-T Vector Cloning Kit (TaKaRa, China) for Sanger sequencing. The primer sequences are shown in Supplementary Table S6.
Agrobacterium tumefaciens-mediated transformation of grapevine PEMs
The PEMs of “Thompson Seedless” used for transformation were induced by immature stamens according to a previous study (Zhou et al. 2014). Transformation was performed using an A. tumefaciens-mediated transformation system, as previously described (Wan et al. 2020). The constructed pKSE401 plasmids containing VviEDR1-sgRNA1, -sgRNA2, -sgRNA3, and -sgRNA2&3 were transferred into competent A. tumefaciens GV3101, respectively. Then, the A. tumefaciens was co-cultured with PEMs for 2 d, and the excess bacteria were washed away. After that, the PEMs were transferred to KBNcc delayed-screening medium (MS, 30 g·L−1 sucrose, 0.3 g·L−1 KNO3, 1.126 mg·L−1 6-BA, 1 g·L−1 Myo-inositol, 0.505 mg·L−1 NOA, Phytagel, 200 mg·L−1 Cef, 100 mg·L−1 Carb) and cultured for 4 weeks. After this delayed screening, the transformed PEMs were transferred to an X3kcc resistance screening medium (MS, 30 g·L−1 sucrose, 1 g·L−1 activated charcoal, Phytagel, 200 mg·L−1 Cef, 100 mg·L−1 Carb, 75 mg·L−1 Kan) until the germinated embryos developed cotyledons. Then, the cotyledon embryos were transferred to a germination medium (15 g·L−1 sucrose, MS, Phytagel) and placed under light for further development. After true leaves appeared, the cultured plantlets were transferred to the rooting medium (30 g·L−1 sucrose, MS, 1 g·L−1 activated carbon, Phytagel, 1 mg·L−1 IBA), and finally, the regenerated plants were transplanted into the growing substrate.
Genotyping and mutant verification
Genomic DNA was extracted from the leaves of regenerated plants and used as a template to amplify the DNA fragments covering the target sites with specific primers for each target, using Planta-Super-Fidelity DNA Polymerase (Vazyme Biotech Co, Nanjing, China). To verify the presence of the engineered sequences, specific oligonucleotide primers (U6-26-F and U6-26-R) were used to amplify the CRISPR/Cas9 sequence (Supplementary Table S5). Sequences containing EDR1 targets in CRISPR-positive lines were amplified and sequenced by the Beijing AuGCT Biotech Yangling Sequencing Department. The sequencing results were analyzed using DSDecodeM (http://skl.scau.edu.cn/dsdecode/) to detect and identify mutations (Liu et al. 2015). Then, the PCR amplicons were cloned into the pMD19-T Vector Cloning Kit (TaKaRa, Beijing, China), and 10 to 20 clones were sequenced and further analyzed to identify the type of mutations. DNAMAN and SnapGene Viewer software were used for sequence chromatogram analysis. Genotyping of the transgenic lines was performed as described previously (Ma et al. 2015).
Genotyping of VvEDR1-chi lines was performed using the Hi-TOM tool according to a previous study (Liu et al. 2019). The primary PCR was performed to amplify the targeted genomic DNA with a pair of site-specific primers with common bridging sequences (5′-ggagtgagtacggtgtgc-3′ and 5′-gagttggatgctggatgg-3′) added at the 5′ end. Then, the PCR amplicons were subjected to next-generation sequencing (NGS). More than 10,000 reads for each amplicon were obtained, and detailed information on the mutation types and positions of each sample was analyzed using the Hi-TOM platform (http://www.hi-tom.net/hi-tom/).
Off-target analysis
The potential off-target sites of each target in the stable transgenic lines were determined by the online tool CRISPR-GE (http://skl.scau.edu.cn/offtarget/), the 5′-NGG-3′ was chosen to be the PAM type, and the Vitis vinifera (IGGP_12x) was chosen to be the target genome (Xie et al. 2017). The top three off-target sites were chosen for analysis (Supplementary Table S1), and the specific primer pairs were designed for each off-target site (Supplementary Table S7). DNA fragments covering the off-target sites were amplified and sequenced.
Powdery mildew infection and evaluation of plant resistance
The detached leaves from the WT and VviEDR1-T3 bi-allelic edited lines #58 and #26 in the culture vessel were inoculated with the powdery mildew isolate En NAFU1, as described previously (Gao et al. 2016). Fully expanded leaves at the third node of the shoot tip were chosen for inoculation. Representative micrographs and hyphal development were observed at 1, 3, 5, and 7 days post-En NAFU1 inoculation (dpi). In addition, two VviEDR1-chi lines, #6 and #7, were transplanted into the chamber. Leaves were collected at 14 dpi, and some samples were used for histochemical staining to detect the mycelial structure of En NAFU1 and the cell reactions in grapevine leaves, and the rest of the samples were used to assess the SA and ethylene content. The VviEDR1-chi lines were also transplanted in the greenhouse, whose environment was full of En NAFU1, to observe the natural occurrence of powdery mildew in the WT and VviEDR1-chi lines.
Histochemistry stain
The phenotype of the interaction between En NAFU1 and grapevine leaves was identified using the method reported by Xiao et al. (2003). The mycelial structure of En NAFU1 and cell death in grapevine leaves at 14 dpi were observed through trypan blue staining. The 3,3′-diaminobenzidine (DAB) staining was used for detecting H2O2 outbreak in situ, and the aniline blue staining was used for detecting callose deposition in situ. The images were acquired using a microscope (BX51, Olympus, Tokyo, Japan). At least three leaves from each replicate were observed, and three biological replicates were evaluated.
SA and ethylene content determination
The free SA and ethylene contents were determined according to previous reports (Cui et al. 2020; Hu et al. 2021). Briefly, the 0.2 g grapevine mature leaves at 14 dpi were ground in liquid nitrogen, 1 mL ethyl acetate was added, and the mixture was vortexed for 10 min. Then, the mixture was centrifuged at 10,000 × g for 10 min at 4°C, and the supernatant was evaporated to dryness in a vacuum concentrator (Eppendorf, Enfield, CT, USA). The dried residue was then resuspended in 50% methanol (v/v) and centrifuged again to collect the supernatant for analysis through the HPLC-MS/MS, with a Shim-pack XR-ODS (2.0 mm I.D. × 75 mm L 1.6 μm) column coupled to a triple quadrupole mass spectrometer (LC-MS8040, Shimazu) which was equipped with an electrospray source (ESI). The peak area of the sample and the internal standard were used to quantify the free SA content (Hu et al. 2021). All measurements were performed in triplicates. The mature leaves of the WT and VviEDR1-chi lines at 14 dpi were washed with deionized water and punched into 1 cm diameter leaf discs. Then, 10 leaf discs from each sample were carefully placed in a 10 mL vial and sealed with a rubber stopper. The sealed vials were kept out of light and placed in a 23°C chamber for 48 h. Then, 1 mL of gas was removed from each vial using a syringe and injected into the gas chromatograph (Agilent, Santa Clara, CA, USA; 7890B-5977A) to determine the ethylene content.
Transcriptome and transcript analysis
The mature leaves from powdery mildew-infected WT and VviEDR1-chi lines (including #6 and #7) were collected for RNA extraction at 2 and 6 dpi, and uninfected leaves were used as controls. Total RNA was isolated using the EZNA Plant RNA Kit (R6827-01; Omega Bio-tek, USA). Approximately 2 μg of total RNA per sample was used for library construction and subjected to deep sequencing on the Illumina NovaSeq 6,000 platform (Novegene Technologies, Beijing). Prior to library construction, RNA quality and quantity were confirmed using an Agilent 2100 Bioanalyzer. RNA-seq data are available in the database of Ensemble Plants (Taxonomy ID 29760). The fragments per kilobase of transcript per million fragments mapped (FPKM) method was used to calculate normalized expression data for each library. Differentially expressed genes (DEGs) were identified by DEseq2 with the criteria of absolute log2 (fold change) ≥ 1 or 0.58 and false discovery rate (FDR) ≤ 0.05 (Love et al. 2014).
To validate the RNA-seq data, reverse transcription quantitative PCR (RT-qPCR) was conducted to analyze the transcription level of selected genes in the WT and VviEDR1-chi lines, and cDNA synthesis was performed using PrimeScript RTase (Takara Biotechnology, Dalian, China). The grapevine VviActin1 gene (GenBank accession number: AY680701) was used as a control to normalize the amount of cDNA in each sample. The RT-qPCR assays were performed as previously described (Hu et al. 2018). The relative expression level of each gene was analyzed using IQ5 software according to the Normalized Expression method. The primers used for RT-qPCR are listed in Supplementary Table S8.
Statistical analysis
Student's t-test (https://www.statskingdom.com/t_test.html) was used to examine the statistical significance of paired data, presented as the mean ± standard deviation of the mean (SD). Minimal statistical significance was set at P < 0.05 and P < 0.01 in all analyses.
Accession numbers
RNA-Seq data from this article can be found in the GenBank data libraries under accession number PRJNA1078883. Sanger sequencing data from this article can be found in the GenBank data libraries under accession number: VviEDR1 (PP375819).
Acknowledgments
We thank Dr. Qi-Jun Chen of China Agricultural University for providing the CRISPR/Cas9 binary vector pKSE401. We thank Dr. Yong-Dong Xie of Chengdu Academy of Agriculture and Forestry Sciences for data visualization processing. We thank the Horticulture Science Research Center (Northwest A&F University) for experimental assistance. We thank Dr. Shunyuan Xiao of the Institute for Bioscience and Biotechnology Research, University of Maryland College Park for polishing the language.
Author contributions
Y.Q.W. and X.N.Y. conceived the study. X.N.Y., Y.G., Q.L.Y., H.Y.Y., M.J.L., L.Z., and Z.S.J. performed the experiments. X.N.Y. and Y.G. wrote the manuscript. J.Y.F. and X.N.X. contributed to the study via consultation. Y.Q.W., X.N.Y., and Y.G. interpreted the experimental data and revised the manuscript. All the authors read and approved the final manuscript.
Supplemental Data
The following materials are available in the online version of this article.
Supplementary Fig. S1. Schematic representation of the pKSE401 vector and the VviEDR1 with target sites.
Supplementary Fig. S2. Efficiency of CRISPR/Cas9 using the grapevine protoplast transfection system.
Supplementary Fig. S3.Agrobacterium tumefaciens-mediated transformation of “Thompson Seedless” and regeneration of transgenic lines.
Supplementary Fig. S4.VviEDR1 mutation types detected in the transgenic lines via CRISPR/Cas9-mediated editing.
Supplementary Fig. S5. Spontaneous necrotic lesions on the leaves of VviEDR1-T3 bi-allelic edited lines.
Supplementary Fig. S6. Spontaneous necrotic lesions on the mature leaves of VviEDR1-chi lines.
Supplementary Fig. S7. The VviEDR1-T3 bi-allelic edited lines enhanced the resistance to powdery mildew in grapevine.
Supplementary Fig. S8. The resistance to powdery mildew was stably inherited by vegetative propagation.
Supplementary Fig. S9. The VviEDR1-chi lines enhanced the resistance to powdery mildew of natural disease in the greenhouse.
Supplementary Fig. S10. The VviEDR1 mutation types and mutation ratios in leaves of VviEDR1-chi line #7-clones.
Supplementary Fig. S11. Correlation of gene expression levels estimated by RNA-Seq and RT-qPCR.
Supplementary Fig. S12. VviEDR1 regulates genes under pathogen-free conditions.
Supplementary Fig. S13. Analysis of relative transcript expression of Cas9 and sgRNA in VviEDR1-chi lines by RT-qPCR.
Supplementary Table S1. Analysis of potential off-target sites in transgenic plants
Supplementary Table S2. The DEGs in VviEDR1-chi lines compared to the wild type in the absence of pathogen
Supplementary Table S3. DEGs of powdery mildew- and VviEDR1-induced gene
Supplementary Table S4. The primers used to VviEDR1 sequence amplified
Supplementary Table S5. Details of target sites of VviEDR1
Supplementary Table S6. The primers used to detect mutations by PCR/restriction enzyme assay and regenerated lines
Supplementary Table S7. The primers used for off-target analysis
Supplementary Table S8. The primers used for RT-qPCR
Funding information
This work was supported by the National Natural Science Foundation of China (Grant No. 31972986, 32272670) and Key Research and Development Projects of Shaanxi Province (Program No. 2023-YBNY-059).
Data availability
All data that support the results are included in this article and within its Supplementary data published online.
Dive Curated Terms
The following phenotypic, genotypic, and functional terms are of significance to the work described in this paper:
References
Author notes
Xue-Na Yu and Ye Guo contributed equally to this work.
The author responsible for distribution of materials integral to the findings presented in this article in accordance with the policy described in the Instructions for Authors (https://dbpia.nl.go.kr/plphys/pages/General-Instructions) is Ying-Qiang Wen ([email protected]).
Conflict of interest statement.All authors declare no conflicts of interest.