-
PDF
- Split View
-
Views
-
Cite
Cite
Dorian Winter, Norbert Polacek, Isabella Halama, Barbara Streicher, Andrea Barta, Lead-catalysed specific cleavage of ribosomal RNAs, Nucleic Acids Research, Volume 25, Issue 9, 1 May 1997, Pages 1817–1824, https://doi.org/10.1093/nar/25.9.1817
- Share Icon Share
Abstract
Ribosomes have long been known to require divalent metal ions for their functional integrity. Pb2+-induced cleavage of the sugar-phosphate backbone has now been used to probe for metal binding sites in rRNA. Only three prominent Pb2+ cleavages have been detected, with cleavage sites 5′ of G240 in 16S rRNA and two sites 5′ of A505 and C2347 in 23S rRNA. All cleavages occur in non-paired regions of the secondary structure models of the rRNAs and can be competed for by high concentrations of Mg2+, Mn2+, Ca2+ and Zn2+ ions, suggesting that lead is bound to general metal binding sites. Although Pb2+ cleavage is very efficient, ribosomes with fragmented RNAs are still functional in binding tRNA and in peptidyl transferase activity, indicating that the scissions do not significantly alter ribosomal structure. One of the lead cleavage sites (C2347 in 23S RNA) occurs in the vicinity of a region which is implicated in tRNA binding and peptidyl transferase activity. These results are discussed in the light of a recent model which proposes that peptide bond formation might be a metal-catalysed process.
Introduction
The ribosome is a multifunctional RNP complex composed of several highly structured RNAs which are associated with many proteins. The high evolutionary conservation of the secondary structures of rRNAs strongly argues for their importance in ribosomal function. Divalent metal ions are known to be essential for the functional integrity of RNAs, either by organizing complex RNA structures (structural metal ions) or by their direct involvement in catalytic functions (catalytic metal ions) (1). Similar to other RNA-containing enzymes, ribosomal activity is strongly dependent on the presence of divalent metal ions (2,3). Although the proposed importance of Mg2+ for ribosomes is still based on the structural aspect, we have recently suggested a model which proposes that Mg2+ ions might also be of functional significance for the translational apparatus (4).
Previously, the prevailing assumption was that the main enzymatic reaction of the ribosome, peptidyl transfer, was catalysed by protein(s), but no single protein or group of proteins was found to be capable of catalysing peptide bond formation in the absence of rRNA. However, there are several indications that RNA plays an important part in the peptidyl transferase reaction, the strongest being their high secondary structure conservation and the fact that the peptidyl transferase region is mainly composed of RNA (for recent reviews see 4–6). Furthermore, the findings of Noller and co-workers (7) that 50S subunits of Thermus aquaticus stripped of their proteins by extensive phenol extraction retain almost full activity greatly strengthened the idea that a part of 23S rRNA might be involved in catalysis of peptidyl transfer. This idea might be not so far-fetched, as model experiments have shown that RNA is able to catalyse reactions on carbon centres, such as cleavage of an amide bond (8), self-acylation of RNA with activated amino acids (9) and self-alkylation of ribozymes (10), suggesting a wide array of possible catalytic activities for RNA enzymes. Furthermore, a ribozyme which catalyses amino acid transfer reactions has been selected in an in vitro selection system (SELEX) (11).
If peptidyl transferase activity indeed belongs to RNA enzymes, there is a high probability that the reaction is catalysed by metal ions, as all RNA-catalysed reactions characterized so far have been demonstrated to involve metal ions in their catalytic mechanisms (1). We have therefore proposed that divalent metal ions coordinated by RNA are involved in catalysis of peptidyl transfer (4). There are at least three ways in which a metal ion may aid peptide bond formation: the developing negative charge on the leaving group (tRNA ribose) could be stabilized by a directly coordinated metal ion or metal ion coordination to the oxygen of the carbonyl group of the amino acid may render the carbon centre more susceptible to nucleophilic attack. Furthermore, a metal ion which coordinates a hydroxyl group could act as a general base on the amino group, enhancing the nucleophilicity of the amine nitrogen.
The proposal that metal ions are involved in catalysis is compatible with all known parameters of peptide bond formation. In a first attempt to localize high affinity metal binding sites in rRNAs we approached this issue by metal hydroxide-induced specific cleavage of rRNA.
The ability of metal hydroxide ions, in particular Pb(II) ions, to depolymerize RNA has been used to map the conformation of larger RNAs and to follow discrete structural changes at different functional stages, as described for structural probing of 5S RNA and the 3′-end of 16S RNA (12,13). In contrast, divalent metal ions bound to defined metal binding sites in highly structured RNA have long been known to catalyse site-specific cleavage in a pH-dependent manner (14). The best-studied example is lead-induced specific cleavage of yeast tRNAPhe. X-ray studies have indicated that at neutral pH a lead hydroxide ion binds to bases in the T loop close to a magnesium binding site, thus orienting the hydroxide in such a way that it specifically cleaves a phosphodiester bond in the D loop of the tRNA (15,16). Specific lead cleavage has also been exploited to probe the correct structure of various RNAs, including tRNAs (17,18) and RNase P (19,20). Furthermore, Streicher et al. (21) have identified specific lead cleavage sites in the catalytic core of group I introns. Several lines of evidence now suggest that cleavage by divalent metal ions (Pb2+, Ca2+, Sr2+, Mg2+ and Mn2+) originate from metal ions bound close to the splice sites in the catalytic centre of group I introns (22). Therefore, metal-induced RNA cleavage seems to be a useful method by which to trace high affinity metal ion binding sites in highly structured but flexible RNA domains.
Lead-induced specific cleavage can be performed at neutral pH and was therefore used to investigate general metal binding sites in 70S ribosomes of Escherichia coli. We found one prominent cleavage site in 16S RNA and two in 23S RNA. These cleavages were dependent on the structural integrity of the ribosome, on pH and they could be competed for by other divalent metal ions, indicating that they originate from a general metal binding pocket. Although the cleavage reactions were very efficient, they did not influence ribosomal function. The locations of the cleavage sites are discussed for their possible significance for ribosomal structure and function.
Materials and Methods
Materials
70S ribosomes and 50S and 30S subunits were prepared from E.coli MRE600 and K12 (strain DH1) as described (23). Preparation of Ac[3H]Phe-tRNA was basically as described previously (24). SDS-agarose gel electrophoresis of rRNA was performed according to Sambrook et al. (25).
Conditions for metal cleavage and purification of rRNA
Aliquots of 12 pmol ribosomes were incubated for 15 min at room temperature in 20 mM Tris-HCl, pH 7.4, 100 mM KCl, 6 mM MgCl2, 0.4 mM EDTA and 2 mM DTE, typically in a volume of 20 µl (standard preincubation). For metal cleavage, freshly prepared Pb(OAc)2 was added at the desired concentration and further incubated for 4 min at room temperature. The reaction was stopped by addition of excess EDTA with respect to divalent metal ion. The samples were precipitated with ethanol and pellets were resuspended in 50 µl buffer A (10 mM Tris-HCl, pH 7.4, 100 mM NaCl, 5 mM EDTA, 0.5% SDS). rRNAs were then purified by phenol extraction, ethanol/0.3 M sodium acetate precipitated and dissolved in water to a concentration of 0.3 µg/µl.
Lead cleavage of denatured ribosomes
For heat denaturation ribosomes were preincubated for 15 min as described above followed by incubation at 30, 40, 50, 60, 70, 80 and 95°C for 2 min. The samples were immediately cooled on ice to prevent renaturation and were treated for lead cleavage as previously discussed. For chemical denaturation, after standard preincubation, SDS was added to a final concentration of 0.5% (w/v). An aliquot was additionally treated with proteinase K (final concentration 1% w/v).
Competition of lead cleavage by divalent metal ions
Ribosomes (0.3 µM) were preincubated in standard buffer containing 2 mM MgCl2 and the desired concentration of another divalent metal ion (Mg2+, Mn2+, Ca2+ or Zn2+). Then Pb2+ was added to a final concentration of 5 mM and the samples were incubated for 4 min at room temperature.
Primer extension analysis
Primer extension analysis on rRNA was performed as described (26) using 19 DNA oligomers distributed over both 16S and 23S rRNAs with an average length of 270 nt. The R primers hybridize to the 23S, the S primers to the 16S rRNA. The numbers in parentheses indicate the first transcribed nucleotide on the rRNAs: R1 (255), R2 (454), R3 (670), R4 (872), R5 (1098), R6 (1347), R7 (1599), R8 (1836), R9 (1905), R10 (2042), R11 (2274), R12 (2493), R13 (2639), R14 (2887), S1 (323), S2 (683), S3 (1046), S4 (1391), S7 (1508). For analysis of 5S rRNA, primer 5S (104) was used. cDNA products were separated on a 6% polyacrylamide sequencing gel (25). Quantitative data were obtained by scanning polyacrylamide gels of the competition experiments using a Molecular Dynamics PhosphorImager.
Tests of ribosomal function
Standard lead cleavage reactions of 70S ribosomes were with 5 mM Pb(OAc)2. The reaction was stopped by addition of EDTA to a final concentration of 5 mM. To remove lead and EDTA, the ribosomes were purified by gel filtration on a Sephacryl S-300 column (cDNA spun column; Pharmacia) equilibrated in buffer B (20 mM HEPES, pH 7.4, 150 mM NH4Cl, 3 mM MgCl2, 2 mM spermidine and 0.05 mM spermine). The columns were centrifuged at 600 g at 4°C for 10 min.
For the binding assay, 8 pmol ribosomes were incubated with 4 pmol Ac[3H]Phe-tRNA (specific activity 2.8 × 104 c.p.m. pmol) in a final volume of 40 µl buffer B for 10 min at room temperature. Aliquots of 20 µl of the reaction were filtered through a cellulose nitrate membrane (0.45 µm; Millipore) whereby ribosome-associated tRNA was bound to the filter. The filter was washed twice with 2 ml buffer B and the radioactivity measured in a scintillation counter.
For the puromycin assay, puromycin was added to the binding reaction to a final concentration of 1 mM. After incubation for 20 min at room temperature or overnight at 0°C an equal volume of 0.3 M sodium acetate in saturated MgSO4 and 10 vol ethyl acetate were added and shaken vigorously. The Ac-[3H]Phepuromycin product was counted in the organic phase.
Results
Lead-induced specific cleavage of ribosomes
Ribosomes from E.coli were incubated for 4 min in standard incubation buffer supplemented with various concentrations of Pb2+ ranging from 0 to 10 mM. rRNAs were isolated and used as templates in primer extension analyses. The primers chosen were spaced every ∼250 nt on the 16S, 23S and 5S rRNAs, so we were able to scan the entire RNAs except for the 3′-ends. Three very prominent cleavage sites seen as strong stops of reverse transcriptase could be observed in the 16S and 23S rRNAs starting from 3 mM Pb2+ (Fig. 1). No such strong band was present in the 5S rRNA (data not shown). Sequence reactions run in parallel revealed one prominent cleavage site at G240 on 16S rRNA (the cleavage occurs 5′ of the indicated nucleotide) and two at A505 and at C2347 on 23S rRNA (Fig. 1). At higher lead concentrations double cuts at G240 and C2347 could be identified. Figure 2 shows the placement of the three strong lead cleavage sites in a 2-dimensional model of the rRNAs. All three sites occur in single-stranded regions.
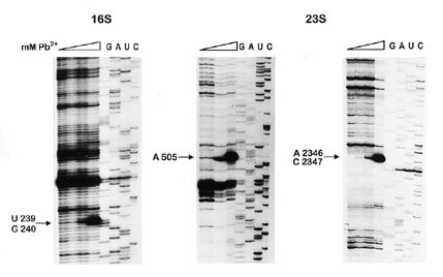
Primer extension and sequence analysis of Pb2+ cleaved 16S and 23S rRNA. Incubation of 70S ribosomes with Pb2+ was performed as described in Materials and Methods. The Pb2+ concentrations were 0, 3, 5 and 10 mM. Lanes G, A, U and C are dideoxynucleotide-terminated sequence reactions carried out on unmodified rRNA whereby the letters denote the nucleotide on the rRNA. Only the three regions with high efficiency cleavages are shown. Positions at which reverse transcriptase is halted due to cleavage of the RNA are indicated by an arrow and the identity and position of the last nucleotide incorporated is indicated.
Four independent preparations of ribosomes were used for the lead-induced specific cleavages and only one exhibited an additional strong cleavage site in the 16S rRNA, which we attributed to an isolation artefact of this particular ribosome preparation. No change in the cleavage pattern was observed when isolated 30S and 50S subunits were used in the incubation. At 10 mM Pb2+ and higher concentrations we observed several additional weaker cleavages (seven in the 16S and 10 in the 23S rRNA), but in the following analyses we concentrated solely on the three specific strong cleavage events.
To better estimate the efficiency of the three major specific lead cleavage reactions, the same RNAs used in the primer extension analyses were seperated on an agarose gel. Figure 3 shows that all RNA fragments predicted from cleavage at the three sites were present. The efficiency of the cuts in the 23S rRNA was quite high, as only a small amount of full-length 23S rRNA was still visible after incubation for 4 min at 5 mM Pb2+ and also fragments A+B and B+C almost disappear at 10 mM Pb2+. The existence of fragment B demonstrates that both cuts (A505 and C2347) can occur in the same 23S molecule. The cut in the 16S RNA seems to be somewhat less efficient and the 240 nt fragment D is not well resolved on the agarose gel. These results clearly show that the observed stops in the primer extension assay are in fact due to metal-induced strand scisson. The relatively high efficiencies of the cleavage reactions suggest that Pb2+ is bound to strong metal binding sites.
pH dependence of the lead cleavage reactions
In order to find out if the reacting species in lead cleavage could be a Pb(OH)+, as has been suggested for lead-induced cleavage of tRNAs (15), the susceptibility of rRNA to lead cleavage at pH 6.6, 7.0 and 7.4 was investigated. As the pKa of a water molecule bound to Pb2+ is ∼7.7 (1), a reduction in the efficiency of the cleavage reaction should be observed at lower pH. Figure 4 shows that the efficiencies for all three cleavage reactions are greatly reduced. Whereas cleavage at C2347 is strongly reduced at lower pH, some cleavage at A505 and G240 could still be obtained at pH 6.6 at 10 mM Pb2+. These results support the hypothesis that a hydroxide ion bound to Pb2+ is the reacting species.
Competition experiments with other divalent metal ions
Crystallographic studies of yeast tRNAPhe revealed that the binding site for a Pb2+ ion, which specifically cleaves the tRNA, overlaps with a strong Mg2+ binding site (16). Recently, a thorough analysis of the catalytic core of a group I intron has led to the characterization of high affinity metal binding pockets where several divalent metal ions, including Pb2+ and Mg2+, can be accomodated (22). Thus, we reasoned that the specific Pb2+ cleavage sites found in highly structured regions of rRNA might not be only indicative of a distinct Pb2+ binding site nearby, but also of a high affinity Mg2+ binding site. Therefore, we perfomed competition experiments with Mg2+ as well as with Mn2+, Ca2+ and Zn2+. Mn2+ and Ca2+ were chosen because they support ribosomal function when substituted for Mg2+, whereas Zn2+ is inhibitory. In these experiments Mg2+ and Pb2+ concentrations were kept at a constant level of 2 and 5 mM respectively, whereas the competing metal concentrations were raised to 100 mM. Figure 5 shows that cleavage was diminished at all three sites and the efficiency of competition was dependent on the competing metal ion. Quantitative evaluation revealed that Mg2+ ions were the least efficient competitors of lead cleavage at all three sites. In contrast, Mn2+, Ca2+ and Zn2+ ions were more effective, with the exception of Zn2+ at G240 in 16S rRNA. In general, cleavage at A505 and C2347 in 23S rRNA was more sensitive to competing divalent metal ions than cleavage at G240. These data suggest that the three strong lead cleavages originate from lead ions bound to general metal binding pockets.
Binding of tRNA and antibiotics does not influence lead cleavage
As metal ions are often involved in catalysis and as practically every step of translation can be inhibited by antibiotics, the influence of antibiotics on lead cleavage was investigated. An observed effect might shed light either on the location of the metal binding sites in the ribosome or on possible antibiotic-induced conformational changes. Therefore, lead cleavage was performed in the presence of 10–1000 µM of the following antibiotics that are known to interact with the ribosome: chloramphenicol, erythromycin, puromycin, sparsomycin, streptomycin, tetracycline, thiostrepton, tiamulin and viomycin. None of these antibiotics had an effect on lead cleavage (data not shown). Futhermore, the influence of tRNA binding on the cleavage reaction was investigated, but no change in the efficiency of the cleavage reactions upon binding of tRNA could be detected (data not shown).
Structural dependence of the lead cleavages
As metal binding and RNA hydrolysis are dependent on the correct RNA structure, we disturbed the structure of rRNA in two ways. Heat denaturation of ribosomes and subsequent treatment with lead (at room temperature) showed a clear correlation between rise in temperature and loss of cleavage. At all three sites lead cleavage gradually disappeared when ribosomes were heated above 70°C (data not shown). On the other hand, ribosomes denatured with 1% proteinase K, 0.5% SDS for 1 h showed that cleavage at A505 was not notably affected by this treatment, whereas the efficiency of cleavage was clearly diminished at G240 and C2347 (data not shown).
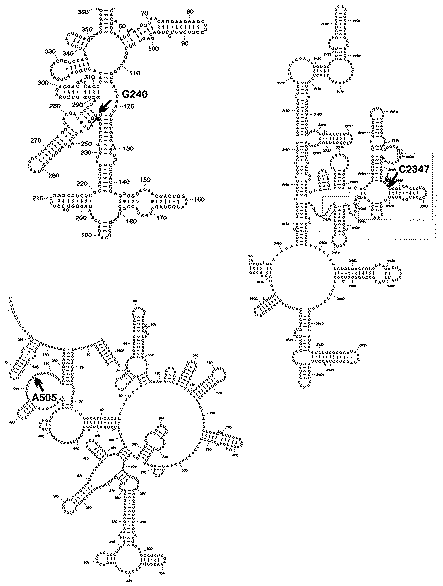
The positions of the three strong Pb2+ cleavage sites on the secondary structure model of 16S and 23S rRNA (45). Only the relevant regions for G240 on the 16S rRNA and for A505 and C2347 on the 23S rRNA are shown. Thick arrows denote the positions of the three strong cleavage sites, the thin arrows indicate the two adjacent minor cleavage sites, which are only visible at 10 mM Pb2+. Tertiary interactions are connected by thin long lines or dotted lines.
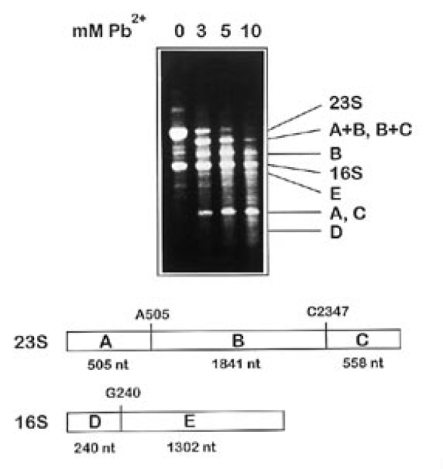
Separation of rRNAs from Pb2+-cleaved 70S ribosomes on an agarose gel. Ribosomes were incubated with various concentrations of Pb2+, RNA isolated and run on a 1.5% agarose gel with 0.5% SDS. Ethidum bromide staining of the gel reveals all the RNA fragments expected from the three strong cleavage sites. Schematic representations of these events are depicted below.
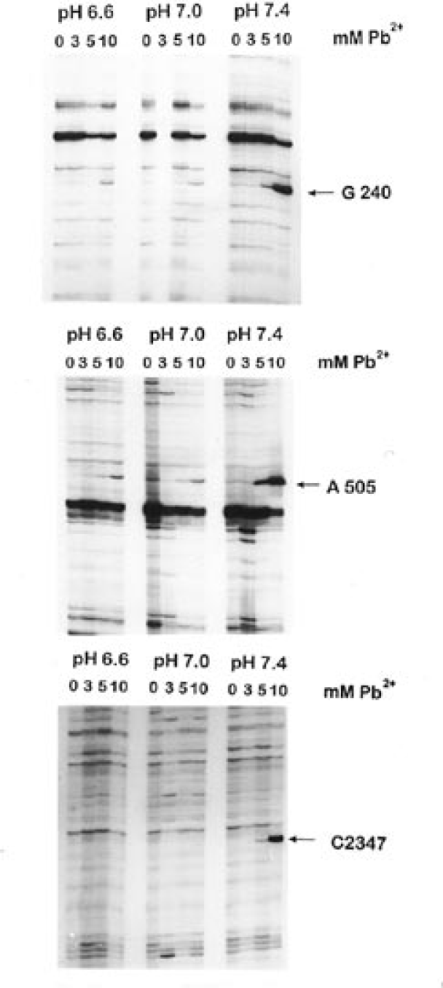
Analysis of the pH dependence of the Pb2+ cleavage reaction. Standard incubations with various concentrations of lead were done under three different pH conditions. Primer extension analysis of the three strong cleavage sites are shown and the positions of the nucleotides are indicated with an arrow.
Activity of lead-treated ribosomes
To analyse the influence of the fragmented rRNAs on the functionality of ribosomes, their ability to bind tRNA and perform peptide bond formation was measured. After lead treatment, which was stopped by addition of EDTA, the ribosomes were purified by gel filtration. As a control, ribosomes were incubated with an equimolar mixture of Pb2+ and EDTA and purified as described. Binding activity and reactivity towards puromycin were measured for each ribosome preparation and compared with untreated ribosomes. Each assay was done simultaneously with three aliquots and the whole experimental sequence was repeated twice. As can be seen in Figure 6, both ribosomal activities were virtually unaffected by fragmentation of the rRNA with lead ions.
Discussion
Divalent metal ions, in particular Mg2+, have long been known to be crucial for formation and maintenance of the active ribosomal structure and neither monovalent metal ions nor spermidine nor putrescine can substitute for a certain amount of divalent metal ions (2,27–29). However, there has been no direct demonstration of a catalytic metal ion involved in peptide bond formation. Because no metal binding site has ever been mapped to any of the rRNAs we set out to investigate this issue by lead-induced cleavage of rRNAs.
Analysis of specific lead cleavage sites in highly structured RNA has been widely used as a probe for the integrity of tertiary structures of RNAs and for identifying potential catalytically important metal ion binding sites (17–22). Treatment of ribosomes from E.coli with lead ions revealed three strong cleavages in rRNAs, two in the large and one in the small subunit. We propose that these cuts result from Pb2+ ions bound to highly structured regions of rRNA which can form metal ion binding pockets.
X-ray data on yeast tRNAPhe crystals soaked with Pb2+ have shown that the binding site of the cleaving Pb2+ overlaps a Mg2+ binding site (16). As both ions cannot bind simultanously, the Pb2+ must be able to displace Mg2+ from its binding site. The fact that all three cleavages can be competed for by higher concentrations of Mg2+, Mn2+, Ca2+ and Zn2+ suggests that lead was bound to a general metal binding site. Similar results have been obtained for group I introns (21) and RNase P (19). Furthermore, the clear pH dependence of the lead cleavage argues for the involvement of Pb(OH)+, as was proposed for cleavage of tRNAPhe.
The existence and number of high affinity metal binding sites in ribosomes have not been determined. Here we present evidence for three metal binding sites which bind Pb2+ in such a manner that they position a hydoxyl group in the correct orientation and distance to effect proton abstraction from a 2′-OH of a ribose. There are probably many more metal binding sites in rRNA, as in yeast tRNAPhe three lead binding sites have been identified by X-ray crystallography but only one Pb2+ ion cleaves tRNA efficiently. Another prerequisite for efficient cleavage is a certain flexibility of the region to be cleaved and, indeed, all three cleavages occur at flexible hinge regions.
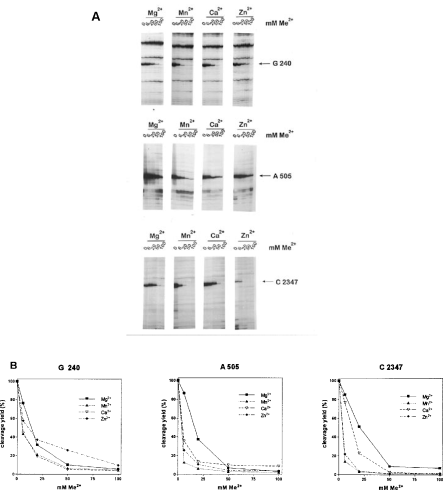
Competition experiments of the lead cleavage reaction with four different metal ions. Lead cleavage reactions were done in buffer containing 2 mM Mg2+ and 5 mM Pb2+ with increasing amounts of either Mg2+, Mn2+, Ca2+ or Zn2+. (A) Primer extension analysis of the three strong Pb2+ cleavage sites are shown and the positions of the nucleotides are indicated with an arrow. (B) Quantitative PhosphorImager analysis of the competition experiments.
The relatively few additional cuts which are visible at higher concentrations of lead (>10 mM) reflect the compact and highly ordered structure of rRNA in the ribosome, because unstructured RNA is efficiently depolymerized by lead ions (30). In this respect it is interesting to note that we could still detect lead cleavage at A505 and G240 in rRNA which was purified twice by phenol/SDS extraction (unpublished observation). This indicates that a part of the rRNAs retain their structure without proteins, although ribosomal proteins may also help to protect the structure of rRNA. This assumption seems very likely, as Gornicki and co-workers (12) have probed the 3′-end of 16S RNA and found that two strong lead cleavage sites were protected in the 30S subunits and 70S ribosomes.
Sometimes single cuts in the rRNA, like those caused by the drugs colicin E3 (31) and α-sarcin (32), result in complete inhibition of ribosomal function. In contrast, several sites in rRNAs are known whose cleavage does not affect ribosomal function, as naturally fragmented rRNA exists in certain species (reviewed in 33). The three Pb2+ cleavage sites described in this paper are very efficient, but they do not inhibit tRNA binding or peptidyl transferase activity. Therefore, the three sites are located at positions where cleavage does not disturb any essential structure of the rRNA in any significant way.
![Activity of lead-cleaved ribosomes. Binding of Ac-Phe-tRNA (2 pmol) and peptidyl transferase activity of lead-cleaved ribosomes (4 pmol) were compared with untreated ribosomes and ribosomes incubated under the same conditions with Pb2+/EDTA. Specific activity of the Ac[3H]Phe-tRNA: 2.8 × 104 c.p.m./pmol.](https://oup.silverchair-cdn.com/oup/backfile/Content_public/Journal/nar/25/9/10.1093_nar_25.9.1817/2/m_25-9-1817-fig006.gif?Expires=1750735981&Signature=H9eg3shJuKzbSHvWg-ePiFU3c6CdzLDE6taDGPwcWIVxFf~TZYHmZvY4wBCF-4LDhKL9NN7I0mBLDsKni~0dHcgG2KiMfKeDPob0GGnCD0w7D8hJZZOCrm~Y~AI9AZi4esugd7RZqERA9G4578lWrLoRELaLLzNM8Ld9E-ZGy3bZcRTyaJMYsHVEFHhhdBBw6WCPGPNHXY5ypdyL-WGXWw2PzGTN71eT2cgYxdGUx261ORwxVEH1CCgaCCzRuBPLDRjRxCm3WqCOZ-NInV9WgV3jn~XTrDG~rCUw~90WNKMMoYtn2ZMpk46cmv5aUk8i0OlMaLwnVrc7gqqZ9yLUjQ__&Key-Pair-Id=APKAIE5G5CRDK6RD3PGA)
Activity of lead-cleaved ribosomes. Binding of Ac-Phe-tRNA (2 pmol) and peptidyl transferase activity of lead-cleaved ribosomes (4 pmol) were compared with untreated ribosomes and ribosomes incubated under the same conditions with Pb2+/EDTA. Specific activity of the Ac[3H]Phe-tRNA: 2.8 × 104 c.p.m./pmol.
It was proposed for some antibiotics that they might act by distortion of rRNA (34). Therefore, we investigated the effects of several antibiotics on lead-catalysed hydrolysis. The fact that none of the identified cuts were influenced gives us further information about the divalent metal ion binding sites: (i) they do not overlap with antibiotic binding sites; (ii) the binding of antibiotics does not induce extensive spatial rearrangements that disturb or even destroy the metal binding pocket, either directly or via long distance conformational changes.
The question whether the three metal binding sites contain metals important for the structure or function of the ribosome can at this point be answered only in a speculative way. In the absence of high resolution models it is impossible to predict from the position of the cleavage sites the nucleotides to which the metals are coordinated. These nucleotides could be far away in the secondary structure, as was demonstrated for tRNAPhe, where the metal ion was bound to nucleotides in the T loop and the cleavage site was located in the D loop (16). Therefore, discussion of the positions of the lead cleavage sites is confined solely to well proven secondary structure models of rRNAs (35–39) and to preliminary tertiary models of 16S RNA. Furthermore, the wealth of data from biochemical, genetic and immunological experiments can be taken into account for discussion of functionally relevant regions on the rRNAs.
Both cleavages G240 in 16S and A505 in 23S rRNA are located in regions which have never been implicated in tRNA binding or in peptidyl transferase activity and might therefore be caused by metals which are important in coordinating RNA structure. In fact, both regions lie in the 5′-terminal domains of 16S and 23S RNAs, which have both been shown by their restistance to various RNases and denaturing conditions to fold into highly organized and stable structures (36,40). G240 in 16S RNA is located in a region where protein S17 shows footprints and this region lies at the bottom of the 30S subunit in all published 3-dimensional models (41–43). A505 is located in domain I of 23S RNA and L24 has been shown to bind to this region (36). As no localization of L24 by immunoelectron microscopy has been reported it could not be placed on a model of the 50S subunit (44).
On the other hand, C2347 (and C2440, at which cleavage occurs at higher lead concentrations) in 23S RNA are located in domain V and are adjacent to regions implicated in tRNA binding and peptidyl transferase action (for reviews see 4–6). In particular, L27, a protein known to be close to the peptidyl transferase centre (6), was crosslinked to a nearby region (fragment 2332–2337). Therefore, the Pb2+ ion causing cleavage at C2347 is bound in a metal binding pocket near the peptidyl transferase centre. As suggested by the competition experiment, this metal binding site could naturally harbour a Mg2+ ion. Although we cannot predict from the cleavage site where the metal ion binds, this metal ion might be a canditate for a catalytic metal ion involved in peptide bond formation. This possibility is not necessarily contradicted by the fact that ribosomes are still active after cleavage of the RNA, as cleavage might occur in a region which does not influence metal binding and hence does not inhibit potential activities. Further experiments are necessary to prove or disprove the assumptions about structurally or catalytically important metal binding sites in rRNAs.
Acknowledgements
We would like to thank S.Dorner for help with the computer work and B.Weiser for the XRNA program and R.Schroeder, K.Nierhaus and Z.Rattler for invaluable discussions and infinite patience. This work was supported by a grant (P09454-MIB) from the Österreichischer Fonds zur Förderung der wissenschaftlichen Forschung to A.B.
Comments