-
PDF
- Split View
-
Views
-
Cite
Cite
Sukumar Saha, Elder Pupo, Afshin Zariri, Peter van der Ley, Lipid A heterogeneity and its role in the host interactions with pathogenic and commensal bacteria, microLife, Volume 3, 2022, uqac011, https://doi.org/10.1093/femsml/uqac011
- Share Icon Share
Abstract
Lipopolysaccharide (LPS) is for most but not all Gram-negative bacteria an essential component of the outer leaflet of the outer membrane. LPS contributes to the integrity of the outer membrane, which acts as an effective permeability barrier to antimicrobial agents and protects against complement-mediated lysis. In commensal and pathogenic bacteria LPS interacts with pattern recognition receptors (e.g LBP, CD14, TLRs) of the innate immune system and thereby plays an important role in determining the immune response of the host. LPS molecules consist of a membrane-anchoring lipid A moiety and the surface-exposed core oligosaccharide and O-antigen polysaccharide. While the basic lipid A structure is conserved among different bacterial species, there is still a huge variation in its details, such as the number, position and chain length of the fatty acids and the decoration of the glucosamine disaccharide with phosphate, phosphoethanolamine or amino sugars. New evidence has emerged over the last few decades on how this lipid A heterogeneity confers distinct benefits to some bacteria because it allows them to modulate host responses in response to changing host environmental factors. Here we give an overview of what is known about the functional consequences of this lipid A structural heterogeneity. In addition, we also summarize new approaches for lipid A extraction, purification and analysis which have enabled analysis of its heterogeneity.
Introduction
Lipopolysaccharide (LPS) is generally considered to be an essential component of the outer membrane of Gram-negative bacteria and serves as a physical barrier, protecting the bacteria from its surroundings. However, our understanding of the importance of LPS in Gram-negative bacteria has changed considerably by the discovery of mutant strains of Neisseria meningitidis, Moraxella catarrhalis, and Acinetobacter baumannii which completely lack LPS. This indicates that the essentiality of LPS in Gram-negative bacteria is more complex and varies considerably depending on the species and strain background. While LPS may not be essential in some organisms, several studies indicated that strains lacking LPS are less virulent and more susceptible to antibiotics. The basic LPS structure, including the membrane-anchoring lipid A moiety differs considerably among different bacterial species, and these differences influence not only the outer membrane barrier function but also the immunological properties of LPS (see Fig. 1). While hexa-acylated lipid A has the ability to induce the strongest proinflammatory reaction after binding with the TLR4/MD-2 receptor, distinct lipid A structures of different bacteria can vary in their immunogenic potential and TLR4-mediated signaling capacity.
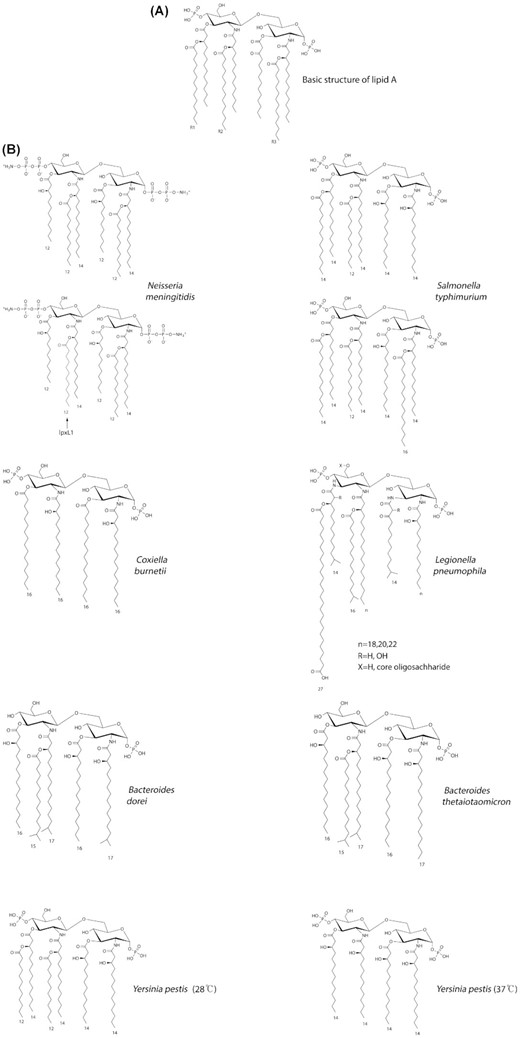
(A)The basic structure of lipid A consists of two glucosamine units, in an β (1→6) linkage, with attached acyl chains and normally containing one phosphate group on each glucosamine. The E. coli lipid A structure contains 6 acyl chains. Primary acyl chains are directly attached to the sugar moieties and usually between 10 and 16 carbons in length, secondary acyl chains are esterified with the beta-hydroxyl groups of primary acyl chains. E. coli lipid A, as an example, typically has four C14 hydroxy acyl chains attached to the sugars and one C14 and one C12 attached to the beta-hydroxy groups. Lipid A is considered the most conserved domain of Gram-negative bacterial LPS but it still shows a great degree of diversity among bacterial species. Differences are found in the number and modifications of the phosphate residues, the number and length of the acyl chains and, though less common, the chemistry of the disaccharide backbone. In E.coli, altering the phosphates, number and position of acyl chains of lipid A individually or in combination can give a wide range of TLR4/MD-2 responses and cytokine production. Modification of lipid A can also provide resistance against antimicrobial peptides by charge repulsion or decreasing of the fluidity of the outer membrane, as well as alter the activation potential of the inflammasome. The presence and length of the secondary acyl chains (R1, R2, and R3) varies between bacteria and is linked to the ability of the lipid A species to induce innate immune function. (B) Some examples showing the variation in lipid A structures from different bacteria. For N. meningitidis, both wildtype and lpxL1 mutant structures are shown; for S.typhimurium, hexa- and hepta-acylated forms are shown.
Recent studies have begun to explore LPS alterations during in vivo growth instead of lab-grown bacteria, which has been made possible by advances in mass spectrometry techniques used for analysis of complicated samples containing only small amounts of LPS. In this way, much presently hidden structural variation among strains has become apparent. It is not generally appreciated (i) how heterogeneous the TLR4/MD-2 activating lipid A part of LPS is, both within and between bacterial species, and (ii) how this can profoundly affect virulence of pathogenic bacteria, or interactions of commensal organisms with the immune system of their host. In this review, we want to discuss lipid A heterogeneity in the context of interactions of pathogenic and commensal bacteria with their hosts.
LPS deficiency
For most Gram-negative bacteria LPS is an essential component of the outer membrane. Studies with Escherichia coli which worked out the lipid A biosynthesis pathway showed that only conditional mutations are tolerated in the first steps and complete knockouts are not viable (Karow and Georgopoulos 1993). However, we reported 15 years ago how Neisseria meningitidis can survive without LPS when the lpxA gene is inactivated, which is required for the first step in the LPS biosynthesis pathway (Steeghs et al. 1998). Since that time, LPS deficiency has also been reported for some other bacterial species, i.e. Moraxella catarrhalis (lpxA), Yersinia ruckeri (lpxD) and Acinetobacter baumannii (lpxA, C.D) ( Peng et al. 2005, Henry et al. 2012, Altinok et al. 2016 ). Interestingly, in the case of A.baumannii these LPS-deficient mutants were first isolated not by directed inactivation of lpx genes but by selection for resistance against colistin (Moffatt et al. 2010). Subsequently, they were also recovered as patient isolates after colistin treatment, showing that loss of LPS can have a selective advantage under specific in vivo conditions (Cai et al. 2012, Agodi et al. 2014, Mavroidi et al. 2015). Also with N.meningitidis, an isolate from both blood and CSF of a meningitis patient has been found that lacked LPS, in this case due to a missense mutation in lpxH (Piet et al. 2014). The specific selective forces that led to its proliferation were unknown, but antibiotic selection was not involved in this case. Possibly, evasion of innate immunity mediated by TLR4 activation or specific immunity mediated by anti-LPS antibodies led to the outgrowth of this specific mutant strain.
A crucial question is why LPS is a crucial building block for the outer membrane of some bacterial species while for others it is apparently nonessential. Several studies have begun to address this point. Differences in cell envelope stress response systems have been suggested, or misassembly of major outer membrane proteins. In some cases lipid A precursors may accumulate, and different bacteria may differ in their ability to tolerate this. An lptD mutant of A.baumannii has a disruption in the LPS export pathway and accumulates the precursor lipid IVa causing a growth defect, while this is apparently not the case for an E.coli strain engineered to express only this structure (Bojkovic et al. 2016). In the case of lpxH inactivation, a diacylated glucosamine intermediate may still be formed which is toxic for A.baumannii while this is apparently not the case for N.meningitidis (Piet et al. 2014). Even closely related species such as N.meningitidis and N.gonorrhoeae differ in their tolerance for loss of LPS, as a lipid A biosynthesis block could not be introduced in the latter despite several attempts by us and others. For A.baumannii, comparative transcriptional profiling has been done on strains without LPS, showing increased expression of lipoprotein and phospholipid transport genes (Henry et al. 2012). Further, screening of multiple strains showed that only some could tolerate loss of LPS, and this was pinned down to the absence of penicillin-binding protein 1A which is involved in peptidoglycan biosynthesis (Boll et al. 2016). Also, specific lipoproteins were overexpressed as potential compensatory mechanism. Clarifying the molecular basis of such species-specific compensatory mechanisms is important for understanding basic aspects of outer membrane biogenesis, but also has practical importance as lipid A biosynthesis provides a promising target for new antibiotics. It is therefore crucial to know how widespread the ability of bacteria is to survive without LPS.
Bacteria specifically engineered for LPS deficiency can have important biotechnological uses, as they lack a major activator of innate immunity the presence of which is undesirable for many applications. In particular, LPS-deficient strains can be used for making less reactogenic inactivated whole-cell vaccines or live attenuated vaccines. However, complete loss of LPS may be disadvantageous as compared to just attenuated LPS by mutations leading to incomplete acylation, as the latter strains can be more robust and still retain some adjuvant activity. Indeed, for N.meningitidis bacterial cells without LPS had strongly reduced immunogenicity, but this was apparently not the case for M.catarrhalis and A.baumannii (Garcia-Quintanilla et al. 2014). This may be related to species differences in compensatory mechanisms, as we found loss of outer membrane lipoproteins in LPS-deficient N.meningitidis, while in A.baumannii their expression was reported to be increased instead (Steeghs et al. 2001, Henry et al. 2012). As bacterial lipoproteins are strong activators of TLR2, their increased or decreased expression can also impact on overall innate immune activation. Other applications comprise the purification of proteins, polysaccharides or other biomolecules without any endotoxin contamination. While the isolation of completely LPS-deficient E.coli has not been possible, an alternative is the use of strains engineered to make the minimal structure compatible with viability, i.e. tetra-acylated lipid A without KDO residues which lacks any detectable TLR4-activating capacity (Golenbock et al. 1991). However, it should be remembered that TLR4 activation is highly species-specific, and partial lipid A structures may still have agonistic activity in some mammalian species. When comparing human, mouse, pig and rabbit receptor activation by a panel of mutant LPS structures, we found major species differences, with human TLR4/MD-2 the most and rabbit TLR4/MD-2 the least discriminatory.
Mechanisms generating lipid A heterogeneity
Lipid A modifications can affect many physiological processes of bacteria, including structural integrity and permeability of the outer membrane, susceptibility to antimicrobial peptides, immune stimulation, formation of outer membrane vesicles and pathogenesis. There are basically two mechanisms for generating intrastrain lipid A heterogeneity: (i) regulation of gene expression and enzyme activity, and (ii) mutations in genes responsible for lipid A biosynthesis. LPS modifications due to regulation of gene expression and enzyme activity have been reviewed recently by Simpson and Trent (Simpson and Trent 2019). Neisseria meningitidis and Neisseria gonorrhoeae have been studied extensively for phenotypic variation of LPS. These bacteria can change their surface structures in response to their surroundings including the host defense system, and the resulting antigenic variability contributes to adaptation to their tissue microenvironment, distribution in the host and virulence. Clinical investigation has revealed a large repertoire of different LPS structures among meningococcal and gonococcal isolates, and mixed populations of organisms are constantly generated due to the on-off switching of LPS biosynthesis genes (Fransen et al. 2010, Ladhani et al. 2012, Rodenburg et al. 2012, du Plessis et al. 2014, Persa et al. 2014, Piet et al. 2014, Fazio et al. 2015). Exchange of genetic material by transformation and recombination also occurs frequently in N. meningitidis (Taha et al. 2002), further expanding the LPS gene repertoire.
Naturally occurring heterogeneity is also found in the lipid A produced by N. meningitidis which leads to alteration of the acylation pattern as well as modulation of endotoxic activity (van der Ley et al. 2001, Fransen et al. 2010, Brouwer et al.2011, Ladhani et al. 2012, Rodenburg et al. 2012, du Plessis et al. 2014, Persa et al. 2014, Fazio et al. 2015). Pentaacylated and tetraacylated LPS result from inactivation of the genes lpxL1 and lpxL2, respectively, and both mutants activate human TLR4 much less efficiently than wild type bacteria (van der Ley et al. 2001, Fransen et al. 2010). Meningococcal strains harbouring lpxL1 mutations occur naturally, are more likely to cause a milder and more protracted disease in older children and young adults, and are often associated with a particular genetic lineage (cc23). Such mutants may have a selective advantage over wild type strains in their survival and spreading due to their less efficient detection by the innate immune system (Ladhani et al. 2012, du Plessis et al. 2014, Fazio et al. 2015). Chronic meningococcemia is also associated with lpxL1 mutations (Brouwer et al. 2011, Persa et al. 2014). So structural heterogeneity in Neisserial lipid A exists and this heterogeneity correlates with the bioactivity of LPS.
Lipid A structure in vivo: new analytical techniques
The structure of LPS from ex vivo samples collected from animal or human bacterial infections is usually determined after extensive cultivation of bacterial specimens in vitro. The basis for this is that standard methods of structural analysis, including nuclear magnetic resonance spectroscopy and mass spectrometry (MS), commonly require much larger quantities (e.g., milligrams) of LPS than those present in samples recovered from bacterial infections. Given that bacteria can modify LPS structure in response to changes in their growth environment, this has the disadvantage that the structure of LPS obtained after bacterial multiplication in vitro may not fully reproduce that originally present in vivo. Consequently, important effects of the in vivo biological environment on LPS structure and their role in bacterial pathogenesis may be neglected.
However, the analysis of LPS directly from ex vivo extracts is difficult because these samples may typically contain low numbers of bacteria (e.g., down to 105 CFU/ml in significant lower respiratory tract and urinary infections (Khasriya et al. 2013, Gadsby et al. 2015) of correspondingly low LPS content (e.g., in the order of 102–103 pg/ml) along with abundant host-derived material. To approach this problem, micro-scale methods for extraction of low levels of LPS together with mass spectrometry techniques of increased sensitivity are being developed. In this context, two main research strategies have been adopted comprising either direct analysis of biological samples without in vitro culture or analysis of small-scale cultures of bacterial isolates with minimal passage. The rationale for the latter strategy is that by keeping bacterial passage in vitro at a minimum a less inaccurate picture of LPS structure in vivo can be obtained. For instance, it has been shown that disease-specific modification of the lipid A (e.g., addition of palmitate to the lipid A of Pseudomonas aeruginosa in clinical cystic fibrosis isolates) detected by MS analysis of the lipid A extracted from minimally passaged bacteria can be lost after multiple bacterial passages in vitro (Ernst et al.1999). Furthermore, small-scale bacterial culture provides a means to amplify and isolate bacteria from the biological matrix making samples less complex and technically less challenging than the original biological extracts. The structural analysis of LPS/lipid A from small-scale in vitro cultures of clinical isolates, as small as a few bacterial colonies, has been enabled by the introduction of sensitive MS-based micro-methods including, among others, microwave-assisted enzymatic lysis coupled to capillary electrophoresis (CE)-electrospray ionization (ESI)-MS (Dzieciatkowska et al. 2008), microwave-assisted enzymatic digestion and detergent-free mild hydrolysis in combination with matrix-assisted laser desorption ionization (MALDI) time-of-flight (TOF) MS (Zhou et al. 2009) and ammonium hydroxide/isobutyric acid micro-extraction followed by MALDI-TOF MS (Hamidi et al. ). Furthermore, it has been demonstrated that lipid A mass spectra can also be obtained by directly analyzing intact bacteria by MALDI-TOF MS in an optimized matrix solvent and this method requires only as low as 104-105 heat-inactivated bacteria for lipid A MS analyses (Larrouy-Maumus et al. 2016).
Recent studies have also started to explore lipid A alterations during in vivo growth by directly characterizing biological samples without in vitro bacterial multiplication. For example, lipid A micro-extraction by an ammonium hydroxide/isobutyric acid (El Hamidi et al. 2005) or TRI Reagent based method together with MALDI-TOF MS have been used to probe lipid A structure directly from organ tissues of mice infected with the human pathogen Klebsiella pneumoniae (Llobet et al. 2015). Lipid A mass spectra obtained from lung (∼106 CFU/gram of tissue) and spleen samples showed that K. pneumoniae is able to alter its lipid A in vivo and that this occurs in a tissue-dependent manner. Lipid A from lung isolates were found to contain a 2-hydroxyacyl modification produced by the PhoPQ-regulated oxygenase LpxO, which was not present in the lipid A recovered from spleen tissues. Remarkably, minimal passage of bacteria from lung isolates in vitro led to the loss of 2-hydroxyacyl modification of lipid A, reinforcing that environmental conditions encountered by bacteria in vivo which affect lipid A structure are not always replicated in vitro. Lipid A modification by the LpxO enzyme in vivo was found to facilitate innate immune evasion by K. pneumoniae through decreasing the activation of inflammatory responses and promoting resistance of bacteria to antimicrobial peptides (Llobet et al. 2015).
A notable improvement in the sensitivity of lipid A structural analysis has been achieved recently by the introduction of norharmane (9H-pyrido[3,4-b]indole) as matrix for lipid A analysis by MALDI-MS (Scott et al. 2016). Replacement of the standard 2,5-dihydroxybenzoic acid matrix with norharmane resulted in 10-fold enhancement in the limit of detection of lipid A by MALDI-MS enabling lipid A MS detection at the picogram level, which approximates lipid A levels in clinical samples (Scott et al. 2016). Lipid A micro-extraction by ammonium hydroxide/isobutyric acid (Hamidi et al. ) in combination with Norharmane-based MALDI-MS have been applied to the analysis of lipid A structure directly from tissues extracted from mice (host) and ticks (vector) experimentally infected with Francisella novicida. These bacteria are known to regulate lipid A structure in vitro depending on growth temperature through the preferential addition of either a 3-OH C18 acyl group at 37°C or a shorter 3-OH C16 acyl group at 18°C–25°C to lipid A by variants 1 and 2 of the lipid A-modifying N-acyltransferase enzyme LpxD (Li et al. 2012) respectively. Consistent with these in vitro observations, MS analysis of lipid A directly extracted from in vivo infected mouse spleens (∼106–107 CFU per spleen) and whole ticks (107 CFU per tick) revealed that F. novicida remodels its lipid A in vivo and incorporates a higher proportion of a long 3-OH C18 acyl group in its lipid A when growing in the higher body temperature of the mammalian host compared to the tick vector (Scott et al. 2016). Previously, incorporation of a long 3-OH C18 acyl group in the lipid A by the enzyme LpxD1 has been linked to increased antibiotic resistance and full expression of F. novicida virulence in mice (Li et al. 2012).
Lipid A structural heterogeneity and outer membrane barrier function
Structures of lipid A vary widely among different bacterial species (see Table 1). Sometimes a single bacterial species may contain more than one lipid A structure (Raetz and Whitfield 2002). In addition, specific environmental conditions can have a strong influence on the lipid A composition. Under standard growth conditions lipid A of E. coli is the biphosphorylated backbone disaccharide β-d-GlcpN4P-(1→6)-α-d-GlcpN1P, which is hexaacylated without any modifications. However, exposure to unfavourable environmental conditions including temperature, pH, excess of metal ions, presence of antimicrobial peptides, or chelating agents like EDTA can lead to profound changes in the lipid A composition (Raetz et al. 2007, Klein et al. 2009, Klein et al. 2013). It involves change in the number or composition of acyl chains, phosphate groups, or functional groups which are attached covalently (Raetz et al. 2007, Needham et al. 2013). The effect of temperature is clearly demonstrated in the case of Y. pestis: when cultured at 21°C–28°C it expresses hexaacylated lipid A structures but when the bacteria are grown at 37°C it becomes tetraacylated (Kawahara et al. 2002, Rebeil et al.2004, Knirel et al. 2005). In the case of E.coli, growth at lower temperature leads to incorporation of a longer mono-unsaturated secondary acyl chain by LpxP (Carty et al. 1999).
Lipopolysaccharides of Pathogenic Bacteria . | ||||
---|---|---|---|---|
Bacterial species . | Intra or extracellulara . | Lipid A structure . | TLR4 activation potentialb . | References . |
Escherichia coli | Intra- and extracellular | Hexa-acylated | Strong | Beutler and Rietschel 2003, Raetz and Whitfield 2002, Zähringer et al. 1994 |
Salmonella typhimurium | Facultative intracellular | Hexa and Hepta-acylated | Guo et al. 1997, Guo et al. 1998 | |
Salmonella minnesota | Facultative intracellular | Hexa-acylated, or hepta-acylated | Janusch et al. 2002, Qureshi et al. 1985 | |
Neisseria meningitidis | Facultative intracellular | Hexa- or penta- acylated | Hexa-acylated strains more strongly activate TLR4 than the penta-acylated ones | Fransen et al. 2009, Kulshin et al. 1992 |
Neisseria gonorrhoeae | Intra- and extracellular | Hexa-acylated | Post et al.2002 | |
Klebsiella pneumoniae | Mostly extracellular but can survive within the macrophages | Hexa-and hepta-acylated | Hepta-acylated form weakly activates TLR4 | Kamaladevi and Balamurugan 2016, Silipo et al.2002 |
Yersinia spp | Facultative intracellular | Tetra-acylated (37°C) and hexa-acylated (26°C) | Tetra-acylated form weakly activates TLR4 | Kawahara et al.2002, Knirel et al.2005, Montminy et al. 2006, Rebeil et al. 2004, Reinés et al. 2012 |
Proteus mirabilis | Extracellular | Hexa- or hepta- acylated | McCoy Andrea et al. 2001, Zabłotni et al. 2018 | |
Helicobacter pylori | Facultative intracellular | Tetra-acylated and hexa-acylated | Hexa-acylated form strongly activates TLR4 | Needham et al. 2013, Ogawa et al.2003, Raetz et al. 2007 |
Campylovacter jejuni | Facultative intracellular | Hexa-acylated | Weak | Korneev et al. 2018 |
Moraxella sp | Extracellular | Hepta-acylated | Masoud et al. 2011 | |
Desulfovibrio desulfuricans | Intra and extracellular | Hexa- and hepta-acylated | Wolny et al. 2011 | |
Vibrio cholerae O1 | Facultative intracellular | Hexa-acylated | Chatterjee and Chaudhuri 2003 | |
Vibrio cholerae O139 | Extracellular | Octa-acylated | Chatterjee and Chaudhuri 2003 | |
Prevotella denticola | Extracellular | Penta-acylated | Weak | Larsen et al.2015 |
Prevotella intermedia | Extracellular, some strains are intracellular | Penta-acylated | Weak | Hashimoto et al. 2003, Larsen et al. 2015 |
Porphyromonas gingivalis | Intra- and extracellular | Tetra- and penta acylated | Penta-acylated form strongly activates TLR4 | Colombo et al. 2007, Curtis et al. 2011, Kumada et al. 1995, Lee et al. 2018, Reife et al. 2006 |
Francisella spp | Facultative intracellular | Tetra-acylated | Weak | Phillips et al. 2004, Que-Gewirth et al. 2004 |
Leptospira interrogans | Intra and extracellular | Hexa-acylated | Boon Hinckley et al. 2005 | |
Legionella pneumophila | Facultative intracellular | Hexa-acylated | Weak | Shevchuk et al. 2011 |
Bordetella pertussis | Intra and extracellular | Penta- acylated | Weak | El Hamidi et al. 2009 |
Bordetella bronchiseptica | Intra- and extracellular | Hexa-cylated | Strong | Marr et al. 2010 |
Chlamydia trachomatis | Intracellular | Penta-acylated | Weak | Yang et al.2019 |
Coxiella burnettii | Intracellular | Tetra-acylated | Weak | Toman et al. 2004 |
Capnocytophaga canimorsus | Extracellular | Penta-acylated | Weak | Ittig et al. 2012 |
Brucella spp | Intracellular | Hepta-acylated | Weak | Barquero-Calvo et al. 2007 |
Burkholderia mallei | Facultative intracellular | Tetra and Penta-acylated | Weak | Korneev et al. 2015 |
Burkholderia cenocepacia | Facultative intracellular | Penta-acylated | Strong | Korneev et al. 2015 |
Burkholderia dolosa | Facultative intracellular | Tetra-acylated | Strong | Lorenzo et al. 2013 |
Acinetobacter baumannii | Facultative intracellular | Hepta-acylated | Strong | Korneev et al.2015 |
Pseudomonas aeruginosa | Facultative intracellular | Tri- tetra- penta- and Hexa-acylated (depending on environmental conditions) | Weak | Ernst et al.1999, Korneev et al.2015 |
Haemophilus influenzae | Facultative intracellular | Hexa-acylated | Weak | White et al.1999 |
Shigella flexneri | Facultative intracellular | Hexa-acylated | d'Hauteville et al. 2002 | |
Shigella sonnei | Facultative intracellular | Hexa-acylated | Bath et al. 1987 | |
Serratia marcescens | Facultative intracellular | penta-acylated | Strong | Makimura et al. 2007 |
Stenotrophomonas sp | Extracellular | Hexa-acylated | Naito et al. 2017 | |
Aeromonas sp. | Facultative intracellular | Hexa-acylated | El-Aneed and Banoub 2005 | |
Lipopolysaccharides of Commensal Bacteria | ||||
Bacterial species | Intra or extracellulara | Lipid A structure | TLR4 activation potentialb | References |
Bacteroides spp | Extracellular | Penta- and tetra-acylated | Weak | d'Hennezel et al. 2017 |
Bacteroides fragilis | Extracellular | Penta-acylated | Weak | Berezow et al. 2009, Weintraub et al. 1989 |
Bacteroides dorei | Extracellular | Penta-acylated | Weak | Vatanen et al. 2016 |
Bacteroides thetaiotaomicron | Extracellular | Penta-acylated | Weak | Berezow et al. 2009 |
Acinetobacter spp | Extracellular but can survive inside vacuoles | Hexa- and hepta- acylated | Arroyo et al.2011, Beceiro et al. 2011, Boll Joseph et al. 2015 | |
Stenotrophomonas spp | Extracellular | Hexa-acylated | Naito et al. 2017 | |
Veillonella parvula | Extracellular | Not identified yet | Weak | Matera et al.1991, Matera et al. 2009 |
Delftia spp | Extracellular | Hexa-acylated | Naito et al.2017 | |
Prevotella melaninogenica | Extracellular | Penta-acylated | Weak | Council 2013 |
Providencia rettgeri | Extracellular | Hexa-acylated | Munford 2008 | |
Klebsiella oxytoca | Extracellular | Hexa-acylated | Silipo et al. 2002 | |
Burkholderia cepacia | Facultative intracellular | Penta-acylated | Silipo et al.2005 | |
Burkholderia pseudomallei | Intracellular | Penta-acylated | Norris et al.2017 |
Lipopolysaccharides of Pathogenic Bacteria . | ||||
---|---|---|---|---|
Bacterial species . | Intra or extracellulara . | Lipid A structure . | TLR4 activation potentialb . | References . |
Escherichia coli | Intra- and extracellular | Hexa-acylated | Strong | Beutler and Rietschel 2003, Raetz and Whitfield 2002, Zähringer et al. 1994 |
Salmonella typhimurium | Facultative intracellular | Hexa and Hepta-acylated | Guo et al. 1997, Guo et al. 1998 | |
Salmonella minnesota | Facultative intracellular | Hexa-acylated, or hepta-acylated | Janusch et al. 2002, Qureshi et al. 1985 | |
Neisseria meningitidis | Facultative intracellular | Hexa- or penta- acylated | Hexa-acylated strains more strongly activate TLR4 than the penta-acylated ones | Fransen et al. 2009, Kulshin et al. 1992 |
Neisseria gonorrhoeae | Intra- and extracellular | Hexa-acylated | Post et al.2002 | |
Klebsiella pneumoniae | Mostly extracellular but can survive within the macrophages | Hexa-and hepta-acylated | Hepta-acylated form weakly activates TLR4 | Kamaladevi and Balamurugan 2016, Silipo et al.2002 |
Yersinia spp | Facultative intracellular | Tetra-acylated (37°C) and hexa-acylated (26°C) | Tetra-acylated form weakly activates TLR4 | Kawahara et al.2002, Knirel et al.2005, Montminy et al. 2006, Rebeil et al. 2004, Reinés et al. 2012 |
Proteus mirabilis | Extracellular | Hexa- or hepta- acylated | McCoy Andrea et al. 2001, Zabłotni et al. 2018 | |
Helicobacter pylori | Facultative intracellular | Tetra-acylated and hexa-acylated | Hexa-acylated form strongly activates TLR4 | Needham et al. 2013, Ogawa et al.2003, Raetz et al. 2007 |
Campylovacter jejuni | Facultative intracellular | Hexa-acylated | Weak | Korneev et al. 2018 |
Moraxella sp | Extracellular | Hepta-acylated | Masoud et al. 2011 | |
Desulfovibrio desulfuricans | Intra and extracellular | Hexa- and hepta-acylated | Wolny et al. 2011 | |
Vibrio cholerae O1 | Facultative intracellular | Hexa-acylated | Chatterjee and Chaudhuri 2003 | |
Vibrio cholerae O139 | Extracellular | Octa-acylated | Chatterjee and Chaudhuri 2003 | |
Prevotella denticola | Extracellular | Penta-acylated | Weak | Larsen et al.2015 |
Prevotella intermedia | Extracellular, some strains are intracellular | Penta-acylated | Weak | Hashimoto et al. 2003, Larsen et al. 2015 |
Porphyromonas gingivalis | Intra- and extracellular | Tetra- and penta acylated | Penta-acylated form strongly activates TLR4 | Colombo et al. 2007, Curtis et al. 2011, Kumada et al. 1995, Lee et al. 2018, Reife et al. 2006 |
Francisella spp | Facultative intracellular | Tetra-acylated | Weak | Phillips et al. 2004, Que-Gewirth et al. 2004 |
Leptospira interrogans | Intra and extracellular | Hexa-acylated | Boon Hinckley et al. 2005 | |
Legionella pneumophila | Facultative intracellular | Hexa-acylated | Weak | Shevchuk et al. 2011 |
Bordetella pertussis | Intra and extracellular | Penta- acylated | Weak | El Hamidi et al. 2009 |
Bordetella bronchiseptica | Intra- and extracellular | Hexa-cylated | Strong | Marr et al. 2010 |
Chlamydia trachomatis | Intracellular | Penta-acylated | Weak | Yang et al.2019 |
Coxiella burnettii | Intracellular | Tetra-acylated | Weak | Toman et al. 2004 |
Capnocytophaga canimorsus | Extracellular | Penta-acylated | Weak | Ittig et al. 2012 |
Brucella spp | Intracellular | Hepta-acylated | Weak | Barquero-Calvo et al. 2007 |
Burkholderia mallei | Facultative intracellular | Tetra and Penta-acylated | Weak | Korneev et al. 2015 |
Burkholderia cenocepacia | Facultative intracellular | Penta-acylated | Strong | Korneev et al. 2015 |
Burkholderia dolosa | Facultative intracellular | Tetra-acylated | Strong | Lorenzo et al. 2013 |
Acinetobacter baumannii | Facultative intracellular | Hepta-acylated | Strong | Korneev et al.2015 |
Pseudomonas aeruginosa | Facultative intracellular | Tri- tetra- penta- and Hexa-acylated (depending on environmental conditions) | Weak | Ernst et al.1999, Korneev et al.2015 |
Haemophilus influenzae | Facultative intracellular | Hexa-acylated | Weak | White et al.1999 |
Shigella flexneri | Facultative intracellular | Hexa-acylated | d'Hauteville et al. 2002 | |
Shigella sonnei | Facultative intracellular | Hexa-acylated | Bath et al. 1987 | |
Serratia marcescens | Facultative intracellular | penta-acylated | Strong | Makimura et al. 2007 |
Stenotrophomonas sp | Extracellular | Hexa-acylated | Naito et al. 2017 | |
Aeromonas sp. | Facultative intracellular | Hexa-acylated | El-Aneed and Banoub 2005 | |
Lipopolysaccharides of Commensal Bacteria | ||||
Bacterial species | Intra or extracellulara | Lipid A structure | TLR4 activation potentialb | References |
Bacteroides spp | Extracellular | Penta- and tetra-acylated | Weak | d'Hennezel et al. 2017 |
Bacteroides fragilis | Extracellular | Penta-acylated | Weak | Berezow et al. 2009, Weintraub et al. 1989 |
Bacteroides dorei | Extracellular | Penta-acylated | Weak | Vatanen et al. 2016 |
Bacteroides thetaiotaomicron | Extracellular | Penta-acylated | Weak | Berezow et al. 2009 |
Acinetobacter spp | Extracellular but can survive inside vacuoles | Hexa- and hepta- acylated | Arroyo et al.2011, Beceiro et al. 2011, Boll Joseph et al. 2015 | |
Stenotrophomonas spp | Extracellular | Hexa-acylated | Naito et al. 2017 | |
Veillonella parvula | Extracellular | Not identified yet | Weak | Matera et al.1991, Matera et al. 2009 |
Delftia spp | Extracellular | Hexa-acylated | Naito et al.2017 | |
Prevotella melaninogenica | Extracellular | Penta-acylated | Weak | Council 2013 |
Providencia rettgeri | Extracellular | Hexa-acylated | Munford 2008 | |
Klebsiella oxytoca | Extracellular | Hexa-acylated | Silipo et al. 2002 | |
Burkholderia cepacia | Facultative intracellular | Penta-acylated | Silipo et al.2005 | |
Burkholderia pseudomallei | Intracellular | Penta-acylated | Norris et al.2017 |
Intracellular bacteria: Bacteria, which have the capability to enter and survive in the cells of the host organism. Extracellular bacteria: Bacteria, which can survive and multiply outside the host cell. Facultative intracellular: They are basically extracellular but they can survive within the cells.
TLR4 activation potential is given relative to that of hexa-acylated and bis-phosphorylated LPS of E. coli.
Lipopolysaccharides of Pathogenic Bacteria . | ||||
---|---|---|---|---|
Bacterial species . | Intra or extracellulara . | Lipid A structure . | TLR4 activation potentialb . | References . |
Escherichia coli | Intra- and extracellular | Hexa-acylated | Strong | Beutler and Rietschel 2003, Raetz and Whitfield 2002, Zähringer et al. 1994 |
Salmonella typhimurium | Facultative intracellular | Hexa and Hepta-acylated | Guo et al. 1997, Guo et al. 1998 | |
Salmonella minnesota | Facultative intracellular | Hexa-acylated, or hepta-acylated | Janusch et al. 2002, Qureshi et al. 1985 | |
Neisseria meningitidis | Facultative intracellular | Hexa- or penta- acylated | Hexa-acylated strains more strongly activate TLR4 than the penta-acylated ones | Fransen et al. 2009, Kulshin et al. 1992 |
Neisseria gonorrhoeae | Intra- and extracellular | Hexa-acylated | Post et al.2002 | |
Klebsiella pneumoniae | Mostly extracellular but can survive within the macrophages | Hexa-and hepta-acylated | Hepta-acylated form weakly activates TLR4 | Kamaladevi and Balamurugan 2016, Silipo et al.2002 |
Yersinia spp | Facultative intracellular | Tetra-acylated (37°C) and hexa-acylated (26°C) | Tetra-acylated form weakly activates TLR4 | Kawahara et al.2002, Knirel et al.2005, Montminy et al. 2006, Rebeil et al. 2004, Reinés et al. 2012 |
Proteus mirabilis | Extracellular | Hexa- or hepta- acylated | McCoy Andrea et al. 2001, Zabłotni et al. 2018 | |
Helicobacter pylori | Facultative intracellular | Tetra-acylated and hexa-acylated | Hexa-acylated form strongly activates TLR4 | Needham et al. 2013, Ogawa et al.2003, Raetz et al. 2007 |
Campylovacter jejuni | Facultative intracellular | Hexa-acylated | Weak | Korneev et al. 2018 |
Moraxella sp | Extracellular | Hepta-acylated | Masoud et al. 2011 | |
Desulfovibrio desulfuricans | Intra and extracellular | Hexa- and hepta-acylated | Wolny et al. 2011 | |
Vibrio cholerae O1 | Facultative intracellular | Hexa-acylated | Chatterjee and Chaudhuri 2003 | |
Vibrio cholerae O139 | Extracellular | Octa-acylated | Chatterjee and Chaudhuri 2003 | |
Prevotella denticola | Extracellular | Penta-acylated | Weak | Larsen et al.2015 |
Prevotella intermedia | Extracellular, some strains are intracellular | Penta-acylated | Weak | Hashimoto et al. 2003, Larsen et al. 2015 |
Porphyromonas gingivalis | Intra- and extracellular | Tetra- and penta acylated | Penta-acylated form strongly activates TLR4 | Colombo et al. 2007, Curtis et al. 2011, Kumada et al. 1995, Lee et al. 2018, Reife et al. 2006 |
Francisella spp | Facultative intracellular | Tetra-acylated | Weak | Phillips et al. 2004, Que-Gewirth et al. 2004 |
Leptospira interrogans | Intra and extracellular | Hexa-acylated | Boon Hinckley et al. 2005 | |
Legionella pneumophila | Facultative intracellular | Hexa-acylated | Weak | Shevchuk et al. 2011 |
Bordetella pertussis | Intra and extracellular | Penta- acylated | Weak | El Hamidi et al. 2009 |
Bordetella bronchiseptica | Intra- and extracellular | Hexa-cylated | Strong | Marr et al. 2010 |
Chlamydia trachomatis | Intracellular | Penta-acylated | Weak | Yang et al.2019 |
Coxiella burnettii | Intracellular | Tetra-acylated | Weak | Toman et al. 2004 |
Capnocytophaga canimorsus | Extracellular | Penta-acylated | Weak | Ittig et al. 2012 |
Brucella spp | Intracellular | Hepta-acylated | Weak | Barquero-Calvo et al. 2007 |
Burkholderia mallei | Facultative intracellular | Tetra and Penta-acylated | Weak | Korneev et al. 2015 |
Burkholderia cenocepacia | Facultative intracellular | Penta-acylated | Strong | Korneev et al. 2015 |
Burkholderia dolosa | Facultative intracellular | Tetra-acylated | Strong | Lorenzo et al. 2013 |
Acinetobacter baumannii | Facultative intracellular | Hepta-acylated | Strong | Korneev et al.2015 |
Pseudomonas aeruginosa | Facultative intracellular | Tri- tetra- penta- and Hexa-acylated (depending on environmental conditions) | Weak | Ernst et al.1999, Korneev et al.2015 |
Haemophilus influenzae | Facultative intracellular | Hexa-acylated | Weak | White et al.1999 |
Shigella flexneri | Facultative intracellular | Hexa-acylated | d'Hauteville et al. 2002 | |
Shigella sonnei | Facultative intracellular | Hexa-acylated | Bath et al. 1987 | |
Serratia marcescens | Facultative intracellular | penta-acylated | Strong | Makimura et al. 2007 |
Stenotrophomonas sp | Extracellular | Hexa-acylated | Naito et al. 2017 | |
Aeromonas sp. | Facultative intracellular | Hexa-acylated | El-Aneed and Banoub 2005 | |
Lipopolysaccharides of Commensal Bacteria | ||||
Bacterial species | Intra or extracellulara | Lipid A structure | TLR4 activation potentialb | References |
Bacteroides spp | Extracellular | Penta- and tetra-acylated | Weak | d'Hennezel et al. 2017 |
Bacteroides fragilis | Extracellular | Penta-acylated | Weak | Berezow et al. 2009, Weintraub et al. 1989 |
Bacteroides dorei | Extracellular | Penta-acylated | Weak | Vatanen et al. 2016 |
Bacteroides thetaiotaomicron | Extracellular | Penta-acylated | Weak | Berezow et al. 2009 |
Acinetobacter spp | Extracellular but can survive inside vacuoles | Hexa- and hepta- acylated | Arroyo et al.2011, Beceiro et al. 2011, Boll Joseph et al. 2015 | |
Stenotrophomonas spp | Extracellular | Hexa-acylated | Naito et al. 2017 | |
Veillonella parvula | Extracellular | Not identified yet | Weak | Matera et al.1991, Matera et al. 2009 |
Delftia spp | Extracellular | Hexa-acylated | Naito et al.2017 | |
Prevotella melaninogenica | Extracellular | Penta-acylated | Weak | Council 2013 |
Providencia rettgeri | Extracellular | Hexa-acylated | Munford 2008 | |
Klebsiella oxytoca | Extracellular | Hexa-acylated | Silipo et al. 2002 | |
Burkholderia cepacia | Facultative intracellular | Penta-acylated | Silipo et al.2005 | |
Burkholderia pseudomallei | Intracellular | Penta-acylated | Norris et al.2017 |
Lipopolysaccharides of Pathogenic Bacteria . | ||||
---|---|---|---|---|
Bacterial species . | Intra or extracellulara . | Lipid A structure . | TLR4 activation potentialb . | References . |
Escherichia coli | Intra- and extracellular | Hexa-acylated | Strong | Beutler and Rietschel 2003, Raetz and Whitfield 2002, Zähringer et al. 1994 |
Salmonella typhimurium | Facultative intracellular | Hexa and Hepta-acylated | Guo et al. 1997, Guo et al. 1998 | |
Salmonella minnesota | Facultative intracellular | Hexa-acylated, or hepta-acylated | Janusch et al. 2002, Qureshi et al. 1985 | |
Neisseria meningitidis | Facultative intracellular | Hexa- or penta- acylated | Hexa-acylated strains more strongly activate TLR4 than the penta-acylated ones | Fransen et al. 2009, Kulshin et al. 1992 |
Neisseria gonorrhoeae | Intra- and extracellular | Hexa-acylated | Post et al.2002 | |
Klebsiella pneumoniae | Mostly extracellular but can survive within the macrophages | Hexa-and hepta-acylated | Hepta-acylated form weakly activates TLR4 | Kamaladevi and Balamurugan 2016, Silipo et al.2002 |
Yersinia spp | Facultative intracellular | Tetra-acylated (37°C) and hexa-acylated (26°C) | Tetra-acylated form weakly activates TLR4 | Kawahara et al.2002, Knirel et al.2005, Montminy et al. 2006, Rebeil et al. 2004, Reinés et al. 2012 |
Proteus mirabilis | Extracellular | Hexa- or hepta- acylated | McCoy Andrea et al. 2001, Zabłotni et al. 2018 | |
Helicobacter pylori | Facultative intracellular | Tetra-acylated and hexa-acylated | Hexa-acylated form strongly activates TLR4 | Needham et al. 2013, Ogawa et al.2003, Raetz et al. 2007 |
Campylovacter jejuni | Facultative intracellular | Hexa-acylated | Weak | Korneev et al. 2018 |
Moraxella sp | Extracellular | Hepta-acylated | Masoud et al. 2011 | |
Desulfovibrio desulfuricans | Intra and extracellular | Hexa- and hepta-acylated | Wolny et al. 2011 | |
Vibrio cholerae O1 | Facultative intracellular | Hexa-acylated | Chatterjee and Chaudhuri 2003 | |
Vibrio cholerae O139 | Extracellular | Octa-acylated | Chatterjee and Chaudhuri 2003 | |
Prevotella denticola | Extracellular | Penta-acylated | Weak | Larsen et al.2015 |
Prevotella intermedia | Extracellular, some strains are intracellular | Penta-acylated | Weak | Hashimoto et al. 2003, Larsen et al. 2015 |
Porphyromonas gingivalis | Intra- and extracellular | Tetra- and penta acylated | Penta-acylated form strongly activates TLR4 | Colombo et al. 2007, Curtis et al. 2011, Kumada et al. 1995, Lee et al. 2018, Reife et al. 2006 |
Francisella spp | Facultative intracellular | Tetra-acylated | Weak | Phillips et al. 2004, Que-Gewirth et al. 2004 |
Leptospira interrogans | Intra and extracellular | Hexa-acylated | Boon Hinckley et al. 2005 | |
Legionella pneumophila | Facultative intracellular | Hexa-acylated | Weak | Shevchuk et al. 2011 |
Bordetella pertussis | Intra and extracellular | Penta- acylated | Weak | El Hamidi et al. 2009 |
Bordetella bronchiseptica | Intra- and extracellular | Hexa-cylated | Strong | Marr et al. 2010 |
Chlamydia trachomatis | Intracellular | Penta-acylated | Weak | Yang et al.2019 |
Coxiella burnettii | Intracellular | Tetra-acylated | Weak | Toman et al. 2004 |
Capnocytophaga canimorsus | Extracellular | Penta-acylated | Weak | Ittig et al. 2012 |
Brucella spp | Intracellular | Hepta-acylated | Weak | Barquero-Calvo et al. 2007 |
Burkholderia mallei | Facultative intracellular | Tetra and Penta-acylated | Weak | Korneev et al. 2015 |
Burkholderia cenocepacia | Facultative intracellular | Penta-acylated | Strong | Korneev et al. 2015 |
Burkholderia dolosa | Facultative intracellular | Tetra-acylated | Strong | Lorenzo et al. 2013 |
Acinetobacter baumannii | Facultative intracellular | Hepta-acylated | Strong | Korneev et al.2015 |
Pseudomonas aeruginosa | Facultative intracellular | Tri- tetra- penta- and Hexa-acylated (depending on environmental conditions) | Weak | Ernst et al.1999, Korneev et al.2015 |
Haemophilus influenzae | Facultative intracellular | Hexa-acylated | Weak | White et al.1999 |
Shigella flexneri | Facultative intracellular | Hexa-acylated | d'Hauteville et al. 2002 | |
Shigella sonnei | Facultative intracellular | Hexa-acylated | Bath et al. 1987 | |
Serratia marcescens | Facultative intracellular | penta-acylated | Strong | Makimura et al. 2007 |
Stenotrophomonas sp | Extracellular | Hexa-acylated | Naito et al. 2017 | |
Aeromonas sp. | Facultative intracellular | Hexa-acylated | El-Aneed and Banoub 2005 | |
Lipopolysaccharides of Commensal Bacteria | ||||
Bacterial species | Intra or extracellulara | Lipid A structure | TLR4 activation potentialb | References |
Bacteroides spp | Extracellular | Penta- and tetra-acylated | Weak | d'Hennezel et al. 2017 |
Bacteroides fragilis | Extracellular | Penta-acylated | Weak | Berezow et al. 2009, Weintraub et al. 1989 |
Bacteroides dorei | Extracellular | Penta-acylated | Weak | Vatanen et al. 2016 |
Bacteroides thetaiotaomicron | Extracellular | Penta-acylated | Weak | Berezow et al. 2009 |
Acinetobacter spp | Extracellular but can survive inside vacuoles | Hexa- and hepta- acylated | Arroyo et al.2011, Beceiro et al. 2011, Boll Joseph et al. 2015 | |
Stenotrophomonas spp | Extracellular | Hexa-acylated | Naito et al. 2017 | |
Veillonella parvula | Extracellular | Not identified yet | Weak | Matera et al.1991, Matera et al. 2009 |
Delftia spp | Extracellular | Hexa-acylated | Naito et al.2017 | |
Prevotella melaninogenica | Extracellular | Penta-acylated | Weak | Council 2013 |
Providencia rettgeri | Extracellular | Hexa-acylated | Munford 2008 | |
Klebsiella oxytoca | Extracellular | Hexa-acylated | Silipo et al. 2002 | |
Burkholderia cepacia | Facultative intracellular | Penta-acylated | Silipo et al.2005 | |
Burkholderia pseudomallei | Intracellular | Penta-acylated | Norris et al.2017 |
Intracellular bacteria: Bacteria, which have the capability to enter and survive in the cells of the host organism. Extracellular bacteria: Bacteria, which can survive and multiply outside the host cell. Facultative intracellular: They are basically extracellular but they can survive within the cells.
TLR4 activation potential is given relative to that of hexa-acylated and bis-phosphorylated LPS of E. coli.
Salmonella spp overcome the action of cationic antimicrobial peptides (CAMPs) by several modifications of their lipid A, including the addition of an additional palmitoyl chain (16:0), a 2-OH group to one the secondary chains and Ara4N to the phosphates (Guo et al. 1997). In a similar way, Klebsiella pneumoniae resists the host innate immune system by addition of Ara4N to the lipid A phosphates and by switching from a potently agonistic hexa-acylated to a weak hepta-acylated lipid A (Kamaladevi and Balamurugan 2016). Some gastrointestinal pathogens, such as Helicobacter pylori and Campylobacter jejuni have a different pattern of lipid A components. Lipid A of most clinical isolates of H. pylori lacks 4′-phosphate while an ethanolamine phosphate is attached to the reducing GlcN of the lipid A, which is only tetra-acylated with longer chains of 16-18 carbon atoms. Much more diversity is observed in the lipid A of C. jejuni where the disaccharide backbone of the most common forms has both glucosamine (GlcN) and diacylglycerol (DAG). The phosphate groups are substituted with bis-phosphorylated ethanolamine or with 3 or 4 additional phosphate units and some strains express from tetra-to hexa-acylated lipid A forms (Moran et al. 1997, Stephenson et al. 2013, Korneev et al. 2018). Extreme structural diversity is reported among different strains of Vibrio cholera biotype E1 Tor which expresses hexa-acylated lipid A modified with either glycine or diglycine units attached at the 3′-position (C12:0) with a 3-OH groups (Hankins et al.2011, Henderson et al. 2017). Glycine or diglycine modification decrease the negative charge of the bacterial surface which provides resistance to cationic antimicrobial peptides such as polymyxin. On the other hand, The pandemic V.cholerae biotype Classical strain is devoid of these glycine/diglycine modifications and is polymyxin-sensitive (Henderson et al. 2017). An interesting form of lipid A micro-diversity among strains of the human gut bacterium Desulfovibrio desulfuricans has been reported by (Zhang-Sun et al. 2015). Desulfovibrio desulfuricans shares the di-phospho-di-glucosamine lipid A with Enterobacteriaceae, with also the same fatty acid distribution. However, some strains of D. desulfuricans isolated from the same host have a different lipid A structure with different pro-inflammatory properties.
High levels of lipid A heterogeneity are also present in the genera of the Bacteroidetes phylum, consisting of Bacteroides, Alistipes, Parabacteroides, and Prevotella (Wexler and Goodman 2017). Bacteroides LPS expresses a different lipid A structure compared to the prototypical Proteobacterial lipid A, since different Bacteroides species only possess penta- and tetra acylated species, containing branched fatty acids (15–17 carbon atoms in length), and the sugar backbone is substituted with only one phosphate group (Weintraub et al. 1989, Berezow et al. 2009, Vatanen et al. 2016). This lipid A modification contributes to reduced activation of the TLR4/MD-2 LPS receptor (Rietschel et al. 1998, Phillips et al. 2004, Que-Gewirth et al. 2004, Kanistanon et al. 2008, Munford 2008, Coats et al. 2009, Cullen and Trent 2010, Coats et al. 2011, Cullen et al. 2011). Broad conservation of the LpxF enzyme across commensal Bacteroidetes is responsible for removing a single lipid A phosphate residue which provides resistance to antimicrobial peptides and increases bacterial resilience in the intestine (Cullen et al. 2015).
Reports are not available on the LPS from gut Prevotella species but the lipid A and R-LPS structures have been characterized from two Prevotella species (P. denticola and P. intermedia) found in the human oral cavity (Hashimoto et al. 2003, Di Lorenzo et al. 2016). Their lipid A moieties are penta-acylated and decorated by phosphoethanolamine, and have low inflammatory capacity similar to Bacteroides (Larsen et al. 2015). Tetra- and penta acylated lipid A structures of Porphyromonas gingivalis LPS have been shown to activate the TLR4-mediated NF-κB signaling pathway differentially and modulate the expression of proinflammatory cytokines (Herath et al. 2013). Over-acylated and bi-phosphorylated LPS have been identified in P. gingivalis isolates from patients with chronic periodontitis (Herath et al. 2013, Strachan et al.2019). Zhang-Sun and his co-workers(Zhang-Sun et al. 2019) very recently characterized the LPSs of three Ralsonia species: Ralstonia eutropa, R. mannitolilytica, and R. pickettii. They showed that lipid A of R. pickettii is penta-acylated and was of low inflammatory capacity compared to the other two species having hexa-acylated lipid A. Production of outer membrane vesicles (OMVs) was increased by the under-acylated LPS containing R. pickettii and these can enter into the blood more easily than do the bacteria. Deacylation of lipid A also lead to increased production of OMVs in Salmonella enterica serovar Typhimurium (Elhenawy et al. 2016).
When considering these examples of low-activity penta-acylated lipid A, it is interesting to note that a bioinformatic analysis of available bacterial whole genome sequences showed that while the lpxL gene was found in most gram-negative bacteria, the lpxM gene was exclusively present in Gammaproteobacteria (Brix et al. 2015). Since LpxM is required for adding the sixth acyl chain, most bacteria may thus make only the less inflammatory penta-acylated form. Therefore, the typical hexa-acylated lipid A from E.coli may not be representative, and low-activity LPS forms may be much more common than generally assumed.
The permeability properties of the outer membrane barrier have a major impact on the susceptibility or resistance to antibiotics. Mutations altering the lipid or protein composition of the outer membrane can lead to the development of drug resistant bacteria (Pagès et al. 2008). LPS plays a major role in the outer membrane permeability and modifications in the lipid A structure can affect antimicrobial susceptibility of the organisms (Vaara 1992, Delcour 2009). LPS deficient mutants of E. coli having deletions in any of the genes required for the inner part of core-oligosaccharide are more susceptible towards cationic AMPs (Ebbensgaard et al. 2018) such as melittin, indolicidin, cecropin and colistin. LPS-deficient colistin resistant A. baumannii clinical isolates have been reported previously (Moffatt et al. 2010, Moffatt et al. 2013) E. coli and Salmonella typhimurium are protected by their LPS from the antimicrobial activity of medium and long chain fatty acids (Freese et al. 1973). Fatty acid resistance of meningococci largely depends on the specific composition of the LPS core oligosaccharide as well as hexa-acylation of the lipid A (Murray et al. 2001, Fisseha et al.2005, Schielke et al. 2010).
The gut microbiome, lipid A structure and the immune response
The gut microbiome, the largest ecosystem in the human body, performs a variety of important functions including immune modulation. Their capsular polysaccharides, cell envelope constituents and metabolites are the key components which influence the immune system of the host (Arnolds and Lozupone 2016, Kespohl et al. 2017). It is now well established that microbes are in constant contact with the immune system on every surface of the human body and provide training to the immune system in early life (Olszak et al. 2012). The host-microbiome interactions in the intestine induce antimicrobial responses from the epithelia including the secretion of antibacterial factors such as α-defensins, angiogenins and RegIIIc, protecting against pathogenic microbes and subsequent abnormal immune responses (Hooper et al. 2003, Cash et al. 2006). Gut microbiota play an important role in neutrophil migration and function (Ogawa et al. 2003) and also contribute to the differentiation of T cell populations into Th1, Th2, and Th17 (Kespohl et al. 2017) or into regulatory T cells (Francino 2014). The cytokines secreted from Th17 cells have significant impact on intestinal homeostasis and inflammation (Sonnenberg et al. 2011, Rossi and Bot 2013). Short-chain atty acids such as butyrate produced by Clostridia spp cause increased production of regulatory T cells in the intestine, and supplementation of these bacteria confers resistance to colitis in mice (Atarashi et al. 2011). A derivative of indole, indoxyl 3-sulphate, produced by the gut microbiota from tryptophan modulates immune functions which may confer protection from graft versus host disease (Ghimire et al. 2018). The gut epithelium is equipped with pattern recognition receptors (PRRs) which can directly sense commensal bacteria as well as pathogens in the intestine, leading to activation of proinflammatory pathways and innate immunity of the host (Macpherson et al. 2005). The PRRs recognize a variety of common bacterial structures such as LPS of the Gram-negative and lipoteichoic acid of the gram-positive cell wall.
As described above, the detailed lipid A structures differ considerably among distinct bacterial species and determine the immunological activity of LPS (Brandenburg et al. 1993, Seydel et al. 2000). For example, an LpxF-deficient mutant B. thetaiotaomicron strain was unable to stably colonize the murine gut, thus confirming the need for lipid A dephosphorylation in colonization of the intestine (Cullen et al. 2015). This new finding shows that variation in the lipid A of species such as Bacteroides influences the commensal composition in the host gut. Another study by Cullen and colleagues showed that modification of surface structures with phosphoethanolamine (PEA) by the transferase EptC is key for promoting commensalism of the bacterium Campylobacter jejuni in its avian host (Cullen et al. 2013). EptC adds PEA to the lipid A, but also modifies the flagellar rod protein and several N-linked glycans (Cullen et al. 2013). Thus, the exact cause of the reduced colonization by the EptC-deficient mutant strain is difficult to pinpoint. The addition of PEA to the lipid A increased its ability to activate TLR4/MD-2, but at the same time provided resistance to cationic antimicrobial peptides, which indicates that in this case resisting antimicrobial peptides is more important than reducing the pro-inflammatory response.
The role of LPS in the gut system has been studied in some pathological conditions such as inflammatory bowel disease and enterocolitis. The LPS concentration in the blood of such patients was estimated to be more than 40%–60% higher as compared to healthy individuals (Pastor Rojo et al. 2007). Inflammatory balance, cell death, and intestinal permeability depend on the shifts in the microbiota during infection, disease and trauma through compositional changes in the resident LPS population. Serotype specific LPS responses may be responsible for the pathogenesis of IBD and other chronic inflammatory diseases in the intestine (Stephens and von der Weid 2019). It is plausible that the intact intestinal epithelium prevents the entry of too much LPS into the systemic circulation in healthy individuals while in diseased states such barrier functions are disrupted, allowing absorption of much higher levels of LPS into the circulation leading to endotoxemia (Pastor Rojo et al. 2007). But also under non-pathological conditions, small amounts of LPS can be absorbed from the intestinal tract and can be detected at low concentrations (1–200 pg/mL) in the blood plasma of healthy individuals (Laugerette et al. 2011). The impact of such low LPS concentrations in the circulation remains undefined. It is well established that low-activity lipid A from some bacteria can interfere with appropriate TLR4/MD-2 signaling via competitive inhibition (Curtis et al. 2011, Tan et al. 2015). Interestingly, d'Hennezel and his co-workers(d'Hennezel et al. 2017) reported that members of Bacteroidetes group are the major contributors of LPS in human gut and their LPS is non-stimulatory and inhibits TLR4-dependent cytokine production. Purified capsular polysaccharide from B. fragilis suppresses the production of IL-17 and reduces inflammation in the colon by modulating CD4 lymphocytes to produce IL-10 (Mazmanian et al. 2008). These results underline the important role of gut commensal bacteria in the management of inflammation.
A growing body of evidence suggests that the gut microbiome may be a key factor in influencing predisposition to autoimmunity, inflammatory disorders and allergic diseases ( Eppinga et al. 2014, Fyhrquist et al. 2014, Scher et al. 2015, Forbes et al. 2016, Zákostelská et al. 2016). On this note, the early childhood exposure in priming and establishing immune responses seems to be key. This was demonstrated when oral inoculation of Clostridium in early life resulted in resistance to induced gut inflammation in adult mice (Atarashi et al. 2011). More recently, a study followed the gut microbiota in the first three years of life of infants from Northern Europe (Finland and Estonia) where early-onset of autoimmune diseases are common and Russia where it is less prevalent, and found low amounts of Bacteroides species in Russian infants while they were dominant in the Finnish and Estonian infants (Vatanen et al. 2016). This translated to exposure to an underacylated lipid A structure, which in turn inhibits the innate immune signaling and prevents the induction of endotoxin tolerance (Vatanen et al. 2016). This induction of immune tolerance by exposure to highly potent LPS from E. coli showed a decrease in the incidence of diabetes in mice, whereas the same could not be observed with mice treated with underacyated LPS from B. dorei (Vatanen et al. 2016). Altogether, these studies highlight that it is not merely the presence of commensal species or the amount of LPS present in the gut, but also the specific lipid A structure they make which has a major role in the development of inflammatory diseases, autoimmunity and allergies. In addition the exposure to highly potent lipid A structures in early years of life is important for the induction of regulatory T cells and immune tolerance, thereby preventing the onset of these immunological disorders later in life.
Immune mechanisms involved in intestinal commensalism and the role of LPS related to gut health and disease need to be investigated further, considering the vast number of different gram-negative bacterial species in the gut. Untill now, isolation of LPS from gut microbiota involved conventional methods and the data generated are mostly derived from bacteria grown in vitro. As described above, their lipid A structures may differ considerably when they are present in the human gut. So it is essential to develop research methods including innovative mass spectrometry analyses of highly complex in vivo samples to investigate to role of gut microbial LPS under natural conditions and preferably in both diseased and healthy situations in order to validate the present concepts (Cani 2018).
Biotechnological applications
The great range of possible lipid A structural modifications can be utilized for vaccine development. The innate immunity activation capacity of LPS makes it a potent adjuvant, either as a component of LPS-containing vaccines based on whole bacterial cells or outer membrane vesicles (OMVs), or when added as purified component to subunit vaccines. Fine-tuning the biological activity of LPS can be done by utilizing the natural heterogeneity of lipid A and selecting those with an optimal balance between immunostimulatory and toxic properties. In addition, the great natural variety of lipid A biosynthetic and modifying enzymes can be put to use for the generation of novel variants with improved adjuvant properties. A combinatorial lipid A bioengineering approach has been used to generate extensive panels of such LPS derivatives in Escherichia coli (Needham et al. 2013) and Neisseria meningitidis (Zariri et al. 2016). In Bordetella pertussis, heterologous expression of lipid A biosynthesis enzymes enabled the depletion of endotoxic activity of vaccines based on whole cells or OMVs (Geurtsen et al. 2006, Asensio et al. 2011, Arenas et al. 2020). Complete genetic detoxification of LPS in E.coli led to the development of endotoxin-free production strains for recombinant proteins (Mamat et al. 2015). For studies of the role of the microbiome in health and disease, and possible therapeutic modifications to its composition, it will be important to take the lipid A structures of the relevant bacteria into account, for instance through MS structural analysis. Advances in new analytical techniques (Pupo et al. 2021) will make this increasingly possible, but have until now not been used to their full potential.
ACKNOWLEDGEMENTS
We would like to extend our thanks to Dr. Md. Abdul Hannan, Department of Biochemistry and Molecular Biology, Bangladesh Agricultural University, Mymensingh, Bangladesh, for helping us in preparing the figure for this manuscript.
Conflict of interest statement
The authors declare no conflicts of interest.
Funding
This work was financially supported by a Marie Curie fellowship of the European Commission (project number 796009).
References