-
PDF
- Split View
-
Views
-
Cite
Cite
Franz G Zingl, Deborah R Leitner, Himadri B Thapa, Stefan Schild, Outer membrane vesicles as versatile tools for therapeutic approaches, microLife, Volume 2, 2021, uqab006, https://doi.org/10.1093/femsml/uqab006
- Share Icon Share
ABSTRACT
Budding of the bacterial surface results in the formation and secretion of outer membrane vesicles, which is a conserved phenomenon observed in Gram-negative bacteria. Recent studies highlight that these sphere-shaped facsimiles of the donor bacterium's surface with enclosed periplasmic content may serve multiple purposes for their host bacterium. These include inter- and intraspecies cell–cell communication, effector delivery to target cells and bacterial adaptation strategies. This review provides a concise overview of potential medical applications to exploit outer membrane vesicles for therapeutic approaches. Due to the fact that outer membrane vesicles resemble the surface of their donor cells, they represent interesting nonliving candidates for vaccine development. Furthermore, bacterial donor species can be genetically engineered to display various proteins and glycans of interest on the outer membrane vesicle surface or in their lumen. Outer membrane vesicles also possess valuable bioreactor features as they have the natural capacity to protect, stabilize and enhance the activity of luminal enzymes. Along these features, outer membrane vesicles not only might be suitable for biotechnological applications but may also enable cell-specific delivery of designed therapeutics as they are efficiently internalized by nonprofessional phagocytes. Finally, outer membrane vesicles are potent modulators of our immune system with pro- and anti-inflammatory properties. A deeper understanding of immunoregulatory effects provoked by different outer membrane vesicles is the basis for their possible future applications ranging from inflammation and immune response modulation to anticancer therapy.
Outer membrane vesicles (OMVs), which typically range from 20 to 400 nm in diameter, are naturally secreted nonliving facsimiles of their donor cells. OMV production seems to be a common feature of all Gram-negative bacteria. Although several mutations cause reduced vesiculation levels compared with the parental wild-type strain (McBroom et al. 2006; Schwechheimer and Kuehn 2013; Schwechheimer, Kulp and Kuehn 2014), to our best knowledge no mutant with abolished OMV release has been reported so far. Thus, shedding of OMVs seems to be a beneficial feature of Gram-negative bacteria. Given the energy cost for replacing the surface and periplasmic components exported via the vesicles, it can be hypothesized that OMV formation provides an advantage for the donor cells and was therefore evolutionarily maintained. Indeed, multiple functions and involvement in diverse physiological processes have been reported for OMVs, including inter- and intraspecies communication, nutrient-scavenging mechanisms, biofilm formation, defense against phages and antimicrobial agents, pathogenesis, bacterial stress response and faster adaption to new environments (Nevot et al. 2006; Song et al. 2008; Kulkarni and Jagannadham 2014; Kulkarni, Nagaraj and Jagannadham 2015; Schwechheimer and Kuehn 2015; Reyes-Robles et al. 2018; Cooke et al. 2019; Zingl et al. 2020). In summary, OMVs seem suitable delivery vehicles for a variety of biomolecules. Currently, OMVs represent the only mechanism of Gram-negative bacteria for secretion and subsequent delivery of hydrophobic compounds to target cells at high concentrations. Moreover, experimental evidence indicates that luminal as well as surface-associated proteins of OMVs are fairly protected from proteases, extending the half-life of proteinaceous effectors in proteolytic environments (Kesty and Kuehn 2004). OMVs have been therefore suggested as a new secretion system type zero (Guerrero-Mandujano et al. 2017).
Notably, OMVs are loaded with microbe-associated molecular patterns (MAMPs), such as lipopolysaccharides (LPSs), peptidoglycans, lipoproteins, proteins and nucleic acids, which are recognized by the immune system and allow them to interact with host cells and modulate the immune system. MAMPs on OMVs act as effectors, making them capable of manipulating the immune cascades in the host. This review focuses on three key features of OMVs offering potential therapeutical applications: (i) alteration of the host immune response, (ii) stimulating the immune system as potential vaccine candidates and (iii) delivery and/or protection of their cargo (Fig. 1).
OMVS AS MODULATORS OF THE INNATE IMMUNE RESPONSE
OMVs can act as potent modulators of inflammatory responses in a wide range of host tissues, including the mucosal epithelial surfaces representing the first line of defense of our body (Fig. 1). The recognition of the OMV-associated MAMPs depends on specific host pattern recognition receptors (PRRs), which are present at the cell surface, in the endosomes or in the cytoplasm. Toll-like receptors (TLRs), nucleotide-binding oligomerization domain-like receptors, C-type lectin receptors and RIG-1-like receptors comprise the four families of PRRs. MAMP recognition by the host PRRs activates several host cell signaling cascades, thereby inducing expression of cell signaling molecules and antimicrobial peptides. Ismail and coworkers reported one of the first studies with regard to immunostimulatory capabilities of OMVs demonstrating a dose-dependent induction of a pro-inflammatory chemokine interleukin-8 (IL-8) upon stimulation of human gastric epithelial cells with OMVs derived from Helicobacter pylori (Ismail, Hampton and Keenan 2003). OMVs of Neisseria gonorrhoeae,Pseudomonas aeruginosa and H. pylori were shown to mediate a PRR response facilitating bacterial clearance and production of the antimicrobial peptide human β-defensin in human epithelial cells (Kaparakis et al. 2010). Moreover, several reports highlight the recognition of OMV-associated MAMPs by TLRs, representing membrane-spanning receptors with a cytoplasmic Toll/IL-1 receptor homology domain for downstream signaling (Jin and Lee 2008).
The activation of the TLRs is linked to the activation of transcription factors such as NF-κB (nuclear factor 'kappa-light-chain-enhancer' of activated B-cells) and MAP (mitogen-activated protein) kinase and to the release of pro-inflammatory cytokines, chemokines and type I interferons. For example, TLR3 and TLR7, which recognize dsRNA and ssRNA, have been shown to be stimulated by OMVs decorated with viral and parasite antigens (Fitzgerald and Kagan 2020; Yarovinsky et al. 2005). However, TLR4 is by far the most extensively studied member with regard to OMV signaling, as it interacts with lipid A, the hydrophobic membrane anchor and endotoxic part of the LPS (Poltorak et al. 1998; Hoshino et al. 1999; Park et al. 2009; Kawai and Akira 2010). OMVs derived from diverse bacteria, such as Escherichia coli, P. aeruginosa and Fusobacterium nucleatum, can activate the TLR4 cascade in various host cells resulting in activation of downstream NF-κB pathways and inflammatory cytokine release (Soderblom et al. 2005; Zhao et al. 2013; Engevik et al. 2021). Not surprisingly, several studies have shown that alteration of the lipid A moiety can massively alter the immune response. For example, OMVs containing underacylated LPS via deletion of lipid A acyltransferases, e.g. HtrB, MsbB or PagP, in donor strains, such as Shigellassp., Vibrio cholerae, enterotoxigenic E. coli (ETEC) and Salmonellassp., have been shown to reduce TLR4 stimulation and were therefore applied to OMV-based vaccine development to enhance tolerability (Rossi et al. 2014, 2016; Leitner et al. 2015). In case of the OMV-based Neisseria meningitidis vaccines, reduction of the LPS content was achieved by detergent extraction and resulted in less TLR4 activation (Fredriksen et al. 1991; Oster et al. 2005).
While TLR4 is essential for recognition of extracellular LPS, recent reports unraveled that intracellular cytosolic LPS can be recognized in a TLR4-independent manner via noncanonical caspase-dependent inflammasome activation (Hagar et al. 2013; Kayagaki et al. 2013). Importantly, OMVs are readily internalized by a variety of host cells and can function as transport vehicle for LPS (O'Donoghue and Krachler 2016). Indeed, OMVs derived from E. coli trigger cytosolic caspase activation resulting in pyroptosis and pro-inflammatory cytokine release (Vanaja et al. 2016). Moreover, OMVs of Bordetella pertussis have been recently shown to activate the noncanonical inflammasome pathway in murine macrophages, which is most likely triggered by the lipooligosaccharide component (Elizagaray et al. 2020). In contrast to the strict specificity of TLR4 for hexa-acylated lipid A, the caspase activation seems to be more relaxed allowing recognition of diverse lipid A variants with regard to the acylation pattern, but the precise molecular mechanism and structural requirements for the inflammatory caspase activation are not yet fully understood (Zamyatina and Heine 2020).
In addition, internalized OMVs can be recognized by cytoplasmic PRRs such as NOD1 and NOD2 resulting in phosphorylation of kinase signaling proteins, NF-κB activation and release of cell signaling proteins like cytokines and chemokines. These cytosolic receptors are recruited to the cytosolic compartment of the host cells after detection of bacterial peptidoglycan (Girardin et al. 2003). Peptidoglycans being a cell wall component of both Gram-positive and -negative bacteria are found to be enriched in OMVs. Studies performed with OMVs of H. pylori,P. aeruginosa and N. gonorrhoeae showed that the intracellular trafficking of peptidoglycan was essential for NOD1-mediated inflammatory response in nonphagocytic cells (Kaparakis et al. 2010). In addition, OMVs from V. cholerae non-O1 and non-O139, causing gastroenteritis and extraintestinal infections rather than cholera, have shown to elicit NOD1- and NOD2-dependent immune responses (Bielig et al. 2011). Apart from its sensory function, NOD receptor also contributes in maintaining intestinal homeostasis and microbiota balance (Rehman et al. 2011; Philpott et al. 2014). In this context, OMV-mediated stimulation of the NOD receptors can be seen as a mechanism by which the gut microbiota modulates the immune response.
Once OMVs have breached the epithelial barrier, they can also interact with various immune cells such as neutrophils, macrophages and dendritic cells in the host submucosa. For example, OMVs from N. meningitidis stimulate neutrophils resulting in the production of pro-inflammatory cytokines and chemokines [tumor necrosis factor alpha (TNF-α), IL-1β, IL-8, macrophage inflammatory proteins MIP-1α and MIP-1β] (Lapinet et al. 2000). On the contrary, OMV-mediated delivery of cytotoxic necrotizing factor type 1 (CNF1) toxin from uropathogenic E. coli impaired the antimicrobial and chemotaxis ability of neutrophils (Davis et al. 2006). OMVs are also recognized by human monocytes and macrophages resulting in the production of various pro-inflammatory cytokines and chemokines, e.g. reported for N. meningitidis,H. pylori or Salmonella ssp. (Alaniz et al. 2007; Tavano et al. 2009; Winter et al. 2014). Overall, the high abundance of MAMPs in OMVs can result in strong inflammatory responses by macrophages and monocytes, thereby exacerbating disease progression as shown for Porphyromonas gingivalis and N. meningitidis (Mirlashari et al. 2001; Ellis, Leiman and Kuehn 2010; Imayoshi, Cho and Kaminishi 2011). OMVs also interact with dendritic cells, which play a key role in the initiation of the primary immune response as they are the only antigen-presenting cell type capable of stimulating naïve T cells (Howard et al. 2004). OMVs from N. meningitidis induce the upregulation of MHC (major histocompatibility complex) class II molecules, costimulatory molecules such as CD40, CD80 and CD86, and the secretion of pro-inflammatory cytokines and chemokines in dendritic cells, resulting in a potent humoral immune response and maturation of dendritic cells (Durand et al. 2009). Additionally, OMVs can modulate immune cell signaling. For example, N. meningitidis OMVs were internalized by dendritic cells via binding to the host immune factor bactericidal permeability-increasing protein. The subsequent secretion of pro-inflammatory cytokines and induction of costimulatory molecules suggest a role of N. meningitidis OMVs in dendritic cell maturation (Schultz et al. 2007). Similarly, Cai et al. demonstrated that Acinetobacter baumannii OMVs activated bone marrow dendritic cells and promoted Th2 and humoral immune response (Cai et al. 2019). The potent immune cell stimulation by OMVs can be a beneficial feature along with their use as vaccine candidates providing adjuvant activity to enhance antibody and T-cell responses.
The cargo of OMVs, like endotoxins or RNA, is also able to modulate the host immune system. For example, the heat-labile enterotoxin (LT) from ETEC can be secreted in OMVs as well as in a free soluble form. Notably, activator protein 1 is essential for TNF-α and IL-6 activation by soluble LT, while OMV-associated LT induced TNF-α and IL-6 via an alternative pathway through NF-κB (Chutkan and Kuehn 2011). Furthermore, Vidakovics et al. showed that the OMVs derived from the respiratory pathogen Moraxella catarrhalis contain the Moraxella immunoglobulin D (IgD) binding protein (MID) and bacterial CpG-DNA motifs (Schaar et al. 2011). These OMVs induced a T-cell-independent B cell activation, via binding of MID to the IgD B cell receptor stimulating PRR signaling in B cells. In addition, MID-containing OMVs also activated lymphocytes in the tonsils resulting in IL-6 and IgM production (Vidakovics et al. 2010). In contrast, intracellular small RNAs (sRNAs) found in OMVs secreted by P. aeruginosa have been shown to downregulate the host immune response. The LPS-depleted sRNA-containing OMVs reduced IL-8 secretion in human airway epithelial cells and mouse lungs (Koeppen et al. 2016). The authors suggested that sRNA in OMVs might target cellular mRNA, thereby reducing IL-8 secretion, neutrophil recruitment and immune cells phagocyting bacteria (Koeppen et al. 2016). In another study, Choi et al. detected microRNA-sized small RNA (msRNA) in OMVs from Aggregatibacter actinomycetemcomitans,P. gingivalis and Treponema denticola (Choi et al. 2017). The OMV-associated msRNAs were able to suppress expression of several cytokines emphasizing the host–OMV interaction potential in periodontal disease (Choi et al. 2017).
Aside from their inflammatory properties, OMVs can also facilitate maintenance of gut homeostasis and activate antimicrobial defense mechanisms. For example, OMVs of N. gonorrhoeae, P. aeruginosa and H. pylori were shown to mediate a PRR response facilitating bacterial clearance and production of the antimicrobial peptide human β-defensin in human epithelial cells (Kaparakis et al. 2010). OMVs derived from Bacteroides fragilis and Akkermansia muciniphila induced anti-inflammatory responses and prevented gut inflammation in an experimental murine colitis model (Shen et al. 2012; Kang et al. 2013). In case of B. fragilis OMVs, the beneficial effect was pinpointed to the immunoregulatory capsular polysaccharide A, which is recognized by TLR2 in host dendritic cells promoting anti-inflammatory cytokine production and regulatory T-cell development (Shen et al. 2012). Moreover, OMVs from A. muciniphila also improved the intestinal barrier integrity in a high-fat diet mouse model outlining their role in the regulation of gut permeability (Chelakkot et al. 2018). Escherichia coli Nissle 1917 is probably the best-studied probiotic species among Gram-negative bacteria and has been associated with anti-inflammatory properties, epithelial barrier restauration, inhibition of epithelial invasion by pathogens and direct antagonistic effects to pathogenic bacteria (Sonnenborn 2016). It is becoming evident that OMVs derived from probiotic E. coli Nissle 1917 contribute to these beneficial features. For example, E. coli Nissle 1917 OMVs upregulate tight junction proteins (ZO-1 and claudin-14) strengthening the epithelial barrier integrity (Alvarez et al. 2016). OMVs from E. coli Nissle 1917 as well as the commensal ECOR12 also constantly activate NOD1 receptors, which suggests that OMVs derived from the gut microbiota trigger a controlled inflammatory response, which contributes to pathogen eradication and gut homeostasis (Canas et al. 2018). Moreover, OMV derived from the gut commensal Bacteroides thetaiotaomicron deliver BtMinpp, a bacterial homolog of eukaryotic multiple inositol polyphosphate phosphatase 1 (MINPP1), into host cells (Stentz et al. 2014). BtMinpp seems to have beneficial effects for both the host and the bacterial community by providing nutrients and by removing potentially carcinogenic metabolites from the gut (Stentz et al. 2014). These observations emphasize that OMVs can have a dual role of promoting inflammation and facilitating gut homeostasis.
OMVS AS VACCINE CANDIDATES
The emergence of multidrug-resistant bacteria has highlighted the need to develop alternative strategies to combat bacterial infections. Among them are preventive measures like vaccines, which have the potential to reduce both the disease burden and the antibiotic use. A recent development of vaccine candidates included the use of OMVs. The fact that they closely resemble the surface of the donor bacterium make them interesting vaccine candidates. Although OMVs contain multiple surface-exposed antigens in their native confirmation, they are nonreplicating avoiding additional inactivation steps, which are applied to whole-cell killed vaccines. Hence, OMVs are highly immunogenic and combine native antigen presentation with proper adjuvant properties (Fig. 1). Moreover, genetic modification of the OMV-producing bacteria allows the improvement of the OMV yield as well as the enhancement of their immunostimulatory and safety profile (van der Pol, Stork and van der Ley 2015). As a result, OMVs have been studied as promising vaccine candidates for various diseases caused by Gram-negative pathogens (Micoli and MacLennan 2020).
Notably, vesicle formation is not restricted to Gram-negative bacteria. Thus, Gram-positive bacteria also release membrane vesicles, which have been studied as vaccine candidates. Among others, vesicles of Clostridium perfringens,Streptococcus pneumoniae,Bacillus anthracis,Mycobacterium tuberculosis and Staphylococcus aureus have been shown to elicit immune responses in mouse models and increase survival upon lethal challenge (Rivera et al. 2010; Jiang et al. 2014; Olaya-Abril et al. 2014; Athman et al. 2015; Choi et al. 2015; Wang et al. 2018). As this review focuses on OMVs derived from Gram-negative bacteria, we will not comprehensively discuss membrane vesicles of Gram-positive bacteria, but kindly refer to recent reviews on that subject for detailed information (Liu et al. 2018; Bose et al. 2020; Briaud and Carroll 2020; Caruana and Walper 2020).
Thus far, the most successful approaches are the OMV-based vaccines against N. meningitidis, which are licensed for human use and were successfully employed to control the meningococcal group B epidemic outbreaks in Cuba (VA-MENGOC-BC®, The Finlay Institute, Havana, Cuba), Norway (MenBVac®, Norwegian Institute of Public Health, Oslo, Norway) and New Zealand (MeNZB®, Chiron Vaccines, Auckland, New Zealand) (Holst et al. 2009, 2013). Furthermore, MenBVac was also successfully used to control an outbreak of N. meningitidis in the Normandy, France (Caron et al. 2011; Sevestre et al. 2017). To decrease endotoxin activity, LPS was removed from OMVs via detergent extraction, leaving the highly variable PorA protein as the immunodominant antigen (Pizza, Bekkat-Berkani and Rappuoli 2020). Although these vaccines were highly effective, they were only designed for protection against clonal outbreaks and the induction of a strain-specific immune response limited their broad application. To generate a vaccine against multiple meningococcal B strains, further research based on reverse vaccinology was utilized. This approach resulted in a multicomponent meningococcal B vaccine registered as Bexsero® (Novartis). It combines three surface-exposed recombinant proteins added to OMVs from the epidemic outbreak in New Zealand and has already been approved for the market (Serruto et al. 2012).
Interestingly, recent epidemiological data including a retrospective case-control study have shown that OMV-based meningococcal vaccines can result in partial protection against gonococcal disease (Petousis-Harris et al. 2017; Petousis-Harris 2018). The observed cross-protection may be based on the high DNA homology of the two closely related species N. meningitidis and N. gonorrhoeae resulting in antibody cross-reactivity against meningococcal and gonococcal antigens (Tinsley and Nassif 1996). A recent study by Leduc and coworkers investigated this hypothesis in a well-characterized female mouse model of N. gonorrhoeae genital tract infection (Leduc et al. 2020). They demonstrated that vaccination of female mice with Bexsero (contains MeNZB OMVs) induces antibodies that recognize N. gonorrhoeae outer membrane proteins including several promising vaccine targets. This is in line with the study of Semchenko et al., wherein vaccination with Bexsero elicited antibodies to N. gonorrhoeae in rabbits and humans (Semchenko et al. 2019). These findings are consistent with the epidemiological data and suggest that a similar OMV-based vaccine could be a possible strategy to combat gonorrhea.
It should be mentioned that several other OMV-based vaccines are currently being developed for human and veterinary use. Strategies to combat Salmonella enterica infections in humans also include the elimination of the main source of infection, which is contaminated poultry products. A combination of a recombinant-produced S. enterica outer membrane protein F and extracted OMVs was shown to induce a strong antibody response in chicken and also rapidly decreased the bacterial load of the animals (Li et al. 2020a).
OMVs have also been successfully decorated with antigens of viral pathogens, such as the influenza A virus (H1N1) and MERS-CoV, which could extend the application of OMV-based vaccines to combat viral infections (Shehata et al. 2019). Recently, a OMV-based vaccine candidate against SARS-CoV-2 using OMVs from N. meningitidis as platform has been reported to induce a strong immune response in mice (Gaspar, Prudencio and De Gaspari 2021). Moreover, OMV-based vaccines against SARS-CoV-2 and Lyme disease are currently tested in preclincal trials (Intravacc 2021a,b).
As previously mentioned, LPS is a potent activator of TLR4, which contributes to vaccine reactogenicity. Thus, the reduction of the endotoxicity of an OMV-based vaccine is essential for a safe application in humans. In the case of the abovementioned MenB vaccines, this has been achieved by an additional detergent extraction step, which removes most of the LPS content but promotes aggregation (van de Waterbeemd et al. 2010). Furthermore, this approach alters the OMV composition through contamination of cytoplasmic proteins as well as removal of other membrane-located components, which may contribute to the OMV vaccines’ adjuvant and immunogenic profile (van de Waterbeemd et al. 2010, 2013a; Zariri et al. 2016). Thus, the absence of numerous potential antigens may require the use of additional adjuvants (Zariri et al. 2016; Gnopo et al. 2017). Due to these drawbacks, further research focused on the use of N. meningitidis strains with genetically modified LPS. These studies demonstrated that deletion of the lipid A acyltransferases, i.e.LpxL1 and LpxL2, resulted in less endotoxic OMVs (van der Ley et al. 2001). Safety and immunogenicity of genetically detoxified N. meningitidis OMVs have been successfully assessed in phase 1 studies (Keiser et al. 2010, 2011).
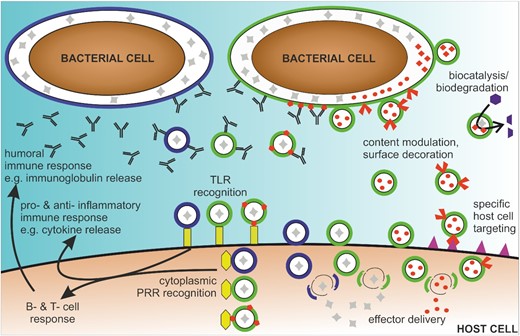
Overview of the therapeutic potential of OMVs. OMVs are released from bacterial cells (green or blue) with natural luminal content (gray). OMVs are recognized by host cell receptors (yellow), i.e. extracellularly via TLRs and in the cytosol after internalization via PRRs, resulting in inflammatory and humoral immune responses. Thus, OMVs could be used to specifically modulate pro- and anti-inflammatory responses. Moreover, OMVs can be used as vaccine candidates derived from an individual bacterial species (green or blue OMVs), derived from multiple species and used as mixtures (green and blue OMVs) or derived from genetically engineered to display heterologously expressed antigens (green OMVs with heterologously expressed antigens in red). Genetic engineering of donor bacterial cells also allows to modulate the composition of OMVs to decorate or load OMVs with heterologously expressed biomolecules (red components in or on OMVs). Applications of such bioengineered OMVs range from biocatalysis and biodegradation to delivery of natural and heterologously effectors to host cells, including targeting of specific host cell types via defined receptor interactions (purple).
Several studies have tried to optimize and standardize the manufacturing process of OMVs. One example is the production process developed for a Shigella sonnei OMV-based vaccine based on two filtration steps and genetically modified donor strains to increase the yield (deletion of tolR), removal of nicotinic acid auxotrophy (insertion of E. coli nadAB in virG) and reduction of endotoxicity (deletion of htrB) (Gerke et al. 2015). As such modifications are widely applicable to many Gram-negative bacteria that have been named ‘Generalized Modules for Membrane Antigens’ (GMMA) (Berlanda Scorza et al. 2012). Overall, this technology resulted in a GMMA-based S. sonnei vaccine candidate 1790GAHB, which has been successfully tested in animal and human trails (Gerke et al. 2015; Launay et al. 2017). Intramuscular administration of the vaccine candidate in healthy adult volunteers was well tolerated and induced antibody production against the O-antigen of S. sonnei, representing the dominant protective antigen (Launay et al. 2017). The vaccine candidate was also tested in a phase 2a clinical study in Kenya, a Shigella-endemic country, and was well tolerated with high immunogenic potency in African adults (Obiero et al. 2017). These promising results highlight the potential of genetically detoxified OMVs as vaccine candidates to combat bacterial diseases.
Aside from spontaneously released natural OMVs, bacterial vesicles can also be generated by cell lysis, e.g. using osmotic shock, physical shearing or detergents. As the yield of natural OMV production is considered to be a bottleneck along the upscaling process for vaccine production, it might be relevant to compare the protective efficacy of differentially produced vesicles. Using A. baumannii as model organism, Li and coworkers compared the yield, composition and protective properties of naturally released OMVs as well as vesicles induced by mechanical shearing or sucrose extraction (Li et al. 2020c). Interestingly, sucrose-extracted OMVs induced the highest protective immunity, which indicates the potential for alternative vesicle preparation strategies with increased yield and high protective efficacy for other OMV-based vaccine candidates. Besides higher yield, treatment of bacterial cultures with sublethal amounts of antimicrobial peptides can result in bacterial vesicles with reduced pro-inflammatory potency. Balhuizen and coworkers could show that sub-bactericidal concentrations of the cathelicidin PMAP-36 not only increase OMV production in Bordetella bronchiseptica but also are enclosed in the released OMVs and thereby attenuate undesired inflammatory responses, most likely via LPS neutralization (Balhuizen et al. 2021).
Mixtures of OMVs derived from multiple strains or even various organisms can be a promising candidate for a broad-spectrum vaccine. This approach has been already exploited in several studies. For example, Tunheim and coworkers reported that a combination of OMVs derived from N. meningitidis serogroups A and W induced high levels of functional antibodies against both serogroups in mice (Tunheim et al. 2013). Notably, the OMV-induced antibody titer was higher or equal to the levels induced by licensed meningococcal conjugate or polysaccharide vaccines (Tunheim et al. 2013). In line with that, Mitra and coworkers used OMVs derived from six Shigella strains to produce a multiserotype OMV vaccine against shigellosis (Mitra, Chakrabarti and Koley 2013). They demonstrated that immunization of adult female mice with the hexavalent OMV vaccine exhibited a consistent broad-spectrum antibody response, which protected the offspring passively against all four serogroups of Shigella (Mitra, Chakrabarti and Koley 2013). As OMVs consist of multiple antigens, a mixture of several strains is not always necessary as shown by a study introducing OMVs as vaccine candidates against nontypeable Haemophilus influenzae (NTHi) (Roier et al. 2012). Key limitations for vaccine development are the high genetic heterogeneity of NTHi strains and the enormous antigenic variability of several surface-exposed antigens (van Alphen et al. 1997; Erwin and Smith 2007). Interestingly, the induction of a protective immune response against several diverse NTHi strains was comparable regardless of whether only one OMV type or an OMV mixture derived from various heterologous strains was used for immunization (Roier et al. 2012). Most likely, the diverse antigens present in the OMVs are already sufficient to overcome the surface variability of NTHi strains.
This is in contrast to V. cholerae, where the O-antigen was identified as the major protective antigen of an OMV-based cholera vaccine (Leitner et al. 2013). This outermost compartment of the LPS is highly variable between different serogroups. Consequently, only a mixture of OMVs derived from V. cholerae serogroups O1 and O139 induced a high-titer protective immune response against both clinically relevant serogroups (Bishop et al. 2010). Leitner and coworkers extended this vaccine concept and demonstrated that immunization with a mixture of OMVs derived from ETEC and V. cholerae conferred protection against both pathogens (Leitner et al. 2015). Moreover, the study introduced genetically modified strains lacking not only a secondary LPS-acyl transferase but also the potent enterotoxins, i.e. cholera toxin or LT. Although the OMVs from these genetically modified strains exhibited less endotoxicity, they retained their potential to induce a high-titer, protective immune response.
As most reports simply mixed different OMVs in equal amounts, comprehensive dose response studies with variations in the OMV ratios are still lacking. Future initiatives need to address whether the efficacy can be improved upon optimization of the individual OMV amounts in immunization mixture and assess the maximum OMV diversity. This could establish well-balanced OMV combinations as a promising tool for the development of broadly protective vaccines against several Gram-negative pathogens.
Based on the natural traits of OMVs as delivery vehicles (see the next section), OMVs derived from genetically engineerable species have been considered as presentation platform for various heterologous antigens. These antigens need to be either deposited in the lumen of OMVs or displayed on the OMV surface. The target protein-based antigens are therefore fused to toxins (e.g. ClyA), outer membrane proteins (e.g. OmpA) or coupled to endogenous autotransporters (e.g. Hbp, AIDA) (Schroeder and Aebischer 2009; Chen et al. 2010; Bartolini et al. 2013; Daleke-Schermerhorn et al. 2014; Fantappie et al. 2014; Kuipers et al. 2015). Besides, the use of E. coli as host for OMV production also other species have been recently investigated, including V. cholerae for expressing ETEC antigens (CFA/I and Flic), N. meningitidis for expressing a borrelial surface-exposed lipoprotein (OspA) and S. enterica serovar Typhimurium for expressing the pneumococcal protein PspA (Muralinath et al. 2011; Leitner et al. 2015; Salverda et al. 2016). Furthermore, antiviral proteins can be displayed at the surface of OMVs exemplified by a study from Rappazzo and coworkers demonstrating that decoration of E. coli OMVs with M2e (ectodomain of an integral membrane protein of influenza A virus) conferred protection against influenza A infection in mice (Rappazzo et al. 2016).
As highlighted above for V. cholerae, protective antigens are not always proteins, but can also be glycan structures. Thus, OMVs have been investigated as carrier system for heterologous expressed glycan antigens. Using this approach, Chen et al. produced glycosylated OMVs that conferred protection against Francisella tularensis by expression of recombinant O-antigen on E. coli OMVs (Chen et al. 2016). Other glycosylated OMVs have been tested against S. pneumoniae in mice and against Campylobacter jejuni in chicken (Price et al. 2016). Furthermore, glycoengineered OMVs might open the possibility to target clinically important host glycans like those displayed on cancer cells. Proof of principle was reported by Valentine and coworkers demonstrating that immunization with glycosylated OMVs expressing the tumor-specific carbohydrate antigens polysialic acid and Thomsen–Friedenreich (T antigen) induced high titers of glycan-specific IgG antibodies (Valentine et al. 2016). In summary, these studies highlight the potential of OMVs as a vaccine platform for the development of polysaccharide vaccines against various glycan antigens of clinical relevance.
OMVS AS EFFECTOR DELIVERY VEHICLES
Multiple Gram-negative bacteria have been shown to use OMVs as delivery vehicle for toxins, e.g. LT of ETEC, cholera toxin of V. cholerae or multiple toxins (Cif and PlcH) of P. aeruginosa, or to transport enzymes in an active conformation, e.g. proteases of Lysobacter sp. XL1 or Pseudomonas fragi (Thompson, Naidu and Pestka 1985; Wai, Takade and Amako 1995; Horstman and Kuehn 2002; Vasilyeva et al. 2008; Bomberger et al. 2009; Rasti, Schappert and Brown 2018). Pseudomonas putida is able to scavenge nutrients by loading several enzymes to catabolize aromatic compounds (Salvachua et al. 2020). Although OMVs reflect the periplasmic content and surface composition of the donor bacterium, several recent reports highlight enrichment or exclusion of defined components indicating cargo selection (Bonnington and Kuehn 2014). Porphyromonas gingivalis alters its protein cargo depending on the loading with proteases or LPS composition (Haurat et al. 2011). Salmonella enterica serovar Typhimurium exhibits differential composition of LPS in their OMVs upon shifting bacteria to a different pH condition, which promotes LPS remodeling of the outer membrane and might enhance bacterial fitness along environmental transitions (Bonnington and Kuehn 2016). Concordantly, a recent study demonstrated that enhanced OMV release by V. cholerae upon host entry allows a faster surface remodeling highlighted by the depletion of outer membrane porin OmpT and accumulation of glycine-modified LPS (Zingl et al. 2020). These surface adaptations confer resistance to host-derived antimicrobial agents and facilitate in vivo colonization fitness of the pathogen. Helicobacter pylori releases OMVs enriched with the protease HtrA, but lacking the type 4 secretion system component VirD4 (Olofsson et al. 2010). Comparative proteome analyses of OMVs and outer membrane derived from N. meningitidis revealed several distinct differences suggesting a selective packing of cargo into these vesicles (Lappann et al. 2013). For example, autotransporter family proteins, e.g. adhesins and proteases, as well as iron and zinc acquisition proteins were found to be enriched in OMVs, while outer membrane porins PorA and PorB as well as putative transglycosylases were drastically underrepresented in OMVs. Uropathogenic E. coli are able to specifically sort the CNF1 into OMVs, which seems to be dependent on the folate-binding protein YgfZ (Yu and Kim 2012). However, evidence for direct interaction between CNF1 and YgfZ is still lacking. Thus, the exact molecular mechanisms and bacterial strategies for differential sorting into the OMVs remain to be elucidated and will be valuable for future use of OMVs as vehicles for effector delivery.
OMVs have a natural ability to stabilize enzyme activity in their lumen and allow a flux of substrate and product through outer membrane porins. Thus, it is not surprising that multiple studies have been conducted investigating the beneficial effects of OMV encapsulation for enzymatic activity (Alves et al. 2015, 2016). Although these reports mainly focused on biocatalysis in biotechnological processes (Fig. 1), these initiatives can path the way for future therapeutical applications. Briefly, current studies highlight that temperature-sensitive enzymes protected within OMVs exhibit increased stability against repeated freeze/thaw cycles or prolonged storage at unfavorable, elevated temperatures (up to 37°C) than the soluble, purified enzyme version. Therefore, OMVs show the same advantages as conventional packing of enzymes into artificial lipid-based micelles or liposomes (Kraft et al. 2014), but can be purified by relative simple high-speed centrifugation, can be already preloaded by the bacteria and can enable the transport of hydrophobic compounds (Brameyer et al. 2018). The latter can be valuable for several biotechnological processes, since many enzymes of interest are highly unstable in aqueous solutions (Sada et al. 1987). For example, Alves and coworkers used OMVs enriched with a phosphotriesterase to efficiently degrade the organophosphate chemical warfare agent simulant paraoxon in environmental water samples highlighting the possible benefits of OMVs for bioremediation (Alves et al. 2018). Furthermore, OMVs decorated with organophosphorus hydrolases were used for biocatalytic decontamination of paraoxon, a highly toxic compound previously used as insecticide (Fu-Hsiang, Chih-Yun and Shen-Long 2017). The OMV-associated enzyme showed increased activity, prolonged half-life, improved temperature stability and enhanced pH tolerance (Fu-Hsiang, Chih-Yun and Shen-Long 2017).
To deposit heterologous proteins of interest in OMVs, several techniques have been reported (Li and Liu 2020b). A classical strategy comprises the fusion of the protein of interest to a signal sequence known to facilitate export to the periplasm. Upon accumulation in the periplasm, the protein of interest will be stochastically trapped in OMVs. Applicable secretion pathways include the Sec system or the twin arginine translocation (TAT) system (Oliver and Beckwith 1982; Chaddock et al. 1995). Latter has the advantage of exporting already folded proteins. Proof of principle was reported by efficient loading of E. coli OMVs with GFP (green fluorescent protein) fused to a TAT signal sequence, which allowed OMV detection by fluorescence measurements (Kesty and Kuehn 2004). Alternatively, the peptides of interest can be fused to target proteins, which are known to be transported to the outer membrane of the host bacterium. Prominent examples are fusions to the outer membrane protein OmpA or ClyA, which harbors a leader sequence resulting in the display of a functional protein on the surface of the vesicles (Kim et al. 2008, 2017a; Lee et al. 2009). Autotransporter systems, such as the Neisseria IgA protease or the E. coli AIDA-I, have also been successfully used to engineer strains for heterologous peptide display on OMVs, including ovalbumin fragments as well as Leishmania and ETEC antigens (Schroeder and Aebischer 2009; Hays et al. 2018; Schetters et al. 2019). A more broadly applicable system is the SpyTag/SpyCatcher system, which can form a covalent linkage between a protein of interest and the delivery system (Brune et al. 2016). Briefly, a protein of interest and an OMV-associated protein are tagged with either the SpyTag or the SpyCatcher domains, respectively. SpyTag and SpyCatcher domains interact with one another and form a covalent isopeptide bond. This technology has been recently used to link a phosphotriesterase to OmpA and thereby guiding the enzyme into OMVs (Alves et al. 2015). Using an ice nucleation protein anchoring motif, Park and coworkers managed to display a trivalent complex of functional cellulase domains on OMVs resulting in faster cellulose hydrolysis than the noncomplexed enzymes (Park et al. 2014). Similarly, the abovementioned organophosphorus hydrolases for pesticide degradation were displayed via the ice nucleation protein technology (Fu-Hsiang, Chih-Yun and Shen-Long 2017). OMV donor strains can also be genetically engineered to display glycan structures on their surface utilizing the loose glycan specificity of the O-antigen ligase. For example, the S. pneumoniae and C. jejuni surface glycans were expressed in an O-antigen E. coli variant resulting in glycosylation-modified OMVs, which induced a protective immune response in animal models (Price et al. 2016).
OMVs have been proposed to be used as a cell-specific drug delivery vehicle as they are able to target specific cells, can protect their cargo and are readily taken up by eukaryotic cells (Fig. 1). Interestingly, transmigration through epithelial cells and systemic distribution into distant organs, in particular the liver, was observed for OMVs derived from the gut commensal B. thetaiotaomicron upon oral administration (Jones et al. 2020). Thus, OMVs could be interesting candidates for long-distance, systemic distribution of effector molecules. Detoxified OMVs derived from E. coli K-12 ΔmsbB have been shown to be effectively internalized by cancer cells via overexpression of an affibody on their surface (Gu et al. 2020). Affibodies were among the first biotechnological engineerable nonantibody scaffold molecules, which allow high-affinity binding to a large number of target proteins (Frejd and Kim 2017). They are small, robust proteins designed on the Z domain of the protein A from S. aureus. Affibodies imitate monoclonal antibodies and show similar antigen-binding capacity, yet are significantly smaller and exhibit improved stability properties. In the study of Gujrati and coworkers, they were used to target specifically a transmembrane receptor (HER2) overexpressed on several cancer cells (Gujrati et al. 2014). Loading of these vesicles with siRNAs to silence the expression of a kinesin spindle protein resulted in significant reduction of tumor growth in animal models (Gujrati et al. 2014). Similarly, detoxified OMVs derived from E. coli have recently been shown to deliver a tumor necrosis factor-related apoptosis-inducing ligand to cancer cells via integrin-targeting peptides and allow deep tissue penetration, which is important for tumor eradication (Gu et al. 2020). Notably, E. coli OMVs have already been shown to have natural tumor-suppressive properties by inducing the production of antitumor cytokines CXCL10 and interferon-γ (Kim et al. 2017b). These natural properties could be further amplified by presenting the ectodomain of programmed death 1 (PD1) on OMVs, which enabled the modified OMVs to bind the PD1 ligand 1 on tumor cells negating the inhibition of T-cell proliferation by tumor cells (Li et al. 2020d). Overall, the activity of the bioengineered OMVs, i.e. immune activation in the tumor microenvironment and suppression of immune evasion by the tumor cells, resulted in a marked reduction of tumor growth in vivo (Li et al. 2020d). Moreover, a recent study demonstrated that Klebsiella pneumoniae OMVs can be effectively enriched with the antitumor agent doxorubicin to treat lung cancer in the murine model (Kuerban et al. 2020). Notably, high cardiac toxicity, being a major adverse, dose-limiting effect of free doxorubicin, was significantly reduced upon association with OMVs (Kuerban et al. 2020).
Recent approaches also include the decoration of OMVs with tumor-specific epitopes as potentially tools for cancer immunotherapy. For example, Grandi and coworkers demonstrated that immunization of mice with E. coli OMVs enriched with epitopes of the tumor markers B16-M30 and B16-M30 was protected from a tumor challenge using B16F10EGFRvIII cells expressing the tumor markers, respectively (Grandi et al. 2017). Similarly, injection of E. coli OMVs enriched with tumoral antigen human papillomavirus type 16 early protein E7 induced potent antitumor CD4+ and CD8+ cell responses suppressing TC-1 tumor growth in mice (Wang et al. 2017).
Notably, the potent self-adjuvant properties of OMVs resulting in strong immunogenicity can be attributed to the high abundance of MAMPs on the bacterial surface, which trigger pro-inflammatory pathways (Mahla et al. 2013). A fine balance between immunogenicity and reactogenicity is key for successful treatment approaches of OMV-based drugs. While a refined triggering of inflammatory cascades can be beneficial for the humoral immune response to an OMV-based vaccine, a strong activation of innate immune responses may cause severe adverse effects, such as fever and septic shock. Such disadvantageous reactogenicity of OMV-based drugs needs to be carefully assessed and potentially minimized, especially in case of potential applications in immunocompromised patients. One of the most abundant and highly reactogenic components in OMVs is the LPS. As described above, specific extraction steps as well as genetic modifications of the donor bacterium can be applied to reduce the reactogenicity of the LPS in OMVs, while retaining their immunogenicity (Holst et al. 2009, 2013; Leitner et al. 2013; Mitra, Chakrabarti and Koley 2013). Practicability and potential requirement of further modifications in the context of immunocompromised patients remain to be elucidated. Aside from the high reactogenicity of OMV-associated MAMPs, additional challenges have to be addressed along the development of OMV-based cancer vaccines, including the selection of suitable antigens for the diverse cancer types, efficacy of heterologous antigen expression and removal of natural immunodominant or immunosuppressive components from OMVs (Zhang et al. 2019).
CONCLUDING REMARKS AND FUTURE PERSPECTIVES
It is becoming increasingly evident that OMVs may be valuable tools for a variety of future medical applications, such as vaccination, cancer therapy and drug delivery. Most progress has been made along the vaccine development with first OMV-based vaccine being approved and commercially available. Thus, studies dealing with safety aspects and ‘good manufacturing practice’ production have been mainly focusing on this application (Frasch et al. 2001; van de Waterbeemd et al. 2010, 2013b; Berlanda Scorza et al. 2012; Rossi, Citiulo and Mancini 2021). As OMVs are produced by living bacteria, several steps are necessary to ensure safety, such as (i) close monitoring of bacterial growth, (ii) standardized isolation techniques using centrifugation, filtration and/or detergent extraction, (iii) sterilization and DNA removal, (iv) purity, quantity and composition analyses of the isolated OMVs and, if applicable, (v) the control of heterologous antigen expression. Furthermore, several assays have been described to assess reactogenicity and toxicity of isolated OMVs, such as the rabbit pyrogenicity test, the monocyte activation test, the limulus amoebocyte lysate test or receptor-specific tests in engineered cell lines (Rossi, Citiulo and Mancini 2021). OMVs are vital players for the bacteria–host crosstalk, e.g. via modulation and shaping of the host's immune response. OMVs may also be attractive multifunctional delivery vehicles for proteins, glycans or small-molecule drugs, which are protected and stabilized in association with OMVs. Moreover, OMVs are efficiently internalized by host cells and first reports indicate that OMVs can be used to target specific cell types. Understanding the host cell responses evoked by individual OMVs from diverse bacterial species, elucidating the principles of OMV internalization into target cells, identification of the involved bacterial effectors, and characterization of their cellular binding partners and pathways are necessary future steps for potential therapeutic applications. Furthermore, future research needs to address current limitations such as safety of OMVs, OMV loading or decoration with heterologous substances, and upscaling of OMV production and purification.
ACKNOWLEDGMENTS
Apologies to all scientists studying OMVs whose work and research could be not mentioned in this review.
FUNDING
This work was supported by the Austrian FWF grant P33073 to SS, DOC-50 (docfund ‘Molecular Metabolism’) to SS and W901-B12 (DK Molecular Enzymology) to FGZ and SS, by the Else Kröner-Fresenius-Stiftung, by BioTechMed-Graz, and by the Land Steiermark and City of Graz.
Conflict of interest
None declared.
REFERENCES
Author notes
These authors contributed equally to this work.