-
PDF
- Split View
-
Views
-
Cite
Cite
Goro Sasaki, Mohamad Zubair, Tomohiro Ishii, Toshikatsu Mitsui, Tomonobu Hasegawa, Richard J. Auchus, The Contribution of Serine 194 Phosphorylation to Steroidogenic Acute Regulatory Protein Function, Molecular Endocrinology, Volume 28, Issue 7, 1 July 2014, Pages 1088–1096, https://doi.org/10.1210/me.2014-1028
- Share Icon Share
The steroidogenic acute regulatory protein (StAR) facilitates the delivery of cholesterol to the inner mitochondrial membrane, where the cholesterol side-chain cleavage enzyme catalyzes the initial step of steroid hormone biosynthesis. StAR was initially identified in adrenocortical cells as a phosphoprotein, the expression and phosphorylation of which were stimulated by corticotropin. A number of in vitro studies have implicated cAMP-dependent phosphorylation at serine 194 (S194, S195 in human StAR) as an important residue for StAR activity. To explore the importance of S194 phosphorylation in StAR function in vivo, we developed a transgenic model using a bacterial artificial chromosome expressing either wild-type (WT) StAR or StAR mutation S194A to rescue StAR knockout (KO) mice. Despite StAR protein expression comparable to or higher than amounts seen with control animals or rescue with WT StAR, S194A StAR did not rescue the neonatal lethality and only partially rescued the sex reversal in male mice observed uniformly in StAR KO mice. Like the StAR KO mice, the adrenal cortex and testicular Leydig cells contained abundant lipid deposits when stained with oil red O. Adrenal StAR from S194A rescue animals lacks an acidic species, which appears upon corticotropin stimulation in animals rescued with WT StAR, consistent with defective StAR phosphorylation. These findings demonstrate that S194 is an essential residue for normal StAR function in the adrenal cortex and testes of mice.
The induction of steroidogenesis by trophic hormones can be divided into acute and chronic phases (1). The acute phase occurs within seconds to minutes and involves mobilization and delivery of cholesterol to the cholesterol side-chain cleavage enzyme (Cyp11a1) on the inner mitochondrial membrane. The chronic phase, in contrast, occurs over hours to days and reflects increased transcription of genes encoding constituents of the steroidogenic pathways and trophic stimulation of the adrenals and gonads. The steroidogenic acute regulatory protein (StAR) facilitates cholesterol delivery from the outer mitochondrial membrane to the inner mitochondrial membrane, where Cyp11a1 catalyzes the first committed step in steroid biosynthesis (2–4). Genetic defects of StAR cause the human disease lipoid congenital adrenal hyperplasia (LCAH, MIM 201710) (5, 6), a condition recapitulated in StAR knockout (KO) mice (7), leading to severely impaired steroid hormone synthesis and abundant lipid deposits containing cholesterol esters in the adrenal cortex and steroidogenic cells of the gonads (8, 9).
StAR initially was identified as a 37-kDa cytosolic precursor protein and a 30-kDa mitochondrial mature protein, the expression and degree of phosphorylation of which were induced upon treating adrenal and Leydig cells with trophic hormones or cAMP analogs (10, 11). Subsequent to the cloning of StAR cDNA, distinct residues that are modified by phosphorylation have been identified, including serine 194 in mouse Star (S194, equivalent to S195 in human StAR), which is conserved among most species (12, 13). In steroidogenic cells, protein kinase A (PKA) mediates StAR phosphorylation upon stimulation with extracellular trophic hormones and elevated intracellular cAMP (11). Several studies have documented that the PKA-mediated phosphorylation at S194/S195 activates StAR function. For example, the mouse Y1 adrenocortical tumor cell PKA-deficient mutants Kin-7 and Kin-8 respond poorly to ACTH and cAMP due to defective cholesterol mobilization, and transfection with wild-type (WT) PKA rescues this defect (10). Another in vitro assay using transiently transfected nonsteroidogenic COS-1 cells demonstrated that substitution of the nonphosphorylatable residue alanine, with mutation S194A for mouse and hamster or S195A for human StAR, decreased StAR function by approximately 50% (12, 13). A heterozygous S195A mutation in StAR was identified in a LCAH patient (14), but this study could not determine whether the reason for StAR loss of function was the absence of S195 phosphorylation. Despite these data from model systems, the precise in vivo role of S194/S195 phosphorylation in the adrenal cortex and Leydig cells remains unknown.
To resolve the controversy of StAR phosphorylation at S194/S195 in the steroidogenic cells of an intact animal, we previously developed a mouse rescue model wherein the WT Star transgene functioned as well as the endogenous gene, leading to full rescue of the LCAH phenotype in Star KO mice (15). In this study, we generated another transgene-rescue mouse, replacing WT Star with mutation S194A, to determine the in vivo role of the posttranslational Star phosphorylation in the adrenal cortex and Leydig cells.
Materials and Methods
Experimental animals
The Institutional Animal Care Research Advisory Committee at University of Texas Southwestern approved all mouse studies. Homologous recombination was used as previously described (16) to modify the WT-Star bacterial artificial chromosome (BAC) that was isolated from a C57Bl/6J mouse genomic DNA library to substitute alanine for serine at codon 194 (S194A, Figure 1). In brief, a 1-kb recombination cassette was cloned into pBluescript KS (−) (Stratagene), and a 1-nucleotide substitution was introduced at codon 194 of the Star coding sequence (TCC, serine, to GCC, alanine) using QuikChange site-directed mutagenesis kit (QIAGEN). The recombination cassettes were subcloned into the shuttle vector pSV1-RecA and then transformed into recombination-deficient Escherichia coli containing the WT-Star BAC. Following homologous recombination and resolution, BAC modification was verified by pulsed-field gel electrophoresis and Southern blot analysis, and the S194A substitution was confirmed by direct sequencing.
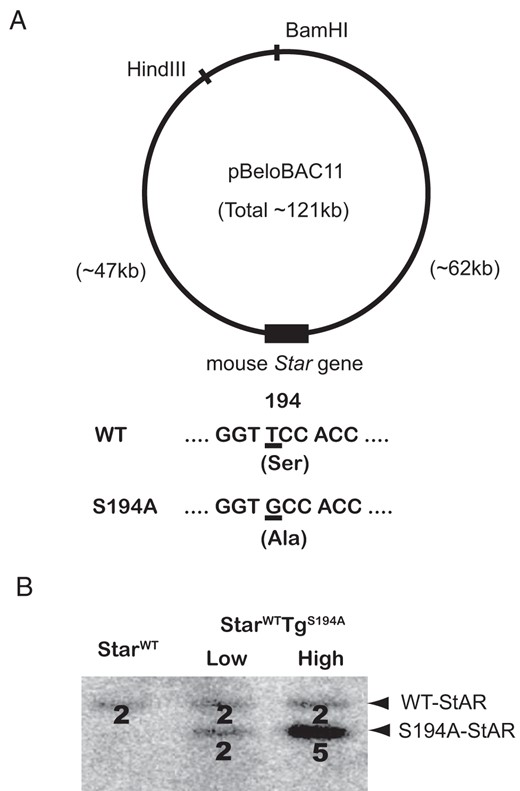
Generation of the S194A-Star BAC transgenic mice. A, Structure of the Star BAC clone. The mouse Star gene was cloned into the HindIII and BamHI sites of pBeloBAC11. The 121-kb Star BAC clone contains the Star structural gene, 47 kb of 5′-flanking region, and 62 kb of 3′-flanking region. The S194A-Star BAC contains the TCC-to-GCC substitution, which encodes the S194A mutant Star protein. B, Two independent S194A-Star BAC transgenic lines (designated “Low” and “High”) were generated. Quantitative Southern blotting after BanI digestion with a 32P-labeled Star probe demonstrates 2 copy numbers (line “Low”) and 5 copy numbers (line “High”) of the S194A-Star transgenes relative to StarWT mice. For counting copy numbers, the analyzed transgenic mice were StarWT and StarWT carrying the S194A-StAR transgene (StarWTTgS194A mice).
The BAC DNAs for pronuclear injection were prepared with the large-construct kit (QIAGEN) followed by purification with CsCl gradient DNA ultracentrifugation. Each BAC was injected as supercoiled DNA into the pronucleus of C57Bl/6J fertilized eggs to produce transgenic pups, which were identified by genomic PCR as previously described (15).
Transgenic mice were always maintained as Star heterozygotes (Star+/−). Either WT- or S194A-Star transgenic mice were crossed with Star+/− mice maintained as a congenic line on the C57BL/6J background (The Jackson Laboratory), to produce Star-knockout (StarKO) mice carrying either the WT-Star BAC transgene (StarKOTgWT) or the S194A-Star BAC transgene (StarKOTgS194A). StarKOTgWT mice were genotyped by quantitative Southern blotting (see below).
StarKOTgS194A mice were genotyped by allele-specific PCR to exclude StAR KO allele that lacks exon 2, intron 2, and exon 3, using a forward primer of exon 3 (5′-AGCTTCTGCCAGTGATGGGTC-3′) and a reverse primer of exon 6 (5′-AAAACCTGCAAGAGGCTTCTCTATCTATGC-3′), followed by digestion with the restriction enzyme BanI, which selectively digests the S194A alleles. StarKO mice were also produced by matings of Star+/− mice, genotyped as previously described (8, 9, 15). All mice were housed in temperature-controlled rooms with a 12-hour light, 12-hour dark cycle and were given food and water ad libitum.
For analyses, newborn mice were killed by decapitation at day 0 of age. To analyze phosphorylated StAR protein, some StarWT newborn mice were treated with 3 sequential sc injections with 10 μg ACTH1–24 fragment (Sigma-Aldrich), followed by decapitation.
Southern blot analysis
Southern blotting was used to analyze the structure of the Star BAC clones and transgenes as previously described (15). In brief, 10 μg of DNA purified from tail snips was digested overnight with NcoI and BanI at 37°C and separated on a 1% agarose gel at 100 V for 2 hours. After transfer to a Nytran membrane (Whatman), the digested DNA was hybridized to a 32P-labeled probe corresponding to 0.7 kb of the Star gene encompassing exon 5 and intron 5 of S194A-StAR BAC. Radioactivity was detected using a Storm 820 scanner (Molecular Dynamics) and quantified using ImageQuant software (Molecular Dynamics).
RNA extraction and real-time RT-PCR
Total RNA was extracted from adrenal glands and testes of newborn mice using TRIzol reagent (Invitrogen) and treated with DNaseI (Roche). Reverse transcription of 1 μg of the RNA was carried out using random hexamers (Roche) and SuperScriptIII reverse transcriptase (Invitrogen). For quantitative analysis of Star, Taqman gene expression assay was performed using the ABI Prism 7700 Sequence Detection system (Applied Biosystems) with the 6FAM-dye-labeled Taqman MGB probes for mouse Star (Mm00441558_m1) and for eukaryotic 18S rRNA (Hs99999901_s1) (Applied Biosystems). Expression values were analyzed by the standard curve method and normalized for 18S rRNA. Cyp11a1 and Hsd3b2 mRNAs were quantified by the intercalater method using their primer pairs and SYBR Premix Ex Taq II (Takara Bio Inc) and ABI Prism 7500 Fast (Applied Biosystems), normalized for Gapdh as previously described (17). All reactions were performed in triplicate to assess well-to-well variability, and only curves having a high correlation coefficient (r2 > 0.99) were used.
Protein preparation and one-dimensional and two-dimensional immunoblotting
Whole adrenal glands and testes were homogenized with a sterile pellet pestle grinder (Kontes) and resuspended in sample buffer (7.2 M urea/ 1.6% Triton X-100/0.8% dithiothreitol/2% lauryl sulfate, all from Sigma-Aldrich). Homogenates (10 μg of protein) were mixed with Tris-Glycine sodium dodecyl sulfate (SDS) sample buffer (Invitrogen), separated on a 12% Tris-glycine gel (Invitrogen) along with prestained molecular weight markers (Bio-Rad Laboratories) and electrophoretically transferred to polyvinylidene difluoride membrane (Bio-Rad). Immunoblot analysis was performed using a rabbit polyclonal anti-StAR antiserum (1:10 000, kindly provided by Dr Dale Hales) and rabbit anti-β-actin antiserum (1:5000, Novus), followed by antirabbit IgG (whole molecule) peroxidase conjugate (1:10 000, Sigma-Aldrich). Proteins were visualized by exposure to x-ray film following treatment of the membrane with Luminol chemiluminescence reagent (Santa Cruz Biotechnology).
Two-dimensional electrophoresis was done as previously described with some modifications (18). A first-dimensional isoelectric focusing gel was made in a small glass rod (1.2 mm diameter × 65 mm), which contained 9.5 M urea, 4.5% polyacrylamide, 1.5% 3-[(3-cholamidopropyl)dimethylammonio]-1-propanesulfonate, 1% Nonidet P-40, and 5% ampholytes (pH 3–10; catalog no. ZM0021, Invitrogen). The upper running electrolyte-cathode buffer was 20 mM sodium hydroxide, and the anode buffer was 10 mM phosphoric acid. Prefocusing was carried out at 150 V for 30 minutes. The homogenized samples were mixed with 2 volumes of sample dilution buffer, which contained 1.25% SDS, 0.25% dithiothreitol, 1% 3-[(3-cholamidopropyl)dimethylammonio]-1-propanesulfonate, 2.25 M urea, and 1.25% ampholytes (pH 3–10). Twenty-microgram (∼10 μL) protein samples were applied on the gel rods, and electrophoresis was performed at a constant voltage of 150 V for 30 minutes and thereafter 250 V for 4 hours. Gel rods were then equilibrated with 2× Tris-glycine SDS-sample buffer for 15 minutes (125 mM Tris, pH 6.8, 0.1% SDS), and the gel rods were fixed with 0.8% agarose on the top of the second gel. Electrophoresis was performed in the second dimension on a 12% SDS-PAGE gel, and immunoblotting was performed using the same methods as described above.
Hormone assays
Blood was collected from decapitated newborn mice. Plasma or serum concentrations of hormones were measured with commercially available ELISA kits according to the manufacturers' protocols: ACTH (MD Biosciences) with range of 8–475 pg/mL, limit of detection of 0.46 pg/mL, and intraassay and interassay coefficients of variation (CVs) of 4.2% and 6.2%, respectively; for corticosterone (IBL Laboratories) with range of 0–240 ng/mL, limit of detection of 1.7 ng/mL, and intraassay and interassay CVs of 3.1% and 6.0%, respectively; and for testosterone (IBL Laboratories) with range of 0–16 ng/mL, limit of detection of 0.1 ng/mL, and intraassay and interassay CVs of 3.6% and 7.1%, respectively.
Histologic analysis
Morphologic analysis was performed with frozen tissues harvested from newborn mice. The frozen tissues were embedded in optical cutting temperature compound (Sakura Finetex USA Inc) and sectioned at 10 μm with a Cryostat CM1900 (Leica Corp Instruments GmbH). The sections were analyzed by staining with oil red O (Sigma-Aldrich) and hematoxylin (Richard-Allan Scientific).
Statistical analysis
The mean values between groups were analyzed by Mann-Whitney U test; differences with P < .05 were considered significant.
Results
Modification of the Star BAC, changing serine 194 to alanine (S194A), and generation of BAC transgenic mice
To permit us to explore structure-function aspects of StAR within steroidogenic cells, we previously developed a system to drive Star expression in vivo, using bacterial artificial chromosome (BAC) transgenesis (15). The WT-Star BAC clone that we previously described includes 47 kb upstream of the transcription initiation codon, the entire Star structural gene, and 62 kb downstream of the termination codon (Figure 1A). To determine the consequences of preventing phosphorylation at serine 194, we modified the WT-Star BAC with mutation S194A (S194A-Star). The S194A-Star BAC was then used to generate 2 distinct transgenic lines: transgenic mice with 2 copies of the transgene (Tg-LowS194A) and 5 copies of the transgene (Tg-HighS194A) (Figure 1B). The BAC transgenesis did not cause any overt phenotypic changes in the transgenic mice heterozygous for carrying the S194A-Star transgene with comparable copy numbers (StarWTTg-LowS194A), whereas the transgenic mice heterozygous for carrying the S194A-Star transgene with relatively higher copy numbers (StarWTTg-HighS194A) exhibited decreased fertility (see below).
To characterize Star transcripts and proteins directed by the Star transgenes, we crossed either StarWTTg-LowS194A or StarWTTg-HighS194A mice with mice heterozygous for Star KO allele (Star+/−), generating Star KO (StarKO) mice that carried either the Tg-LowS194A or Tg-HighS194A (StarKOTg-LowS194A or StarKOTg-HighS194A, respectively). In samples obtained from the adrenal glands and testes of newborn mice, the Tg-LowS194A transgene directed expression of Star mRNA and protein at levels roughly comparable to those seen in StarWT mice (Figure 2, A and B). In contrast, the Tg-HighS194A transgene directed 3- to 5-fold higher expression of Star mRNA and protein than was found in StarWT mice. Thus, the 2 different Star transgenes provided the opportunity to examine the effect of either normal or high expression of S194A-Star. Because StarKO females are known to retain normal estradiol production in the neonatal ovaries without apparent histologic changes (7, 8), we mainly focused on the adrenal and testicular function in this study.
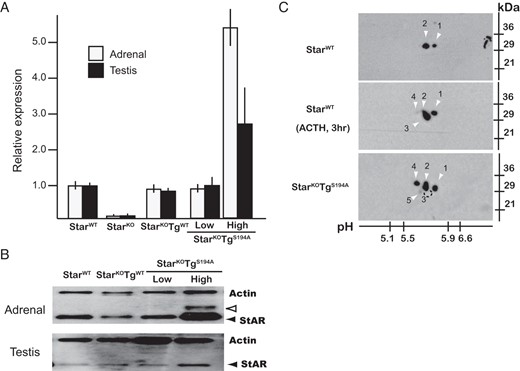
Expression of Star mRNA and protein in StarKO mice carrying the WT or S194A-Star transgene (StarKOTgWT or StarKOTgS194A mice, respectively). A, Transcriptional levels of Star mRNA: The S194A Star BAC transgenes direct normal levels of Star mRNA in the adrenal cortex and testis. Adrenal or testicular RNA was extracted from newborn pups and analyzed by real-time RT-PCR. Transcript levels are normalized to StarWT mice (1.0), and data are shown as mean ± SEM (n = 5). B, Expression levels of Star protein. Tissue lysates were prepared from adrenal glands and testes of newborn mice, and levels of Star protein were quantitated by immunoblot analyses using anti-StAR and antiactin antisera. The Star protein was detected as a preprotein of 37 kDa (open arrowhead) and a mature protein of 30 kDa (solid arrowhead), and their levels are comparable among StarWT, StarKOTgWT (StarKO mice carrying WT-Star transgene), and StarKOTg-LowS194A mice, but significantly higher in StarKOTg-HighS194A mice. C, Adrenal Star protein resolved by two-dimensional electrophoresis. Proteins were obtained from adrenal glands of newborn mice. To stimulate S194 phosphorypation, StarWT mice were injected with ACTH1–24 before decapitation, as described in Materials and Methods. Mature StAR of approximately 30 kDa migrated from pH 5.5 to 5.9, shown as species 1–5. In StarWT mice, species 1 and 2 were recognized in the basal state, and species 1, 2, 3, and 4 were detected after ACTH1–24 stimulation. In StarKOTg-LowS194A mice, species 3 disappeared, whereas species 4 was augmented, and species 5 emerged.
An important premise of our transgenic strategy is that the S194A missense mutation interferes with PKA-mediated phosphorylation of Star protein. To verify this prediction, we subjected total adrenal extracts from animals before and after ACTH1–24 stimulation to two-dimensional gel electrophoresis as described in Materials and Methods. WT-Star protein migrated as 2 species with different isoelectric points (1 and 2; Figure 2C), and 2 additional forms (3 and 4; Figure 2C) emerged after ACTH1–24 stimulation, most prominently species 3. In the StarKOTg-LowS194A mice that exclusively expressed the S194A-Star transgene, species 3 did not appear despite ACTH1–24 stimulation (see below), species 4 was increased, and a new species 5 emerged with a more acidic isoelectric point, suggesting another chemical modification at other site(s) of the Star protein. These data are consistent with species 3 representing a Star molecule phosphorylated at S194 upon ACTH stimulation.
The S194A-Star transgene fails to rescue the phenotypes of StarKO mice
We next examined the function of the Star protein in primary steroidogenic tissues of newborn mice. To compare the function of WT-Star and the S194A mutation, we used StarKO mice carrying the WT-StAR transgene (StarKOTgWT), which show comparable expression of StAR mRNA and protein to StarWT mice in the adrenal glands and testes, as previously reported (15).
One important indicator of Star transgene product function is the ability to rescue adrenocortical insufficiency. StarKO mice invariably died within the first week after birth as we found previously (7), whereas nearly all StarKOTgWT mice survived the postnatal period comparable to StarWT mice, demonstrating that the WT-Star BAC is sufficient to rescue viability for the StarKO mice (15). In contrast, both the StarKOTg-LowS194A and StarKOTg-HighS194A mice exhibited high neonatal lethality, but less than that seen in StarKO mice (Table 1), suggesting that comparable expression of S194A-Star protein fails to restore StAR function. Because we could obtain some viable StarKOTg-LowS194A, StarKOTg-HighS194A, and StarKO mice at 0.5 day after birth, we used these surviving newborn mice for further studies of Star function.
. | StarKO . | StarKOTgS194A . | StarKOTgWT . | |
---|---|---|---|---|
Low . | High . | |||
Viable StarKO ± Tg mice (n) | 6 | 13 | 2 | 35 |
Viable WT littermates (n) | 36 | 31 | 5 | 35 |
Viability (%) | 17 | 42 | 40 | 100 |
Masculinized mice (n) | 0 | 3 | 2 | 20 |
Total male mice (n) | 5 | 9 | 2 | 20 |
Masculinization (%) | 0 | 33 | 100 | 100 |
. | StarKO . | StarKOTgS194A . | StarKOTgWT . | |
---|---|---|---|---|
Low . | High . | |||
Viable StarKO ± Tg mice (n) | 6 | 13 | 2 | 35 |
Viable WT littermates (n) | 36 | 31 | 5 | 35 |
Viability (%) | 17 | 42 | 40 | 100 |
Masculinized mice (n) | 0 | 3 | 2 | 20 |
Total male mice (n) | 5 | 9 | 2 | 20 |
Masculinization (%) | 0 | 33 | 100 | 100 |
Because most StarKO and StarKOTgS194A mice were neonatal lethal, viability and masculinization were assessed on day 0.5. Viability was calculated on numbers of viable WT mice in the same litter. Masculinization was macroscopically evaluated by scrotum pigmentation and anogenital distance of survived male mice.
. | StarKO . | StarKOTgS194A . | StarKOTgWT . | |
---|---|---|---|---|
Low . | High . | |||
Viable StarKO ± Tg mice (n) | 6 | 13 | 2 | 35 |
Viable WT littermates (n) | 36 | 31 | 5 | 35 |
Viability (%) | 17 | 42 | 40 | 100 |
Masculinized mice (n) | 0 | 3 | 2 | 20 |
Total male mice (n) | 5 | 9 | 2 | 20 |
Masculinization (%) | 0 | 33 | 100 | 100 |
. | StarKO . | StarKOTgS194A . | StarKOTgWT . | |
---|---|---|---|---|
Low . | High . | |||
Viable StarKO ± Tg mice (n) | 6 | 13 | 2 | 35 |
Viable WT littermates (n) | 36 | 31 | 5 | 35 |
Viability (%) | 17 | 42 | 40 | 100 |
Masculinized mice (n) | 0 | 3 | 2 | 20 |
Total male mice (n) | 5 | 9 | 2 | 20 |
Masculinization (%) | 0 | 33 | 100 | 100 |
Because most StarKO and StarKOTgS194A mice were neonatal lethal, viability and masculinization were assessed on day 0.5. Viability was calculated on numbers of viable WT mice in the same litter. Masculinization was macroscopically evaluated by scrotum pigmentation and anogenital distance of survived male mice.
Because Star also regulates testosterone synthesis in the fetal testes, masculinization of the external genitalia provides another indirect indicator of Star function in male mice. As shown in Table 1, all StarKO males had completely feminized external genitalia, confirming the indispensable role of StAR in male sexual development. Consistent with our previous findings, the StarKOTgWT males exhibited normal male external genitalia, indicating that the WT-Star BAC directed sufficient Star protein expression to produce enough testosterone for normal male sexual development. In contrast, 6 of the 9 StarKOTg-LowS194A mice (67%) possessed undermasculinized external genitalia with hypoplastic penis, poorly pigmented scrotum, and a shortened anogenital distance, which were indistinguishable from females. The remaining one-third of the StarKOTg-LowS194A males, however, exhibited normally masculinized external genitalia, suggesting that S194A-Star retains some activity. Furthermore, all of the StarKOTg-HighS194A mice, which possess 5 copies of the S194A-StAR transgene and express substantially more Star protein than StarKOTg-LowS194A mice, were phenotypically male. These findings suggest that S194A-Star retains a trace of activity, which is generally inadequate to masculinize the external genitalia; however, high expression of S194A-Star supports sufficient testosterone production in utero to masculinize male mice.
The StarKOTgS194A mice showed diminished steroid production comparable to StarKO mice
To quantitatively compare the function of the WT- and S194A-Star transgenes, we determined circulating levels of steroid hormones in newborn mice of different genotypes (Table 2). To evaluate Star function in the adrenal cortex and testes, we measured plasma ACTH, serum corticosterone (the predominant glucocorticoid in mice), and serum testosterone levels for the StarKOTg-LowS194A mice. In StarKO mice, plasma ACTH levels were significantly elevated, and serum corticosterone and testosterone levels were markedly decreased, consistent with severe primary adrenal insufficiency and testicular dysfunction. In the 2 transgene-rescued mice, StarKOTgWT mice had normal ACTH, corticosterone, and testosterone levels, whereas StarKOTg-LowS194A mice showed significantly elevated ACTH and reduced corticosterone and testosterone levels, comparable to those in StarKO mice. These data argue that the S194A-StAR protein cannot maintain normal steroidogenesis in the adrenal and testes.
. | ACTH (pg/mL) . | Corticosterone (ng/mL) . | Testosterone (ng/mL) . |
---|---|---|---|
(n = 10) | (n = 10) | (male, n = 5) | |
StarWT | 36 ± 6.8 | 42 ± 9.9 | 0.25 ± 0.03 |
StarKO TgWT | 23 ± 0.7 | 43 ± 7.1 | 0.28 ± 0.06 |
StarKO Tg-LowS194A | 390 ± 83 a | 19 ± 3.5 a | 0.15 ± 0.01 a |
StarKO | 343 ± 68 a | 15 ± 3.4 a | 0.16 ± 0.01 a |
. | ACTH (pg/mL) . | Corticosterone (ng/mL) . | Testosterone (ng/mL) . |
---|---|---|---|
(n = 10) | (n = 10) | (male, n = 5) | |
StarWT | 36 ± 6.8 | 42 ± 9.9 | 0.25 ± 0.03 |
StarKO TgWT | 23 ± 0.7 | 43 ± 7.1 | 0.28 ± 0.06 |
StarKO Tg-LowS194A | 390 ± 83 a | 19 ± 3.5 a | 0.15 ± 0.01 a |
StarKO | 343 ± 68 a | 15 ± 3.4 a | 0.16 ± 0.01 a |
The values were expressed as the mean ± SEM.
, P < .05. StarKO Tg-HighS194A mice were not available due to low fertility.
. | ACTH (pg/mL) . | Corticosterone (ng/mL) . | Testosterone (ng/mL) . |
---|---|---|---|
(n = 10) | (n = 10) | (male, n = 5) | |
StarWT | 36 ± 6.8 | 42 ± 9.9 | 0.25 ± 0.03 |
StarKO TgWT | 23 ± 0.7 | 43 ± 7.1 | 0.28 ± 0.06 |
StarKO Tg-LowS194A | 390 ± 83 a | 19 ± 3.5 a | 0.15 ± 0.01 a |
StarKO | 343 ± 68 a | 15 ± 3.4 a | 0.16 ± 0.01 a |
. | ACTH (pg/mL) . | Corticosterone (ng/mL) . | Testosterone (ng/mL) . |
---|---|---|---|
(n = 10) | (n = 10) | (male, n = 5) | |
StarWT | 36 ± 6.8 | 42 ± 9.9 | 0.25 ± 0.03 |
StarKO TgWT | 23 ± 0.7 | 43 ± 7.1 | 0.28 ± 0.06 |
StarKO Tg-LowS194A | 390 ± 83 a | 19 ± 3.5 a | 0.15 ± 0.01 a |
StarKO | 343 ± 68 a | 15 ± 3.4 a | 0.16 ± 0.01 a |
The values were expressed as the mean ± SEM.
, P < .05. StarKO Tg-HighS194A mice were not available due to low fertility.
To further assess the mechanism of reduced steroid production, we measured mRNAs for the enzymes Cyp11a1 and Hsd3b2 common to the adrenal cortex and testis. Cyp11a1 transcripts were significantly reduced about 10-fold in adrenal tissue from StarKOTg-HighS194A, StarKOTg-LowS194A, and StarKO mice compared with StarKOTgWT animals, whereas Hsd3b2 transcripts were also reduced in these 3 groups of mice, but the difference did not reach statistical significance (Figure 3). The decreased Cyp11a1 mRNA expression despite elevated plasma ACTH might reflect secondary damage to the adrenocortical cells from StAR dysfunction, with resultant impairment of cAMP generation and transcription. In contrast, no significant differences in Cyp11a1 or Hsd3b2 expression were found in testis among these genotypes (Figure 3). The difference between adrenal and testis results might derive either from the small fraction of Leydig cells in total testis mass or from biological differences in the response to StAR dysfunction in these tissues.
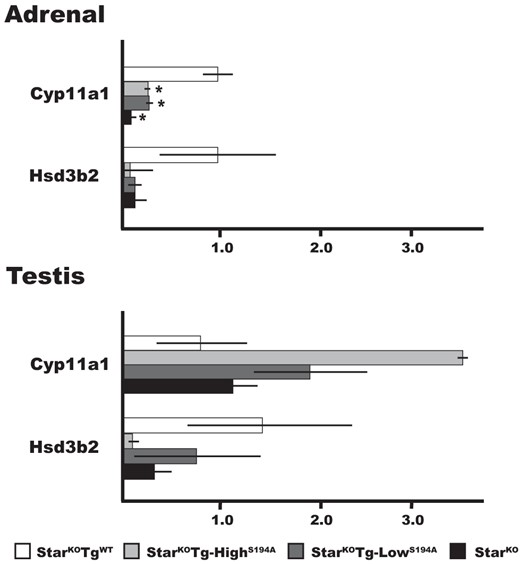
Expression of steroidgenic genes in the adrenal glands and testes. Total RNA from newborn mice was subjected to real-time RT-PCR analysis as described in Materials and Methods. The relative expression of Cyp11a1 and Hsd3b2 mRNAs for the indicated genotypes is shown: StarKOTgWT (n = 3), StarKOTg-HighS194A (n = 3), StarKOTg-LowS194A (n = 4), and StarKO (n = 4) mice. All values are normalized to expression levels in StarWTmice adrenals (1.0), and data are shown as median ± SEM. *, P < .05 relative to levels in StarWT adrenal tissue.
The StarKOTgS194A mice showed lipid accumulation in adrenal cortex and testes comparable to StarKO mice
To evaluate the accumulation of cholesterol esters in the adrenal cortex and testes from newborn mice of different genotypes, we stained these tissues with oil red O to identify lipid deposits. StarWT mice had minimal lipid deposits in adrenal cortex and testes, whereas the StarKO mice exhibited florid deposits in adrenal cortex and large deposits in the interstitial region of testes where the steroidogenic Leydig cells reside (Figure 4), as previously documented (7, 8). The StarKO animals rescued with the WT-Star transgene also contained minimal lipid deposits in the adrenal cortex and testes, comparable to StarWT mice, demonstrating complete rescue. In contrast, the adrenal cortex and testes of animals rescued with the S194A-Star transgene exhibited florid lipid deposits staining with oil red O, even in StarKOTg-HighS194A mice, in which S194A-Star is overexpressed. These results confirm that the S194A-StAR protein cannot support normal cholesterol transport and provide strong evidence that phosphorylation at S194 is required to sustain normal Star function.
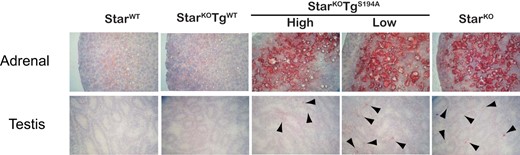
Oil red O staining of adrenal glands and testes from StarWT, StarKOTgWT, StarKOTgS194A (lines “High” and “Low”), and StarKO mice. Organs were harvested from newborn mice and processed as described in Materials and Methods. Shown are lipid deposits stained in adrenal gland (×400 magnification), and testis (×100 magnification) from mice of the indicated genotypes. Arrowheads indicate macroscopic lipid deposits stained in steroidogenic interstitial regions of the testes.
Discussion
Although genetic evidence in human patients with lipoid CAH and in StarKO mice established the crucial role of StAR in steroid biosynthesis, the precise mechanism of StAR-mediated cholesterol transfer in the mitochondria has not been fully defined in vivo (19, 20). To explore structure-function relationships for StAR in primary steroidogenic cells, we developed the transgene-rescue mouse model with StarKO mice. We validated this genetic add-back model by showing 1) the accurately matched endogenous expression of a Star/enhanced green fluorescent protein transgene in adrenal, testes, and ovary; 2) comparable Star transcription and translation in the adrenal and testes and responsiveness to their respective pituitary trophic hormones; and 3) normal phenotype with normal serum steroids in StarKO mice carrying WT Star transgene (StarKOTgWT) (15). In this context, the transgenic rescue strategy allows us to examine in vivo the role(s) of specific StAR residues and modifications, such as S194 phosphorylation on a background that lacks endogenous WT StAR, unlike previously studied systems (10–13). Previous studies of S194A/S195A-StAR using in vitro assays have demonstrated approximately 50% decreased function (12, 13), but these studies used COS-1 cells, which are not true steroidogenic cells and cannot demonstrate the evolution of cumulative cellular damage over time (15, 19–20). For human patients, genetic analyses revealed that compound heterozygosity of S195A/Q258X gives rise to classical LCAH, indirectly suggesting that S194/S195 is an important residue for normal StAR function in vivo (14).
In our study, S194A-Star protein failed to rescue postnatal adrenal or testicular steroidogenesis in StarKO mice, either when expressed in relatively normal amounts in StarKOTg-LowS194A mice or when expressed at high amounts from 5 copies of the transgene (21, 22) in StarKOTg-HighS194A mice (Figure 2, A and B). Raised plasma ACTH and low serum corticosterone and testosterone provide strong evidence for defective S194A-Star function in postnatal mice, although the testosterone values in all animals were unstimulated and thus near the lower limits of the assay (Table 2). Cyp11a1 transcripts were reduced in adrenal tissue of animals rescued with the S194A transgene, suggesting additional cellular damage and impaired cAMP generation secondary to StAR dysfunction (Figure 3). Altered expression of steroidogenic factor 1 (Sf1), other transcription factors, or other enzymes might also contribute to the adrenal and testicular pathology in these animals but was not assessed. Abundant oil red O staining of lipid deposits in the adrenal cortex and interstitial cells of the testes, the hallmark of LCAH, confirms the inability of S194A-Star to prevent cholesterol ester accumulation (Figure 4). Collectively, these findings indicate that phosphorylation-deficient S194A-Star might retain partial activity in model systems but fails to maintain normal steroid production in adrenal cortex and testes of postnatal mice. This interpretation might explain the discrepancies observed between our studies of mouse adrenal and testis and prior studies using transiently transfected cell lines. In both cases, however, important experimental caveats should temper conclusions derived from these studies.
In contrast to the profound defect in adrenal and gonadal steroid production in the postnatal mice, some evidence of fetal testosterone synthesis was observed, because one-third of the StarKOTg-LowS194A and 2 of 2 viable StarKOTg-HighS194A male mice were born with masculinized external genitalia, despite decreased serum testosterone levels at birth (Tables 1 and 2). The masculinization, which is never observed in StarKO males, implies a certain androgen effect during the critical early window of sexual development (23). The pathophysiology of LCAH is believed to follow a biphasic course, with low steroidogenesis initially from both StAR-independent and mutant StAR-dependent pathways, but with sustained tropic stimulation and cholesterol ester accumulation, additional cellular damage ensues, which causes complete absence of steroidogenesis.
With this background, we propose one possible explanation for our findings. The high transgene expression in StarKOTg-HighS194A mice appears to consistently sustain sufficient testosterone production to masculinize all of the males. In contrast, the low transgene expression in the StarKOTg-LowS194A mice might support testosterone production and prevent Leydig cell failure in only a fraction of the animals. In all S194A-Star transgenic mice, however, the second phase of damage occurs and abrogates all steroidogenesis in the adrenals and testes of postnatal animals. One caveat to our interpretation of the differences in the StarKOTg-LowS194A and StarKOTg-HighS194A lines is the small number of live male pups obtained from the StarKOTg-HighS194A line.
Previous studies found attenuated cytoplasmic stability of the S194A/S195A-StAR protein (11) and diminished intrinsic activities, including interactions with the mitochondrial membrane and/or undefined cofactor(s) (24, 25), yet preserved capacity to bind cholesterol in the C-terminal START-related lipid transfer domain (26–28), consistent with trace residual activities of S194A-StAR in this study. Our data suggest that S194/S195 StAR phosphorylation is not essential for cholesterol transfer per se but rather mediates a subsequent step of StAR function, which prevents cholesterol ester accumulation and the second phase of cellular damage observed in LCAH patients (6, 29) and StarKO mice (7, 8). This latter step remains obscure but might involve mitochondrial import of StAR, interaction with other proteins engaged in mitochondrial cholesterol mobilization, or activation of other intracellular cholesterol-trafficking mechanisms.
As shown in Figure 2C, adrenal StAR appears to be phosphorylated at sites other than S194/S195, as observed in StarKOTg-LowS194A mice continuously stimulated by trophic hormones. Another consensus sequence for PKA-mediated phosphorylation has been determined at S56 (S57 in human), which is conserved among most species and near the cleavage site for the mitochondrial targeting sequence (30). In vitro studies using nonsteroidogenic COS-1 cells showed that S56A/S57A StAR proteins demonstrate approximately 20% decreased StAR activity under cAMP stimulation (12, 13), indicating that phosphorylation at S56/S57, if it occurs, serves a relatively minor role in StAR activation. Moreover, recent studies revealed that S232 is another cAMP-dependent phosphorylation site by ERK1/2 to enhance StAR activity (31, 32). Intriguingly, the phosphorylation by ERK1/2 is independent of phosphorylation at other sites yet requires the presence of cholesterol, suggesting a functional role in StAR-mediated cholesterol transfer processes. It is possible that, in the absence of S194/S195 phosphorylation, S194A/S195A-StAR undergoes excess phosphorylation at other sites, which contributes to the pathology seen in the S194A-Star transgenic animals in our study.
Nevertheless, our studies used a single experimental paradigm in the mouse model, and our data are subject to alternative interpretations. The finding that male mice are inconsistently virilized might represent biological variation but also could suggest imperfections in gene deletion or expression. For these reasons, extrapolation of our results to other cell types and species should be applied cautiously. Further studies of the S194A/S195A-StAR proteins might provide insight to the termination of StAR action, the replenishment of mitochondrial cholesterol during steroidogenesis, and the pathophysiology of permanent adrenal and testicular damage in LCAH.
Acknowledgments
We dedicate this study to Dr Keith L Parker (Chief, Division of Endocrinology and Metabolism, Department of Internal Medicine, University of Texas Southwestern Medical Center, Dallas, TX), who was a dedicated mentor for our projects, a brilliant scientist, and a dear friend and leader in molecular steroidogenesis community, but who regretfully died on December 12, 2008. We thank Drs Nathan Bingham and Pancharatnam Jeyasuria for technical assistance of BAC recombination, Dr Dale Hales for providing the anti-StAR antiserum, and Drs Robert Hammer and John Ritter for helpful discussions and assistance in generating the transgenic mice.
This work was supported by National Institutes of Health Grant R01DK54028 (to Keith L. Parker).
Disclosure Summary: The authors have nothing to disclose.
Abbreviations
- BAC
bacterial artificial chromosome
- CV
coefficient of variation
- KO
knockout
- LCAH
lipoid congenital adrenal hyperplasia
- PKA
protein kinase A
- SDS
sodium dodecyl sulfate
- StAR
steroidogenic acute regulatory protein
- WT
wild-type.