-
PDF
- Split View
-
Views
-
Cite
Cite
Thomas Schlegel, Oliver Mirus, Arndt von Haeseler, Enrico Schleiff, The Tetratricopeptide Repeats of Receptors Involved in Protein Translocation across Membranes, Molecular Biology and Evolution, Volume 24, Issue 12, December 2007, Pages 2763–2774, https://doi.org/10.1093/molbev/msm211
- Share Icon Share
Abstract
Transport of polypeptides across membranes is a general and essential process in every cell. This process is utilized by molecular machines composed of soluble and membrane-inserted proteins. At least one component of the molecular transport machines present in different membranes contains a subunit with a domain composed of 3 tetratricopeptide repeat (TPR) motifs. These domains are important for protein–protein interaction, for example, recognition of chaperones. To understand the evolution of these TPR domain–containing receptors involved in protein translocation, we inferred their phylogenetic relationships. We show that the evolutionary rate of these TPR domains is reduced when compared with the remaining sequence. The reduction is explained by the interaction of the TPR domains with their substrates. Based on the TPR tree, we propose that Sec72 recognizes Hsp70 and that Tom34 recognizes Hsp90. The phylogeny can further be used to assign the localization of the Toc64 isoforms to mitochondria or chloroplasts. Our findings are discussed in the context of the evolutionary development of translocation systems with focus on the occurrence of Hsp70/Hsp90-recognizing TPR domains in these machineries.
Introduction
Protein translocation across membranes performed by molecular machines known as translocons is a fundamental process for each cell. Receptors as part of these machines recognizing either folded (Robinson and Bolhuis s; Xu and Massague 2004; Erdmann and Schliebs 2005) or unfolded (Rehling et al. 2004; Soll and Schleiff 2004; Osborne et al. 2005) proteins are common in all known translocation machineries (Wickner and Schekman 2005). The transport toward all cellular membranes depends on a specific signal within the precursor proteins (the proteins translocated across the membranes), which encodes for the organelle targeted and the assembly of cytosolic complexes (Rehling et al. 2004; Soll and Schleiff 2004; Osborne et al. 2005). The membrane-inserted receptor proteins are classified into 2 categories. One class recognizes the “cytosolic transporters” involved in precursor protein delivery, that is, chaperones, whereas receptors belonging to the other class recognize the precursor protein itself (Wickner and Schekman 2005). Interestingly, in the translocons at almost each membrane, at least one receptor containing tetratricopeptide repeat (TPR) motifs domain is present. Such domains are composed of 3 helix bundles (I, II, and III) with 2 helices (a and b) each. This domain is capped by a so-called solvation helix at the C-terminal end. The TPR motifs were first identified in the nuclear protein ssn6 (Schultz et al. 1990), the chromatin-associated replication protein CDC23 (Sikorski et al. 1990), and the protein required for mitotic chromosome disjunction, nuc2+ (Hirano et al. 1990). In recent years, the list of proteins carrying a TPR domain has grown steadily (Goebl and Yanagida 1991; Lamb et al. 1995; D'Andrea and Regan 2003).
In the translocons, the mitochondrial receptors Tom20 (Haucke et al. 1996), Tom70 (Haucke et al. 1996; Young et al. 2003), and Tom34 (Nutall et al. 1997; Young et al. 1998; Chewawiwat et al. 1999; Yang and Weiner 2002); the peroxisomal receptor Pex5 (Gatto et al. 2000; Kumar et al. 2001); the component of the translocon of the endoplasmic reticulum Sec72 (Feldheim and Schekman 1994; Ponting 2000); and the chloroplast translocon component Toc64 (Sohrt and Soll 2000) contain TPR domains. Tom70, Tom34, and Toc64 contain a specific form of the TPR domain, namely, a dicarboxylate clamp-type TPR domain, which was first found in the Hsp70/Hsp90-organizing protein Hop (Scheufler et al. 2000). Hop contains 3 TPR domains (HopTPR1, HopTPR2a, and HopTPR2b), which are structurally (with the exception of HopTPR2b) and functionally analyzed (Scheufler et al. 2000). HopTPR1 recognizes Hsp70, HopTPR2a interacts with Hsp90, and HopTPR2b is proposed to influence the kinetics of Hsp70–Hop–Hsp90 complex formation (Brinker et al. 2002).
Like in Hop (Brinker et al. 2002), the clamp-type domain of Tom70 and Toc64 recognizes the C-terminus of chaperones (Young et al. 2003; Fan et al. 2006; Qbadou et al. 2006). In contrast, the TPR domain of Pex5 recognizes the C-terminal peroxisomal targeting signal 1 of the precursor protein (Gatto et al. 2000). The interaction partner of Sec72 has not been identified yet, even though the association with a protein of unknown function, encoded by YLR301w, was reported (Willer et al. 2003). For Pex5 (Erdmann and Schliebs 2005) as well as Tom34 (Yang and Weiner 2002), a cytosolic localization was suggested. Based on yeast 2-hybrid experiments, an interaction of Tom34 with 2 ATPase-related proteins lysosomal H (+)-transporting ATPase member M (ATP6M) and valosin-containing protein (VCP) was reported (Yang and Weiner 2002). Additionally, an interaction between one of the TPR domains of Tom34 and Hsp90 was observed (Young et al. 1998). Thus, the exact function of Tom34 in protein translocation remains elusive.
Hence, having a TPR domain–containing receptor in each of the translocons of the different membrane systems raises the question if and how the translocase subunits containing a TPR domain evolved from a common ancestor. To this end, the analysis of the occurrence of these domains might be used as a tool to understand the evolution of translocation systems per se. We therefore analyzed the TPR domains of the 5 receptor families (Tom70, Tom34, Pex5, Sec72, and Toc64) putatively involved in protein translocation with respect to their relation to each other. To assign possible functional properties, we compared their TPR domains with TPR domains from chaperone-organizing proteins of the Hop family and with other cochaperones. The consequences of our results for the functional and phylogenetic understanding of the TPR domains and the TPR domain–containing receptor proteins are discussed.
Materials and Methods
Sequence Selection
Sequences were collected by Blast, and the relation to the seed sequence was controlled by a reversed Blast (http://www.ncbi.nlm.nih.gov; Altschul et al. 1997). More specifically, Toc64 from Pisum sativum (Sohrt and Soll 2000), Sec72 from Saccharomyces cerevisiae (Feldheim and Schekman 1994), Pex5 from Homo sapiens (Dodt et al. 1995) and S. cerevisiae (van der Leij et al. 1993), Tom70 from S. cerevisiae (Hase et al. 1983), Tom34 from H. sapiens (Nuttall et al. 1997), and Hop from H. sapiens (Honore et al. 1992) and S. cerevisiae (Nicolet and Craig 1989) served as initial query sequences. In addition, sequences were downloaded from http://www.jgi.doe.gov/. These sequences were produced by the US Department of Energy Joint Genome Institute. To extract TPR domains, we used previous assignments of the TPR domains for hsHop (Scheufler et al. 2000), hsPex5 (Gatto et al. 2000), scSec72 (Ponting 2000), and hsTom70 (Young et al. 2003). From Pex5, TPR motif 1–3 (here termed domain 1) and TPR motif 5–7 (here termed domain 2) were excised. The TPR domain of Toc64 or Tom34 was assigned according to alignments to hsTom70 and hsHop, respectively (Young et al. 1998; Qbadou et al. 2006). In addition, we dissected the TPRs of Hop into TPR1, TPR2a, and TPR2b.
Tree Reconstruction
Sequences were aligned with the program MAFFT (Katoh et al. 2005) version 5.861, resulting in multiple sequence alignments for Hop, Toc64, Tom34, Tom70, Pex5, and Sec72 family. The alignments were split in the subalignments containing the TPR domains and the rest. Thus, we obtained for each receptor a nonTPR subalignment and 1–3 TPR alignments, respectively (see also table 1). Moreover, we created a sequence alignment from all TPR sequences. IQPNNI (Vinh and von Haeseler 2004) was used to reconstruct a maximum likelihood phylogeny, assuming the Whelan and Goldman model (Whelan and Goldman 2001) and constant rates across sites (supplementary fig. 1, Supplementary Material online).
Protein | Domaina | Sequences | TBL |
Sec72 | Entire | 16 | 8.5 |
−TPR | 16 | 9.7 | |
TPR | 16 | 7.5 | |
Toc64 | Entire | 14 | 4.2 |
−TPR | 14 | 4.5 | |
TPR | 14 | 2.8 | |
TPR | 28b | 4.7 | |
Tom70 | Entire | 38 | 12.4 |
−TPR | 38 | 13.16 | |
TPR | 38 | 8.99 | |
Tom34 | Entire | 8 | 1.8 |
−TPR | 8 | 1.97 | |
TPR1 | 8 | 1.0 | |
TPR2 | 8 | 1.6 | |
TPR | 8 | 1 | |
Pex5 | Entire | 55 | 20.5 |
−TPR | 55 | 27.9 | |
TPR1 | 55 | 11 | |
TPR2 | 55 | 9.1 | |
TPR | 55 | 12 | |
Hop | Entire | 57 | 14.9 (13.5)c |
−TPR | 57 | 19.1 | |
TPR1 | 50 | 13.1 | |
TPR2a | 57 | 13.3 | |
TPR2b | 57 | 11.8 |
Protein | Domaina | Sequences | TBL |
Sec72 | Entire | 16 | 8.5 |
−TPR | 16 | 9.7 | |
TPR | 16 | 7.5 | |
Toc64 | Entire | 14 | 4.2 |
−TPR | 14 | 4.5 | |
TPR | 14 | 2.8 | |
TPR | 28b | 4.7 | |
Tom70 | Entire | 38 | 12.4 |
−TPR | 38 | 13.16 | |
TPR | 38 | 8.99 | |
Tom34 | Entire | 8 | 1.8 |
−TPR | 8 | 1.97 | |
TPR1 | 8 | 1.0 | |
TPR2 | 8 | 1.6 | |
TPR | 8 | 1 | |
Pex5 | Entire | 55 | 20.5 |
−TPR | 55 | 27.9 | |
TPR1 | 55 | 11 | |
TPR2 | 55 | 9.1 | |
TPR | 55 | 12 | |
Hop | Entire | 57 | 14.9 (13.5)c |
−TPR | 57 | 19.1 | |
TPR1 | 50 | 13.1 | |
TPR2a | 57 | 13.3 | |
TPR2b | 57 | 11.8 |
Entire, entire sequence of protein; −TPR, entire sequence except the analyzed TPR domains; TPR, the TPR sequence.
The total branch length for the tree including the EST sequences.
In brackets, the total branch length for the 50 sequences analyzed for TPR1.
Protein | Domaina | Sequences | TBL |
Sec72 | Entire | 16 | 8.5 |
−TPR | 16 | 9.7 | |
TPR | 16 | 7.5 | |
Toc64 | Entire | 14 | 4.2 |
−TPR | 14 | 4.5 | |
TPR | 14 | 2.8 | |
TPR | 28b | 4.7 | |
Tom70 | Entire | 38 | 12.4 |
−TPR | 38 | 13.16 | |
TPR | 38 | 8.99 | |
Tom34 | Entire | 8 | 1.8 |
−TPR | 8 | 1.97 | |
TPR1 | 8 | 1.0 | |
TPR2 | 8 | 1.6 | |
TPR | 8 | 1 | |
Pex5 | Entire | 55 | 20.5 |
−TPR | 55 | 27.9 | |
TPR1 | 55 | 11 | |
TPR2 | 55 | 9.1 | |
TPR | 55 | 12 | |
Hop | Entire | 57 | 14.9 (13.5)c |
−TPR | 57 | 19.1 | |
TPR1 | 50 | 13.1 | |
TPR2a | 57 | 13.3 | |
TPR2b | 57 | 11.8 |
Protein | Domaina | Sequences | TBL |
Sec72 | Entire | 16 | 8.5 |
−TPR | 16 | 9.7 | |
TPR | 16 | 7.5 | |
Toc64 | Entire | 14 | 4.2 |
−TPR | 14 | 4.5 | |
TPR | 14 | 2.8 | |
TPR | 28b | 4.7 | |
Tom70 | Entire | 38 | 12.4 |
−TPR | 38 | 13.16 | |
TPR | 38 | 8.99 | |
Tom34 | Entire | 8 | 1.8 |
−TPR | 8 | 1.97 | |
TPR1 | 8 | 1.0 | |
TPR2 | 8 | 1.6 | |
TPR | 8 | 1 | |
Pex5 | Entire | 55 | 20.5 |
−TPR | 55 | 27.9 | |
TPR1 | 55 | 11 | |
TPR2 | 55 | 9.1 | |
TPR | 55 | 12 | |
Hop | Entire | 57 | 14.9 (13.5)c |
−TPR | 57 | 19.1 | |
TPR1 | 50 | 13.1 | |
TPR2a | 57 | 13.3 | |
TPR2b | 57 | 11.8 |
Entire, entire sequence of protein; −TPR, entire sequence except the analyzed TPR domains; TPR, the TPR sequence.
The total branch length for the tree including the EST sequences.
In brackets, the total branch length for the 50 sequences analyzed for TPR1.
Comparative Analysis of the TPR and NonTPR domains
To compare differences in the tree length (Minh et al. 2006) between the nonTPR and corresponding TPR domains, we used a simulation. The sites of the full alignment were permuted to generate 1,000 alignments. From each alignment, we extracted the 2 subalignments, where the length of the first alignment equals that of the TPR alignment and the length of the second alignment equals that of the corresponding nonTPR domain. We use the tree topology of the full alignment to compute the tree length for the random TPR and nonTPR alignments with IQPNNI (Vinh and von Haeseler 2004). Finally, we computed the tree length of the TPR domain and the nonTPR domain of the original data.
Homology Modeling
The Phyre server (http://www.sbg.bio.ic.ac.uk/∼phyre/), the successor of 3DPSSM (Kelley et al. 2000), was used to find suitable templates for modeling of Sec72. The crystal structure of bovine cyclophilin 40 (1IHG; Taylor et al. 2001) was selected as a template for modeling the TPR domain of Sec72 with Modeller (Sali and Blundell 1993; Fiser et al. 2000) v8.2. The homology model was refined with Yasara's Yamber2 force field (http://www.yasara.org/; Krieger et al. 2004).
Results
Phylogenetic Analysis of the TPR Domain–Containing Proteins Involved in Protein Translocation
We have screened the databases for homologous sequences to the 5 known proteins containing a TPR domain involved in protein translocation. Interestingly, Sec72 homologues were only identified in fungi (fig. 1a). Toc64 is only found in plant species (fig. 1b). Tom70 homologues were not found in the group of green plants (fig. 2a), which is in accordance with the fact that an isoform of Toc64 functionally replaces Tom70 in plant mitochondria (Chew et al. 2004). In turn, Tom34, containing 2 TPR domains connected by a conserved linker, was only found in higher metazoa (fig. 2b). Therefore, the Pex5 receptor family is the only one identified in all eukaryotic species (fig. 2c). In addition to the proteins involved in protein translocation across membranes, the protein family organizing Hsp70/90 interactions (Hop) was studied. However, we included in our analysis only sequences of Hop homologues containing the 3 TPR domains.
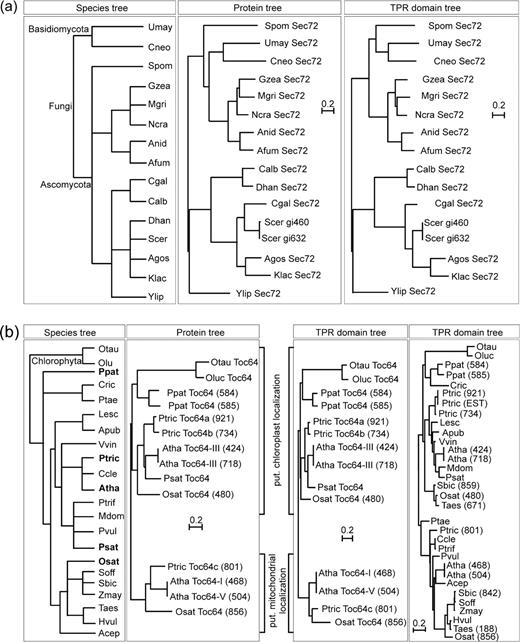
Phylogenetic analysis of Sec72 and Toc64 sequences. Shown are the taxonomy trees according to National Center for Biotechnology Information (NCBI) (http://www.ncbi.nlm.nih.gov/; left; please note that in this case, the branch length does not reflect a phylogenetic distance), the phylogenetic trees of the entire protein sequence (middle), or the TPR domain (right) of the identified sequences coding for Sec72 (a) or Toc64 (b). For Toc64, a fourth tree is included containing identified EST sequences (see supplementary table 3, Supplementary Material online). Please note that in (b) only for species written in bold (left), entire protein sequences were available. For species abbreviation, see supplementary table 1 (Supplementary Material online). To discriminate between multiple isoforms, the first 3 digits of the gene identilication code are shown for Sec72 isoforms or the last 3 digits of the identification code listed in supplementary tables 2 and 3 (Supplementary Material online) are given for Toc64 isoforms. The scale bar given for each tree individually indicates the number of substitutions per site. Trees are rooted to match the distribution of the NCBI taxonomy.
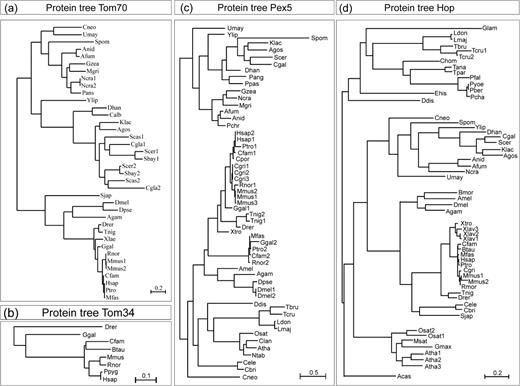
Phylogenetic trees of Pex5, Tom70, and Hop. Shown are the maximum likelihood trees of the entire protein sequence of the identified sequences coding for Tom70 (a), Tom34 (b), Pex5 (c), or Hop (d). The scale bar—given for each tree individually—indicates the estimated number of substitutions per site. Please note that the scale bar length differs between the subfigures. Species abbreviations are listed in supplementary table 1 (Supplementary Material online). The phylogenetic trees of the sequences coding for domains of the proteins are given in supplementary figure 1 (Supplementary Material online).
First, we analyzed the phylogenetic relation of sequences encoding for (putative) receptor proteins involved in protein translocation (figs. 1and 2; supplementary fig. 1, Supplementary Material online). The placement of Tom70 sequences in the tree confirmed the previously reported distribution and supports the notion that a gene duplication has occurred at the base of the Saccharomyces clade (Kurtzman and Robnett 2003; Chan et al. 2006). For Toc64, we obtained 2 subtrees reflecting the different localizations of the protein in the cell, where each subtree follows the species tree (fig. 1b). The top subtree in figure 1b comprises the plastid-localized proteins and the bottom subtree the mitochondrial proteins (Chew et al. 2004; Chan et al. 2006).
Next, we excised the TPR domains from the receptor proteins or Hop sequences (see Materials and Methods) and inferred their phylogeny. We aimed to determine how the described interaction of the TPR domain with other proteins affected their evolutionary history. Again, the trees inferred from the TPR domains agree by and large with the trees of the entire receptor sequences (fig. 1; supplementary fig. 1, Supplementary Material online). Comparing the total amount of evolution as measured by the tree length, substantial differences are observed. Table 1 displays the tree length for the entire sequences, the TPR domains and the nonTPR sequences. A nonTPR sequence in this respect covers the remaining sequence without the here analyzed TPR domain and might thereby include other nonclamp-type TPR domains as in case of Tom70. The TPR domain trees are consistently shorter than the entire and the nonTPR trees. Thus, evolution is more conserved in the TPR domain. To statistically support the observation, we permuted the sites of the alignment. For each permutated alignment, we computed the tree length of the sequence regions assigned as TPR domain and nonTPR, respectively. This approach warrants that the underlying tree topology remains constant, whereas the tree lengths of the subalignments, that is, the random alignment columns assigned to the TPR region, change due to the permutation of the full alignment. Figure 3 displays the resulting random tree length distributions for the 6 receptor protein families. To facilitate a comparison of the different TPR domains, the tree lengths of the randomized alignments were normalized by the corresponding lengths from the original alignments. Thus, the data points are always located at the coordinate (1.0, 1.0).
![Significance analysis for domain-based branch length differences. The length of the phylogenetic tree (tree length [TL]) of the TPR and the nonTPR domains was calculated for the original sequence and for 1,000 permuted alignments (see Materials and Methods). The TL of permuted alignments was normalized according to the tree length for the original sequence. Each dot indicates a value from one simulation and the ellipse the 95% confidence region. Left top: gray circle and solid lines, TPR1; gray square and long dashed line, TPR2a; white diamond and short dashed line,TPR2b; Right top: gray circle and solid lines, Tom34TPR; gray square and dashed line, Tom34TPR2; left bottom: gray circle and solid lines, Pex5-TPR1; gray circle and dashed line, Pex5-TPR2; black circle and gray line, Toc64TPR; right bottom: gray circle and solid lines, Tom70TPR; black circle and gray line, Sec72TPR. The original tree length for each receptor is indicated by an enlarged black dot.](https://oup.silverchair-cdn.com/oup/backfile/Content_public/Journal/mbe/24/12/10.1093_molbev_msm211/2/m_molbiolevolmsm211f03_ht.jpeg?Expires=1750214908&Signature=36S3j5IC1i2SvP037Wzy71fU~clNL3QaFtIk4KXt6CrnXGyLFAdZMQU0CoWDdY9gumCF3PijLxT5RMP10j4a0o11IYHxh~y3DSypGp9kgRIXI2wDUOLXRFnS625h6IKbV-9IFqPpksIGngS~Sd9qb-RlDZjWBJPOSM~4pzWU59t385-B5WIehRBiIYunL2r78qY8h5jAD5BmbNPZ8uqtL4tcVfN9rQoMH0~qb4LpCWHPYhLhjUZl3U6sk4f9YUnAFU3c46Y04cF2fJ9FXpMCVXWFe0d8wd6zhdZEplrjv5iz4BHSjl3FTY0tov2QeI8QkVbzzNIHwwKx8DOcLjIBoA__&Key-Pair-Id=APKAIE5G5CRDK6RD3PGA)
Significance analysis for domain-based branch length differences. The length of the phylogenetic tree (tree length [TL]) of the TPR and the nonTPR domains was calculated for the original sequence and for 1,000 permuted alignments (see Materials and Methods). The TL of permuted alignments was normalized according to the tree length for the original sequence. Each dot indicates a value from one simulation and the ellipse the 95% confidence region. Left top: gray circle and solid lines, TPR1; gray square and long dashed line, TPR2a; white diamond and short dashed line,TPR2b; Right top: gray circle and solid lines, Tom34TPR; gray square and dashed line, Tom34TPR2; left bottom: gray circle and solid lines, Pex5-TPR1; gray circle and dashed line, Pex5-TPR2; black circle and gray line, Toc64TPR; right bottom: gray circle and solid lines, Tom70TPR; black circle and gray line, Sec72TPR. The original tree length for each receptor is indicated by an enlarged black dot.
For 8 out of 10 TPR domains (except Sec72 and HopTPR1), the permutations always lead to longer TPR and shorter nonTPR tree length, as indicated by the location of the simulated distributions. Furthermore, the probability to observe the original tree lengths in the simulated distribution is well below the 5% level for all receptor proteins. For example, the random tree lengths of the TPR domain of Toc64 are 1.16–1.91 times longer than the undisturbed domain. Similarly, we observe a 1.03–1.16 times reduction in tree length for the nonTPR region when compared with the original sequence (fig. 3). In summary, the reduced evolutionary rate of the TPR domain as compared with the nonTPR region is significant and a hallmark of the evolutionary constraints acting on TPR domains.
The General Phylogeny of the TPR Domains
The current functional models for Tom34 (Young et al. 1998), Tom70 (Young et al. 2003), and Toc64 (Qbadou et al. 2006) suggest that their TPR domain recognizes chaperones bound to the precursor protein rather than the targeted precursor protein itself. In turn, Pex5 directly recognizes the C-terminal portion of the incoming precursor (Erdmann and Schliebs 2005). We investigated, how these functional differences are reflected by the phylogeny of TPR domains. To this end, we inferred a maximum likelihood phylogenetic tree with 570 sequences of TPR domains of proteins involved either in protein translocation across membranes or in organization of chaperone complexes. Figure 4 displays the phylogenetic relationship of the TPR domains.
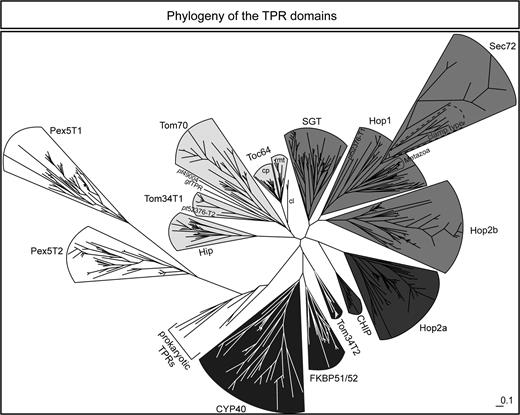
The TPR domains are branched according to the receptor class. All TPR domain sequences of the proteins involved in precursor protein transport (Sec72, Tom70, Toc64, Tom34, and Pex5) as well as TPR sequences of the cochaperones FKBP51/52, Cyp40, CHIP, Hip, SGT, Hop, and prokaryotic TPR–containing proteins were aligned with MAFFT and a phylogenetic tree was constructed with IQPNNI. The figure shows the maximum likelihood tree after 30,000 iterations. mt, mitochondrial subtree of Toc64; cp, chloroplastic subtree of Toc64; cl, the 2 sequences found in Chlorophyta with homology to Toc64; clamp type, cluster of the Sec72 sequences with a conserved clamp-type TPR domain. Each of the 5 groups is marked by different shades from white to black. Pex5: white, Tom34T1/Tom70/Toc64: light gray, SGT/Hop1/Hop2b/Sec72TPR: medium gray, Hop2a/CHIP: dark gray, Tom34T2/Cyp40/FKBP51/52: black.
The TPR domains form 2 major subtrees according to their interaction partners (fig. 4, white vs., gray-scaled groups). The white subtree contains the Pex5 proteins that interact directly with the precursor proteins, and the other comprises the TPR domains that recognize Hsp90 or Hsp70. The latter subtree is further split into 4 groups. Interestingly, in the black subtree, the Hsp90-interacting TPR domains of FKBP51/52 (e.g., Sinars et al. 2003; Denny et al. 2005) and CYP40 (e.g., Taylor et al. 2001; Carrello et al. 2004) are pooled with the C-terminal TPR domain of Tom34 (fig. 4, black) distinct from the dark gray Hop2a cluster. The light gray subtree holds the proteins that are involved in protein translocation including Tom34TPR1, Tom70, and Toc64, for which the separation into TPR domains of chloroplastic and mitochondrial localized proteins is reproduced (fig. 4, see clade cp—chloroplasts vs., mt—mitochondria, Toc64). However, also Hip, containing a nonclamp-type TPR domain interacting with Hsc70 (Hohfeld et al. 1995), is part of this subtree and forms its basis. The dark gray subtree is composed of the Hsp90-interacting TPR domain of Hop (fig. 4, dark gray) and the TPR domain of Chip, initially identified as an Hsp70-binding protein (Ballinger et al. 1999). The clustering of ChipTPR with Hop2a supports observations of an interaction between Chip and Hsp90 (Connell et al. 2001). Hop1, Hop2b, Sec72TPR, and small glutamins-righ protein (SGT-TPR) form the fourth group. The branching of Hop2b with the Hsp70-recognizing Hop1 is in line with recent results indicating an Hsp70–Hop2b interaction (Carrigan et al. 2004; Flom et al. 2007). Remarkably, the Sec72TPR domain clusters together with the Hsp70-recognizing TPR domains of Hop (Hop1 and Hop2b) and SGT (Angeletti et al. 2002). In more detail, Sec72TPR groups with Hop1 of metazoa. This might point to an appearance of Sec72 after metazoa and fungi split roughly 965 MYA (Doolittle et al. 1996). Hence, it might have emerged from an ancestor of Hop found at present in metazoa and fungi. Furthermore, the Sec72TPR sequences from Saccharomycetales are split into 2 subgroups. One subgroup contains a conserved clamp TPR domain, whereas the other one does not. We observed a larger distance of the nonclamp-type Sec72TPR domains from the Hop TPRs than for the clamp-type TPR domains (fig. 4). Hence, it remains to be established that the selected Sec72 sequences code for proteins with similar function.
Interestingly, the recently sequenced proteins from the cryptophyte Guillardia theta (gtTPR; supplementary fig. 4, Supplementary Material online) and the heterokontophyte Phaeodactylum tricornutum (pt49004 and pt52376) cluster with the Tom and the Toc family. More specifically, gtTPR and pt49004TPR group with Tom34's N-terminal TPR domain (Tom34TPR1). The protein pt52376 of P. tricornutum contains 2 TPR domains. Its second TPR domain clusters within the tree of Tom34's N-terminal TPR domain (not specifically indicated in fig. 4). The first TPR domain groups with the Hsp70-recognizing TPRs of Hop. The TPR domain of the ciliate Nyctotherus ovalis sequence (Boxma et al. 2005) is closely related to Tom34TPR2 (supplementary fig. 1d, Supplementary Material online; not specifically assigned in fig. 4), but the expressed sequence tag (EST) reported does not include the N-terminal TPR domain. Thus, both phylogenetic positions of the TPRs from the cryptophyte, heterokontophyte, and the ciliate suggest that the identified sequences participate in protein translocation and might be components of the mitochondrial import machinery.
Summarizing, the phylogenetic trees show a clear split into a mitochondrial and plastidic Toc64 subtree (figs. 1and 4). All clamp TPR domains exhibit a slower evolutionary rate when compared with the remaining sequence (table 1 and fig. 3). On the one hand, this observation could suggest that the TPR domain was optimized for its function early in evolution. On the other hand, its interaction with the rather conserved motif at the C-terminus of the chaperones might have influenced the evolutionary rate. Finally, we classify Sec72 as an Hsp70-recognizing protein. The new sequences from G. theta, P. tricornutum, and N. ovalis fall into the class of Tom34-like proteins opening the discussion of their evolution (see Discussion). Obviously, all 3 conclusions have to be supported by additional experiments.
Discussion
The TPR Domain–Containing Receptors
The analyzed TPR domains of the receptor proteins involved in precursor protein translocation participate in 3 types of interactions. First, they recognize precursor proteins, for which the most relevant experimental data are based on the crystal structure of Pex5 (Gatto et al. 2000; Erdmann and Schliebs 2005). The second and third type of interaction is the recognition of the chaperones Hsp70 or Hsp90, respectively. Here, experimental data are available for Tom34 (Young et al. 1998), Tom70 (Young et al. 2003; Fan et al. 2006), and Toc64 (Qbadou et al. 2006). However, the protein, studied best, is Hop recognizing both Hsp90 and Hsp70 (Young et al. 2004).
We analyzed the phylogenetic relationship of receptor units of the different translocons containing a TPR domain. A comparison of the trees inferred from TPR and nonTPR regions revealed that the TPR domains evolved significantly slower than the remaining sequence (table 1 and figs. 1–3). This can be explained as follows. The interactions of proteins with other components are also reflected in the selection acting on them. It was observed that the higher the number of interactions a protein has with other molecules the slower it evolves. Proteins that act in complexes are on average more constrained than others (Aris-Brosou 2005). We observe the same but on a subsequence level, which documents that the TPR domain of the proteins investigated is the major module for protein interaction within the sequence. Additionally, analyzing the TPR motifs themselves, a similar result was obtained for the concave ligand-binding surface and the opposite convex surface of the TPR domain. (Magliery and Regan 2004, 2005) observed while analyzing all known TPR sequences that the convex surface evolves randomly, whereas the concave surface with respect to constrains enforced by the ligands.
However, for Toc64, Tom70, Sec72, and Tom34, the difference between TPR and nonTPR domain evolution is not as drastic as seen for Pex5 (fig. 3 and table 1). This might be explained by a further limitation of the acceptance of evolutionary changes within the TPR domains of Pex5 by their coaction or as a result of the different substrates (PTS1 signal–containing proteins). The PTS1 signals are defined by a C-terminal canonical sequence of only 3 amino acids (SKL; e.g., Lazarow 2003). Analysis of the crystal structure of Pex5 (1FCH; Gatto et al. 2000) shows that the N-terminal TPR domain (Pex5 TPR1) contributes roughly half as many amino acids as Pex5-TPR2 to bind the C-terminal PTS1 signal of the substrate. This is reflected by a shorter tree length of the Pex5 TPR2 tree, if compared with the tree length of the Pex5 TPR1 tree (table 1 and fig. 3), again supporting, that substrate recognition is the dominating evolutionary constraint.
The Toc64 family
Recently, 3 isoforms of Toc64 were described (Jackson-Constan and Keegstra 2001; Chew et al. 2004). Whereas the first isoform was identified to behave as a typical amidase (Pollmann et al. 2003, 2006), the second isoform is a chloroplastic import receptor (Toc64-III; Chew et al. 2004), and the third is a mitochondrial localized import receptor (Toc64-V; Chew et al. 2004; Chan et al. 2006). The distinct localizations in different membranes and therefore the assembly with different interaction partners of the latter 2 isoforms enforced different developments resulting in a separate clustering of the mitochondrial and plastidic sequences (figs. 1 and 4). This separation was obtained for the entire sequence as well as for the TPR domain only. Within a subtree, the phylogeny represents the expected species tree (compare left tree in fig. 1b to the others). Thus, we can assign a putative localization of protein sequences from EST projects (supplementary table 3 and fig. 1, Supplementary Materials online) based on their clustering with already characterized proteins.
Functional Consequences for Tom34 and Sec72
We aimed to define possible functions of the 2 remaining receptors Sec72 (Feldheim and Schekman 1994; Ponting 2000) and Tom34 (Nuttall et al. 1997; Young et al. 1998; Chewawiwat et al. 1999; Yang and Weiner 2002). In the phylogenetic tree, the sequences of the TPR domains of both protein families cluster with the sequences carrying the Hop motifs but not with that of precursor protein recognizing Pex5 (fig. 4, white). The C-terminal TPR domain of Tom34 (TPR2) branches with the Hsp90-recognizing CYP40 and FKBP51/52 (fig. 4, black). This branching is experimentally supported because the C-terminal TPR domain of Tom34 was found to interact with Hsp90 (Young et al. 1998). The N-terminal TPR domain of Tom34 (TPR1) clusters together with Toc64 and Tom70 suggesting an interaction with Hsp90 as well. Hence, the presence of Tom34, another Hsp90-recognizing protein, points to a higher degree of regulation of mitochondrial protein translocation in Euteleostomi than in, for example, fungi.
The TPR domain of Sec72 shows features of the Hsp70-recognizing HopTPR1, as indicated by the clustering with the HopTPR1 of metazoa (fig. 4). Hence, Sec72 might function as a cytosolically exposed (Willer et al. 2003) Hsp70-recognizing element of the Sec translocon. To further support a putative Hsp70 recognition by Sec72, we extracted alignments of Hsp90- (FKBP51/52, Cyp40, and Hop2a) and Hsp70-recognizing (Hop1 and SGT) TPR domains from the alignment used for calculating the “global” phylogenetic tree (fig. 4). We composed a combined profile (a matrix of the frequency) of each amino acid to occur in Hsp70- or Hsp90-binding TPR domains by subtracting the Hsp70-binding TPR profile from that of the Hsp90-binding TPRs. This matrix was superimposed (supplementary fig. 2, Supplementary Material online) on the amino acid frequency determined for each position of the Sec72TPR.
By this analysis, it appears that most positions in the Sec72TPR facing the chaperone-binding pocket are not occupied by amino acids specific for Hsp90 or Hsp70 binding, respectively (fig. 5a–c; yellow). The other positions are rather uniformly distributed between Hsp90 and Hsp70 selecting amino acids (red/orange vs., cyan/blue). Focusing on the positions previously identified to be involved in chaperone recognition (fig. 5c; Scheufler et al. 2000), we obtained that position 117 in scSec72 (Hop1:49 and Hop2a:270) is almost equally occupied by either leucine (typical for Hsp90) or methionine. For the latter, we observed no significant difference between Hsp70- and Hsp90-binding TPR domains. In this connection, it is important to iterate that the dicarboxylate clamp motif is only conserved in roughly half of the Sec72TPR domains. Leucine at position 117 is only found in those Sec72 sequences without a conserved clamp-type motif. Additionally, introducing an alanine instead of leucine at this position leads to a significantly lower Hsp70 than Hsp90-binding ability for Cyp40 in vitro (Carrello et al. 2004). At position 69 in scSec72 either a phenylalanine or a tyrosine is present in roughly two-thirds of the sequences. Although these amino acids at this position are typical for Hsp90-binding properties, it could be shown for Cyp40 that this position is more important for Hsp70 than Hsp90 interaction in vitro (Carrello et al. 2004). By this, the positioning of the amino acids specific for the Hsp70- or Hsp90-recognizing TPR within the Sec72TPR motif further supports an Hsp70 interaction (fig. 5).
![Comparison of amino acid conservation between Toc64TPRs and the HopTPRs and Sec72. In (a–c), the homology model of Sec72TPR of Saccharomyces cerevisiae is shown. (a) represents the view into the chaperone-binding pocket, and in (b), the model is rotated by 180° around the y axis. From a probability matrix representing for each amino acid the probability to occur in Hsp90- and of Hsp70-binding TPR domains (values in the range [−1.0, 1.0]; values <0 denote Hsp70, and values >0 an Hsp90 preference), the value for the amino acid with the highest frequency in a multiple sequence alignment of Sec72TPR was extracted for every position. Subsequently, the structure is colored by the significance of a residue to define an Hsp90- or Hsp70-binding TPR domain ([−1,−0.5] blue, [−0.5,−0.1] cyan, [−0.1, 0.1] yellow, [0.1, 0.4] orange, [0.4, 1] red). The procedure is described in more detail in supplementary figure 5 (Supplementary Material online). In (c), the same view as in (a) is shown, but only amino acids involved in chaperone binding (Scheufler et al. 2000) are colored. Molecular graphics created with YASARA (www.yasara.org) and PovRay (www.povray.org).](https://oup.silverchair-cdn.com/oup/backfile/Content_public/Journal/mbe/24/12/10.1093_molbev_msm211/2/m_molbiolevolmsm211f05_4c.jpeg?Expires=1750214908&Signature=JVcc7WE3GYzuE4Iuw9BHEZLS8uU0jMs9fjGmRxxr29s1KUTjg-m1KagfYlmQjawCo~76MF9QjMJeFmu4W1v9a6~bNaI8vSbFbRBSzN7-Ig0Y736LMzzFPFEIokDxwiphGqUrBTGukeSlCTxg-rJyJUUtAa9iUGAxt4ovDhuYsHo8tVEQMsuDHW59y2A42mnQQgCw3lFZ2krJXbcE9rDbPrCftIB~WMXMEA0cwzfDvUheCdgJelT8kFerolVo2UFs-IqwakJ4rBf9fFY-HZW0jo0YtsCIc-BoHklgnqhMEmN2nptcJCzhsTfd9~iitUO-LcJUTPzzUdL8i48BL~9C3g__&Key-Pair-Id=APKAIE5G5CRDK6RD3PGA)
Comparison of amino acid conservation between Toc64TPRs and the HopTPRs and Sec72. In (a–c), the homology model of Sec72TPR of Saccharomyces cerevisiae is shown. (a) represents the view into the chaperone-binding pocket, and in (b), the model is rotated by 180° around the y axis. From a probability matrix representing for each amino acid the probability to occur in Hsp90- and of Hsp70-binding TPR domains (values in the range [−1.0, 1.0]; values <0 denote Hsp70, and values >0 an Hsp90 preference), the value for the amino acid with the highest frequency in a multiple sequence alignment of Sec72TPR was extracted for every position. Subsequently, the structure is colored by the significance of a residue to define an Hsp90- or Hsp70-binding TPR domain ([−1,−0.5] blue, [−0.5,−0.1] cyan, [−0.1, 0.1] yellow, [0.1, 0.4] orange, [0.4, 1] red). The procedure is described in more detail in supplementary figure 5 (Supplementary Material online). In (c), the same view as in (a) is shown, but only amino acids involved in chaperone binding (Scheufler et al. 2000) are colored. Molecular graphics created with YASARA (www.yasara.org) and PovRay (www.povray.org).
Receptor-Like Molecules in Lower Eukaryotes
We also obtained sequences without functional assignment but homology to Tom34 in the cryptophyte G. theta, the heterokontophyte P. tricornutum, and the ciliate N. ovalis. This was rather surprising because Tom34 was not identified in fungi, protostomia, or plants. However, TMHMM (Krogh et al. 2001) identified 2 transmembrane helices at the N-terminus of the G. theta EST. The N-terminal transmembrane region might function as a signal sequence. The presence of a second transmembrane helix suggests a relation to the Toc64 family because it was shown that Toc64 contains a transmembrane helix just before the TPR domain (Qbadou et al. 2007), whereas Tom34 is a soluble receptor (Chewawiwat et al. 1999). The TPR domain of the ciliate protein clusters with the C-terminal TPR domain of Tom34 and is thereby of a different type as gtTPR. The N-terminal TPR domain of the P. tricornutum protein pt49004 and the second TPR domain of pt52376 groups with Tom34TPR1 (fig. 4). The first TPR of pt52376 groups with the Hsp70-binding TPR domains of Hop (fig. 4). Therefore, we propose that these proteins are indeed involved in recognition of Hsp90 with the exception of pt52376, which might recognize both chaperones. However, the precise function in protein translocation—whether mitochondrial or chloroplastic—will have to be established in future.
A hypothesis on the History of Hsp-Recognizing TPRs in Protein Translocation
Our analysis suggests a gradual evolution of the TPR-containing import receptors possibly from a preexisting fold (Söding and Lupas 2003). Apparently, they did not evolve altogether and immediately around the time when the endosymbiosis between an α-proteobacterium and the supposably eukaryotic cell took place (fig. 6; eukaryotic cell). Thus, our analysis supports the theoretical considerations of Cavalier-Smith (2006a) that Tom70 has evolved after the genes encoding for small Tim proteins were transferred into the nuclear genome. We suggest that the TPR domain–containing proteins involved in protein translocation evolved several times independently, which is in line with the previous report on mitochondrial TPR–containing receptors (Chan et al. 2006).
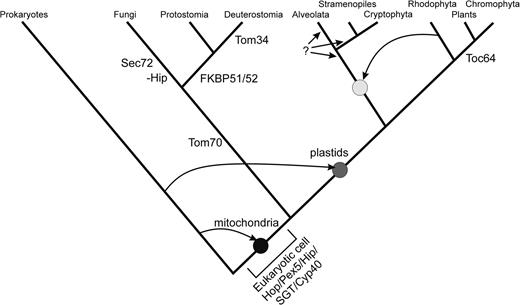
The model of evolutionary development of TPR domain–containing proteins involved in protein translocation. This schematic tree is drawn according to references Stechmann and Cavalier-Smith (2003); McFadden and van Dooren (2004); and Embley and Martin (2006). The black circle indicates the occurrence of the first eukaryotic cell after symbiosis with an α-proteobacterium, the dark gray circle indicates the endosymbiosis with a cyanobacterium, and the light gray circle indicates the secondary endosymbiosis with a red alga. TPR-containing proteins existed already in prokaryotes, which possibly are the origin of the eukaryotic TPRs (see fig. 4). Pex5 and Hop can be found in lower eukaryotes. Tom70 developed on the way to the fungi/metazoa group and Tom34 during development of the bony vertebrates. Sec72 is only found in fungi and is possibly a Hop-like protein (after loss of TPR2a and 2b). Toc64 evolved shortly before or after the split between green algae and plants. The origin of TPR-containing proteins in the upper right group remains elusive (question mark) FKBP51/52 was developed by metazoa. Fungi seem to have lost the cochaperone Hip (−Hip).
First, we found Tom70 homologues with the known domain structure only in the metazoa/fungi group. The sequence with putative homology to Tom70 found in P. tricornutum (pt49004) shows a very similar domain structure but with differences in the putative preprotein binding part. Also, the conserved phenylalanine of Tom70 (position 198 in Tom70 of S. cerevisiae) in its clamp TPR domain is shifted by 2 positions in N-terminal direction. These differences are manifested in pt49004’s phylogenetically closer relation to Tom34TPR1 (fig. 1).
Second, Tom34 sequences were only identified in bony vertebrates (Euteleostomi) (figs. 2 and 6). Here, the linker region between the 2 TPR domains shows a high conservation within the Tom34 family not present in the sequences found in N. ovalis, G. theta, and P. tricornutum (not shown). This and the phylogenetic clustering of the TPR domains of the proteins found in N. ovalis, G. theta, and P. tricornutum (fig. 4) makes it unlikely that these sequences are evolutionary relatives of Tom34 of bony vertebrates.
Hence, we propose that, third, the TPR domain–containing proteins identified in a cryptophyte and a heterokontophyte (sequences with homology to Tom34 in G. theta and P. tricornutum) and in a ciliate (sequence with homology to Tom34, N. ovalis) have evolved independently of the receptor proteins found in plants or metazoa/fungi. This conclusion is supported by the observation that gtTPR matches to Tom34TPR1 and has N-terminally a signal sequence and a transmembrane α-helix not present in Tom34 (TMHMM [Krogh et al. 2001] not shown). In turn, Tom70 contains an N-terminal transmembrane α-helix (Hase et al. 1984) but does not show a significant homology to gtTPR and the spacing between clamp-type TPR and transmembrane α-helix within the sequence is significantly different. Furthermore, the partial Tom34 sequence found in N. ovalis shows a high similarity to Tom34TPR2 and only a very weak similarity to TPR1. The sequences found in P. tricornutum show a domain structure–like Tom70 (pt49004) or Tom34 (pt52376). However, the phylogenetic analysis revealed a closer relationship of the TPR from pt49004 to Tom34TPR1, and the first TPR domain from pt52376 is more alike the Hsp70-binding TPRs of Hop. All together, the relation of the TPR domain–containing proteins of lower eukaryotes to Tom34 might indicate that they evolved independently.
Fourth, Toc64 is the TPR domain–containing import receptor on the plastidic and mitochondrial outer membrane (Chew et al. 2004). Hence, it is tempting to speculate that this receptor evolved after the second endosymbiosis between a mitochondria-containing host and a cyanobacterium. This idea is further supported by the absence of a similar receptor in red algae (McFadden and van Dooren 2004). The dual localization of Toc64 in both mitochondria and chloroplasts (Chew et al. 2004) and the absence of Tom70 in plants might suggest that Tom70 was absent at the time of the second endosymbiosis, even though a loss of this gene cannot be excluded to date.
Fifth, Sec72 represents an Hsp70-recognizing element only, which clearly branches with the HopTPR1 of the same function. Hence, Sec72 might represent a Hop protein after the loss of the other 2 TPR domains. In this context, it is interesting to note that fungi, besides having developed Sec72 during evolution, seem to have lost Hip (fig. 6; −Hip).
Sixth, Pex5 might be the receptor involved in protein import that was evolved first as it can be found in lower eukaryotes as well. However, this protein is not the ancestor of the others (figs. 4 and 6). Some authors even argue that Pex5 might have been derived from the common ancestor of eukaryotes and archaea (Gabaldon et al. 2006).
The emerging picture of evolution of protein translocation suggests that essential components of translocation systems were the seed for the development of the machineries known today and are in case of endosymbiotic organelles inherited from the symbiont (McFadden and van Dooren 2004; Cavalier-Smith 2006a, 2006b; Gabaldon et al. 2006; Löffelhardt et al. 2007 and references therein). This explains the distribution of Pex5, the only essential TPR domain–containing receptor, throughout the entire eukaryotic kingdom (fig. 2, Gabaldon et al. 2006). The TPR domain–containing proteins acting as “regulatory,” “fine tuning,” or “accelerating” components of the translocation machineries without being essential, however, evolved later to enable an efficient distribution of the precursor proteins within the cell explaining the diversity of the components of translocation machineries in organisms of different branches of the tree of life (fig. 6; McFadden and van Dooren 2004; Cavalier-Smith 2006a and references therein). It appears that preexisting domains, folds, or even proteins were “modulated” in their localization, for example, by anchoring to membranes by gene fusion, gene insertions, or deletions (e.g., Lupas et al. 2001). In case of the TPR domain from Toc64, Tom70, Tom34, and Sec72, it is tempting to speculate that chaperone-perceiving domains were “recycled” from chaperone-perceiving folds. For Sec72 clustering with Hop1TPR and the second TPR domain of Tom34 related to Cyp40–TPR (fig. 4), this relation is supported by our findings. For the others, however, future research has to prove this relation.
Supplementary Material
Supplementary figures 1 and 2 and tables 1–3 are available at Molecular Biology and Evolution online (http://www.mbe.oxfordjournals.org/).
We would like to thank Lutz Voigt (Düsseldorf, Germany) for his technical support and Nicole Scherer (Düsseldorf, Germany) for her bioinformatic support. Special thanks to Uwe G. Maier (Marburg, Germany) for providing us with the EST sequence prior to its submission and to Thomas Becker (Freiburg, Germany) and Jason Young (Montreal, Canada) for helpful discussions regarding the project. The work was supported by grants from the Deutsche Forschungsgemeinschaft (SFB-TR01) and from the Volkswagenstiftung to E.S. and Wiener Wissenschafts-, Forschungs- und Technologiefonds to A.v.H.
References
Author notes
T.S. and O.M. equally contributed to this study.
Geoffrey McFadden, Associate Editor