-
PDF
- Split View
-
Views
-
Cite
Cite
Doris Bachtrog, Accumulation of Spock and Worf, Two Novel Non-LTR Retrotransposons, on the Neo-Y Chromosome of Drosophila miranda, Molecular Biology and Evolution, Volume 20, Issue 2, February 2003, Pages 173–181, https://doi.org/10.1093/molbev/msg035
- Share Icon Share
Abstract
Transposable elements constitute a major fraction of eukaryotic genomes. Here, I characterize two novel non-LTR retrotransposons, cloned from the neo-Y chromosome of Drosophila miranda.Worf is 4.1 kb in size and shows homology to the T1-2 non-LTR transposon characterized in Anopheles.Spock is 4.9 kb in size and shows similarity to the Doc element of D. melanogaster. Southern blot analysis of both elements yielded stronger signals for male DNA. In situ hybridization to polytene chromosomes revealed that both elements are accumulating on the neo-Y chromosome of D. miranda. PCR analysis was conducted to investigate the frequency of spock and worf and of the previously identified transposons, TRIM and TRAM, at individual chromosomal sites among 12 strains of D. miranda. Contrary to the observation that element frequencies are usually kept low at individual sites in Drosophila, the four transposons investigated are fixed at their genomic locations on the neo-Y chromosome. These results support the hypothesis that transposons accumulate in nonrecombining regions and may be one cause of the heteromorphism of sex chromosomes.
Introduction
Transposons (TEs) are mobile genetic elements that form a major component of eukaryotic genomes (Finnegan 1989). TEs can be classified into two main groups according to their mechanism of transposition (Berg and Howe 1989; McDonald 1993). DNA transposons mobilize through cut and paste mechanisms that do not involve DNA replication, whereas retrotransposons transpose by reverse transcription of an RNA intermediate (Berg and Howe 1989; McDonald 1993). Retrotransposons are further subdivided into LTR and non-LTR retrotransposons, with the former being bounded by long terminal direct repeats (Finnegan 1989). Transposable elements are likely to be selfish DNA, which persist in the genome due to their ability to undergo replicative transposition (Charlesworth and Langley 1989). Transposition events within the genome can directly generate deleterious effects by inserting into coding or regulatory regions (Charlesworth and Langley 1989; Biemont et al. 1997). Furthermore, ectopic exchange events among copies at nonhomologous genomic locations can lead to chromosomal rearrangements or gene deletions (Langley et al. 1988; Montgomery et al. 1991; Virgin and Bailey 1998). Consistent with their deleterious effects, individual element insertions are normally kept at low frequencies in natural populations of D. melanogaster (Charlesworth and Langley 1989). Under both the insertion and the ectopic exchange models, transposable elements are predicted to accumulate in regions of low recombination (Charlesworth and Langley 1989; Charlesworth, Jarne, and Assimacopoulos 1994; Duret, Marais, and Biemont 2000; Rizzon et al. 2002; Bartolomé, Maside, and Charlesworth 2002).
Heterochromatin is a region of no or suppressed recombination (Baker 1958). TEs have been found to have accumulated on the nonrecombining, heterochromatic Y chromosome of D. melanogaster and D. simulans (Junakovic et al. 1998), as well as in other heterochromatic regions of the D. melanogaster genome (Charlesworth, Jarne, and Assimacopoulos 1994; Pimpinelli et al. 1995). Also, various other groups of eukaryotes contain heterochromatic regions consisting largely of TEs and satellite DNA (Cold Spring Harbor Laboratory 2000; Tilford et al. 2001). Heterochromatin is gene-poor compared with euchromatic regions (Hilliker, Appels, and Schalet 1980; Cold Spring Harbor Laboratory 2000). For example, on the D. melanogaster Y chromosome, which accounts for approximately 13% of a normal male genome (Kennison 1981), fewer than 20 genes have been identified (Carvalho, Lazzaro, and Clark 2000; Carvalho et al. 2001). Thus, TEs could simply accumulate on the Y chromosome or other heterochromatic regions because there are fewer genes, that is, inserted elements are less likely to have deleterious effects on nearby genes. More recently evolved, less degenerated systems are needed to resolve the mechanism behind the observed accumulation of TEs in nonrecombining regions.
Drosophila miranda possess a neo-sex chromosome system, which is formed by the fusion of an autosome (Muller's element C) with the Y chromosome (Macknight 1939). The fused element C, the neo-Y chromosome, is at an intermediate stage in the transition of an ordinary autosome into a degenerate Y chromosome. Some loci on the neo-Y chromosome have been shown to be either malfunctional or completely degenerated (Steinemann and Steinemann 1998), whereas others possess functional alleles on the neo-Y chromosome (Bachtrog and Charlesworth 2002). Nucleotide divergence at silent sites of homologous loci located on the neo-sex chromosomes suggests that the neo-Y chromosome originated about 1MYA (Bachtrog and Charlesworth 2002). Like the true Y chromosome, the neo-Y chromosome shows an increased abundance of heterochromatin and repetitive DNA (Steinemann 1982; Steinemann and Steinemann 1992, 2001). The degeneration of the neo-Y chromosome of D. miranda is thought to be a direct consequence of its lack of recombination, since it is transmitted through males only, and male Drosophila do not undergo meiotic exchange (Gethmann 1988). Thus, D. miranda provides an excellent system for studying the molecular basis of the degeneration of a nonrecombining Y chromosome (Steinemann and Steinemann 1998; Bachtrog and Charlesworth 2002).
Here, I describe the isolation and characterization of two novel non-LTR retrotransposons from the genomic region surrounding the neo-Y–linked exuperantia 1 (exu1) locus in D. miranda, which I call worf and spock. I demonstrate that both elements show an accumulation on the nonrecombining neo-Y chromosome, consistent with theoretical expectations (Charlesworth and Langley 1989; Duret, Marais, and Biemont 2000) and previous observations on the distribution of the TRIM and TRAM retrotransposons (Steinemann and Steinemann 1991, 1997).
Materials and Methods
Drosophila Strains and Genomic Library
The following D. miranda lines were used, with their geographic origin given in parenthesis: 0101.3, 0101.4, 0101.5, and 0101.7 (Port Coquitlam, B.C., Canada), 0101.9, MA28, MA32 (Mather, Calif.), SP138, SP235, SP295 (Spray, Ore.), MSH22, MSH38 (Mt. St. Helena, Calif.). The strains were cultured on banana medium at 18°C.
A genomic library was constructed from D. miranda males, using the Lambda Fix®II Library Kit (Stratagene). High-molecular-weight genomic DNA from D. miranda males was partially digested with Sau3A I and ligated into the lambda vector according to the instructions of the manufacturer.
Detection and Cloning of the TE Sequences
The genomic library of D. miranda was screened for the neo-Y–linked copy of the exuperantia 1 (exu1) gene (Luk et al. 1994). Library screening and standard molecular techniques were carried out according to Sambrook, Fritsch, and Maniatis (1989). To obtain a probe for the library screen, the neo-X-linked copy of the exu1 gene was PCR-amplified from D. miranda, using the primer sequences and PCR conditions described in Yi and Charlesworth (2000a). The obtained PCR product was labeled with the Renaissance Random Primer Fluorescein Labeling Kit and detected with Antifluorescein-AP (NEN) according to the instructions of the manufacturers. Approximately 150,000 phages were screened; the exu1 probe was hybridized at 55°C to replicate filters containing the plaque lifts. Phages from positive plaques were eluted in SM buffer. DNA was prepared from the isolated phage and subcloned into the pZero2.1 vector (Invitrogen). Both DNA strands were sequenced using ABI Prism BigDye chemistry (Perkin-Elmer) on an ABI 377 automated sequencer. Neo-Y linkage of the isolated phage was established by PCR, using various primer combinations that amplify a product only in males.
In Situ Hybridization
Polytene chromosomes of D. miranda were prepared according to the technique of Maside et al. (2001). Probes for the transposable elements were isolated by PCR amplification, using forward and reverse primers internal to each TE. For worf, a 3-kb PCR fragment (forward: 5′-TTCTACCCGAGTCCCAGTTA-3′; reverse: 5′-CAACAGCATCAATCCCATCC-3′) and for spock, a 2.7-kb PCR fragment (forward: 5′-CCGTGAGTAGCGTGGGATTA-3′; reverse: 5′-AGCATAGGAAAGACAAGGAT-3′) were amplified from genomic DNA, followed by agarose gel purification of the PCR products using the QIAquick gel extraction kit (QIAGEN). The DNA fragments were biotin-labeled using Biotin-High-Prime (Roche) and hybridized overnight at 37°C, as described in Maside et al. (2001). Sites of hybridization were detected by staining with diaminobenzidine and peroxidase (Vector Laboratories), and examined under a 40× phase contrast objective. The banding patterns of polytene chromosomes of D. miranda reported by Das et al. (1982) were used as the reference point when determining the sites of hybridization. To test whether the elements are active in the D. miranda genome, three different strains of D. miranda were investigated.
Southern Blot Analysis
Genomic DNA was prepared from 15 to 20 adult flies, using the PUREGENE DNA extraction kit (Gentra). Equal amounts of male and female DNA (5 μg) were digested with the restriction enzymes at 37°C for 5 h and separated on 0.7% agarose gels. The DNA was then denaturated, transferred, and UV–cross-linked to positively charged nylon membranes (Roche). Genomic DNA was digested with two different enzymes for each TE. The first enzyme was chosen to have at least two restriction sites within the TE (worf: Hinf I; spock: Msc I), and the probe used for hybridization only shows homology to an internal restriction fragment. One major band is expected in the Southern blotting experiments, the intensity of which corresponds to the total amount of TE-derived DNA. A second restriction enzyme was chosen for each TE specifically to be informative about the number of distinct insertion sites in the genome. Enzymes were chosen (worf: Pst I; spock: EcoR V) that had at least one cut site within the TE but not within the TE segment used as a probe. The position of a second cut site in the flanking DNA, and therefore the precise size of the band detected by the probe, will be unique to each insertion site in the genome. Thus, this procedure yields an estimate of the number of insertions of each element in the genome. Note, however, that this approach does not allow one to estimate copy number where TEs are arranged in tandem arrays. DNA probes for hybridization were obtained by PCR (worf [1.5-kb fragment]—forward: 5′-TCATTCCAAATTACTGGGAG-3′; reverse: 5′-ATTACCGAACATGCTCCAAA-3′; spock [2.3-kb fragment]—forward: 5′-GACTGCGTCCAAATGAGCGA-3′; reverse: 5′-TTTGCTGAGATAGTCCTGCC-3′), gel purified and fluorescein-labeled using the Renaissance Random Primer Fluorescein Labeling Kit (NEN). The membrane was hybridized to the fluorescein-labeled DNA probe at 65°C overnight according to the manufacturer's instructions. The membranes were washed under the following high-stringency conditions: 15 min each at 65°C, twice with 2× SSC, 1% SDS and twice with 0.2× SSC, 0.1% SDS.
PCR Analysis of Fixation of Elements
To investigate whether elements are fixed at individual sites on the neo-Y chromosome in 12 D. miranda strains, I performed PCR using one primer located inside the TE and the other primer located in the genomic region flanking the element. Thus, the primers should amplify a PCR product only if the TE is present at the specific genomic site in the strain investigated. DNA from single males and females from each line was extracted, using the PUREGENE DNA Extraction Kit (Gentra). I also investigated the transposable elements TRIM and TRAM, which have been identified previously on a neo-Y–linked clone containing the Lcp genes (Steinemann and Steinemann 1991, 1997). The following PCR primers were used, with the forward primers being located in the flanking sequence and the reverse primer in the transposon: spock (forward: 5′-GCTCTCTTATCGGCAACAAA-3′; reverse: 5′-TACCCATCCCCAAGAAGAAT-3′), worf (forward: 5′-CCAGGAGAGGGGCTACAGTG-3′; reverse: 5′-CAGGCTGTTATTGAATCCGT-3′), TRIM (forward: 5′-CTCTCAACAACAGCGAAACA-3′; reverse: 5′-ATGGCAGCAAACCGTCTCCT-3′), TRAM (forward: 5′-CGGTTTCGTTTCCCATCATC-3′; reverse: 5′-TTAGCGGTTCTGCCCCTTCA-3′). PCR reactions were carried out in 25 μl reaction volumes (1.5 mM MgCl2; 0.2 mM of dGTP, dATP, and dTTP and dCTP; 1 μM of each primer; 1U Taq polymerase [Promega]; and 50 to 100 ng of template DNA ). PCR conditions for each locus were as follows: 35 cycles of PCR reactions with denaturation at 94°C for 1 min, annealing at 56°C for 1 min, and extension at 72°C for 2 min. The PCR fragments were separated on a 1% agarose gel.
Results
Sequence analysis of the genomic region surrounding the exu1 gene cloned from the neo-X chromosome and the neo-Y chromosome reveals the presence of two large insertions in the neo-Y–linked copy (fig. 1). Database screens for DNA sequence identities using BLASTN (Altschul et al. 1990) were negative for both insertions. Translated BLASTX searches, in which the nucleotide sequence is translated into all six possible reading frames and searched against the protein database, reveal that both insertions show similarity to non-LTR retrotransposons. The 4,174-bp transposon designated worf shows 34% identity at the amino acid level to the reverse transcriptase domain of the T1-2 element of Anopheles gambiae and 30% identity to the CR1 transposon of the chicken Gallus gallus. The 4,952-bp element called spock reveals 21% identity at the amino acid level to the reverse transcriptase of the Doc transposon of D. melanogaster and 20% to the I-factor of D. teissieri. Sequence comparison between the corresponding DNA stretches from the neo-Y and the neo-X chromosome show strong homology, allowing the exact determination of the boundaries of the TEs (fig. 2). Both elements show no signs of target site duplications (see fig. 2). The sequences of the transposons are deposited in GenBank (accession numbers AY144571 and AY144572).
Characterization of the Worf Transposable Element
The worf transposon has two open reading frames, with the first one having a coding capacity of 386 amino acids and the second having a coding capacity of 965 amino acids (fig. 3). ORF1 shows no similarity to other proteins at the amino acid level, and no conserved domains could be detected when compared with the conserved domain database (CDD) (http://www.ncbi.nlm.nih.gov/Structure/cdd/cdd.shtml). ORF2 shows strong sequence similarity to the reverse transcriptase of several retrotransposons and has a reverse transcriptase domain and an AP endonuclease domain. This copy of worf is integrated into the first intron of the putative gene CG9025 (fig. 1). In situ hybridization to polytene chromosomes in females reveals 24 insertions (fig. 4A). Southern blotting experiments suggest a slightly higher number of copies (∼35 elements) (fig. 5A, lane E). This discrepancy is expected since the latter technique may be better at detecting small, truncated elements and TE insertions in heterochromatic regions.
Characterization of the Spock Transposable Element
The spock transposon encodes two open reading frames, with ORF1 having sequence similarity to a gag-like protein and ORF2 having sequence similarity to a reverse transcriptase (fig. 3). The second ORF contains both a reverse transcriptase domain and an AP endonuclease domain. The cloned neo-Y–linked copy of spock has several stop codons and frame shift mutations, making the exact determination of the coding sequence difficult. This copy of spock is integrated into the intergenic region, between the exu1 gene and the putative gene CG10404 (fig. 1). In females, 16 genomic copies were detected by in situ hybridization (fig. 4C), and the Southern blotting experiment reveals about 25 genomic copies (fig. 5B, lane M).
Accumulation of Worf and Spock on the Neo-Y Chromosome of D. miranda
Southern blots using equal amounts of male and female DNA of D. miranda as well as its sister species D. pseudoobscura were analyzed and are shown in figure 5. Southern blots of Hinf I (worf) or Msc I (spock) digested genomic DNA hybridized with the TE probes should show one major band (as indicated with an arrow at worf lanes A–D and spock lanes I–L in fig. 5). This band's intensity should reflect the total amount of TE-derived DNA. D. miranda shows a stronger hybridization signal than D. pseudoobscura for both TEs, indicating that both worf and spock are more abundant in D. miranda (worf lanes A and B versus C and D and spock lanes I and J versus K and L in fig. 5). For spock, male flies show stronger bands than females for both species (lanes J and L vesus I and K in fig. 5), suggesting that spock is more abundant on the Y chromosome. D. miranda males, however, show the strongest hybridization signals, consistent with an accumulation of both TEs, specifically on the neo-Y. Digestion of D. miranda DNA with Pst I (worf lanes E–H) or EcoR V (spock lanes M–P) allows estimation of the number of genomic copies of those elements because the restriction sites should uncover flanking site heterogeneity, resulting in DNA fragments of different size. The number of elements was found to be higher in males than in females (fig. 5), with worf having approximately 35 elements in females (lane E) and approximately 50 elements in males (lane F) and spock having approximately 25 copies in females (lane M) and approximately 40 copies in males of D. miranda (lane N). Male D. miranda show many more bands than male D. pseudoobscura (worf lane F versus H and spock lane N versus P in fig. 5), suggesting that a large fraction of the elements detected is located specifically on the neo-Y chromosome. Note, however, that these counts are only a rough estimate of the copy number of these two transposable elements in the D. miranda genome.
In situ hybridization to polytene chromosomes was performed to estimate the number of euchromatic copies of spock and worf on different chromosomal arms in D. miranda. The autosomes and the neo-X chromosome contain 24 copies for worf (fig. 4A) and 16 copies for spock (fig. 4C). The neo-Y chromosome has large amounts of heterochromatin, which is underreplicated during polytenization, making it difficult to exactly determine the number of copies. Both elements show intense staining on the neo-Y chromosome (fig. 4B, D), with the total number for worf estimated to be about 30 and the total number for spock estimated to be about to be 25. The detected worf and spock sites on the neo-Y can be directly compared with the number on its homologue, the neo-X. worf shows only six copies on the neo-X, and spock shows only four copies (fig. 4). This direct comparison indicates a neo-Y chromosome–specific accumulation of both elements. Thus, the in situ hybridization data clearly demonstrate that both transposons show an increased abundance on the neo-Y chromosome.
Fixation of TEs on the Neo-Y Chromosome
A PCR approach was employed in order to determine the frequency of the investigated TEs at individual insertion sites among 12 D. miranda strains. A PCR product of the expected size was amplified for all four elements (spock, worf, TRIM, and TRAM) in males of all strains investigated (fig. 6). Thus, all four elements are apparently fixed on the neo-Y chromosome among our 12 D. miranda strains. In females, no product was amplified (fig. 6).
Discussion
I have identified two novel transposons from the genome of D. miranda, which I named worf and spock. Both show homology to non-LTR retrotransposons. BLASTX searches of the TEs reveal that worf clusters with the CRI group, and spock clusters with the jockey group (Malik, Burke, and Eickbush 1999). I show that both worf and spock are accumulating on the nonrecombining neo-Y chromosome of D. miranda, consistent with both theoretical expectations (Charlesworth and Langley 1989; Duret, Marais, and Biemont 2000) and previous observations. The Lcp gene family isolated from the neo-Y chromosome of D. miranda contains the insertion of two retrotransposons, called TRIM and TRAM (Steinemann and Steinemann 1991, 1997). Both TRIM and TRAM were also shown to be preferentially located on the neo-Y chromosome (Steinemann and Steinemann 1991, 1997).
Transposable element frequencies are low at individual chromosomal insertion sites for D. melanogaster (Charlesworth and Langley 1989); in situ hybridization data show that, most of the time, a site is occupied only once by members of a given TE family in a sample size of 10 to 20 (Charlesworth and Langley 1989). The skewed frequency distribution of Drosophila TEs towards rare insertions is evidence for purifying selection acting to remove TEs from natural populations (Charlesworth and Langley 1989; Charlesworth, Lapid, and Canada 1992) and has been used to estimate the strength of selection against TE insertions (Charlesworth and Langley 1989). In agreement with the D. melanogaster data, the autosomal, neo-X, and X-linked copies of both worf and spock were found to be segregating among the different strains investigated (data not shown). The TRIM (Steinemann and Steinemann 1991) and the TRAM (Steinemann and Steinemann 1997) elements were also reported to segregate at their euchromatic locations among strains. In sharp contrast, all four transposon insertions investigated are fixed at their specific chromosomal sites on the neo-Y chromosome near the exu1 and the Lcp genes in D. miranda. Thus, both the accumulation and the fixation of TEs in D. miranda seem to be a unique feature of the neo-Y chromosome. Transposons might be accumulating on the neo-Y chromosome either because their deleterious effect is reduced (i.e., fewer functional genes, insertion into genes that are dosage compensated on the neo-X, or absence of ectopic exchange on the nonrecombining neo-Y) or because of the reduced efficacy of natural selection on a nonrecombining chromosome.
Reduced Gene Expression on the Neo-Y Chromosome?
The neo-Y chromosome of D. miranda is in an intermediate stage in the transition of an ordinary, mostly euchromatic autosome into a degenerated, heterochromatic Y chromosome. α-Heterochromatin undergoes little if any replication during polytenization (Gall, Cohen, and Polan 1971). In contrast to the completely heterochromatic Y chromosome, the neo-Y of D. miranda is still partly polytenized in salivary gland cells (fig. 4). This indicates the presence of euchromatic, genetically active regions on the neo-Y. Some neo-Y chromosome genes have been shown to have degenerated (e.g., the Lcps and Amy [Steinemann, Steinemann, and Lottspeich 1993; Steinemann and Steinemann 1999]), whereas others show functional copies on the neo-Y (e.g., eve, robo, and CycB [Bachtrog and Charlesworth 2002]). Given that there are still many functional genes on the neo-Y chromosome, the accumulation of TEs is unlikely to result solely from a low density of expressed genes. The four TE insertions found so far on the neo-Y chromosome of D. miranda lie outside coding regions, further suggesting that transposons do not simply accumulate on the neo-Y due to the lack of active genes. However, the Lcp4 gene on the neo-Y chromosome contains a large deletion in the coding region flanked by a DNA sequence duplication, probably representing the footprint left behind by an insertion/excision event of a transposable element (Steinemann and Steinemann 1992).
Parts of the neo-X chromosome have been found to be dosage compensated (Bone and Kuroda 1996; Marin et al. 1996; Steinemann, Steinemann, and Turner 1996); thus, the insertion of a TE in or close to a gene on the neo-Y that is dosage compensated on the neo-X is not expected to have any deleterious effects. One could even speculate that some transposable elements on the neo-Y chromosome are fixed by positive selection (Rice 1996). If a gene is dosage compensated on the neo-X, and if the neo-Y–linked copy encodes a malfunctional product, it might be advantageous to knock out the neo-Y–linked allele to silence a malfunctional copy of the gene, which might interfere with the functional copy on the neo-X. This could be achieved by the insertion of a transposable element into the regulatory or coding region of a gene.
Reduced Deleterious Effects of TEs Due to the Lack of Ectopic Exchange?
Transposable elements are expected to accumulate in regions of suppressed recombination under the hypothesis that TEs are selected against due to the deleterious effects of chromosomal rearrangements caused by nonhomologous recombination among copies (Charlesworth and Langley 1989; Virgin and Bailey 1998). Under this model, the TE insertion itself has no associated fitness costs but is selected against because of its indirect deleterious effect caused by ectopic recombination (Montgomery et al. 1991). The neo-Y chromosome completely lacks recombination; thus, selection against elements resulting from ectopic exchange is absent and TEs can accumulate on the neo-Y chromosome in a neutral manner.
Reduced Selection Against Transposons Due to Hill-Robertson Interference?
Selection at linked sites reduces the efficacy of natural selection, a phenomenon known as the Hill-Robertson effect (Hill and Robertson 1966). On a nonrecombining chromosome, where all sites are completely linked, the magnitude of this effect can be enormous (Charlesworth and Charlesworth 2000). Consistent with the predictions of Hill-Robertson interference, we have previously shown that the effective population size of the neo-Y chromosome is greatly reduced (Bachtrog and Charlesworth 2000, 2002) and that the efficacy of natural selection is reduced on the neo-Y, as seen by a higher rate of deleterious amino acid replacements and a lower rate of adaptive evolution on the neo-Y (Bachtrog and Charlesworth 2002). A very crude estimation of the strength of selection against TEs on the neo-Y chromosome can be performed as follows. Data from the frequency distribution of TEs on individual sites in the genome from D. melanogaster suggest that the product of 4Nes for transposons is around 10 to 100 (assuming that selection is the only force of removal of elements [Charlesworth, Lapid, and Canada 1992]). Assuming the same selection coefficient s against TEs for D. miranda and an approximately threefold lower species-wide population size Ne (Yi, Bachtrog, and Charlesworth, unpublished data), 4Nes in D. miranda is expected to be around 3 to 30. The neo-Y chromosome of D. miranda shows a strong reduction in the effective population size compared with other chromosomal regions; the reduction in Ne of the neo-Y chromosome compared with the neo-X is estimated to be around 30-fold (Bachtrog and Charlesworth 2000, 2002). Thus, although this estimation is very crude and depends on several assumptions, 4Nes for transposons on the neo-Y chromosome might well be around or below unity. If this is indeed the case, slightly deleterious TEs might accumulate on the neo-Y due to random genetic drift (Kimura 1983).
Various forms of Hill-Robertson effects are compatible with the reduction in Ne and can produce the observed accumulation of TEs on the neo-Y chromosome of D. miranda (for a review see Charlesworth and Charlesworth 2000): (1) Purifying selection against strongly deleterious alleles (background selection) can strongly reduce the effective population size of the neo-Y chromosome and thus increase the fixation probability of slightly deleterious mutations such as transposons (Charlesworth 1994). (2) Muller's ratchet (Muller 1964), the random loss of the class of chromosomes containing the fewest number of TE insertions, can cause the accumulation and fixation of TEs on the neo-Y chromosome (Gordo and Charlesworth 2001). (3) The fixation of a strongly advantageous mutation (selective sweep) arising on the nonrecombining neo-Y chromosome can drag along to fixation all deleterious TEs it is initially associated with (Rice 1987).
Transposable Elements and Y Chromosome Degeneration
Both the accumulation of transposable elements (see above and Steinemann and Steinemann 1991, 1997) and amino acid substitutions (Bachtrog and Charlesworth 2002; Yi and Charlesworth 2000b) can lead to the observed inactivation of Y-linked genes and, in the long term, to the degeneration of the Y chromosome. In various Drosophila species, few genes required for male fertility remain on the Y chromosome (Carvalho, Lazzaro, and Clark 2000; Kurek et al. 2000; Carvalho et al. 2001). One peculiarity of these Y-linked genes is the presence of mega-base–sized introns, consisting largely of TE-derived and satellite DNA (Kurek et al. 2000). Interestingly, the worf transposon is fixed in the first intron of the CG9025 gene, possibly reflecting the initial stages in the evolution of the mega-base–sized introns on the Y chromosome.
Steinemann and Steinemann (1998) proposed a model in which they suggest that the first step in Y chromosome degeneration is driven by the accumulation of transposable elements. The enriched occurrence of these elements along a degenerating Y chromosome could account for the switch from euchromatic to heterochromatic chromatin structure (Steinemann and Steinemann 1998). There is evidence that TEs cause the formation of heterochromatic DNA; tandem arrays of engineered P elements give rise to de novo formation of heterochromatic structures (Dorer and Henikoff 1994). The accumulation of transposons, together with the accumulation of satellite DNA (Charlesworth, Sniegowski, and Stephan 1994), can cause heterochromatinization of the chromosome, leading to the silencing of genes on the neo-Y chromosome. Our results on the distribution of worf and spock corroborate the importance of TEs in the evolution of Y chromosomes.
E-mail: [email protected].
Thomas Eickbush, Associate Editor
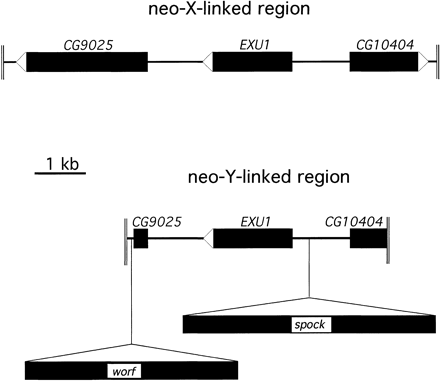
Schematic map of the genomic region surrounding the exu1 gene on the neo-Y and the neo-X chromosome of D. miranda. The neo-Y–linked region contains the insertion of two transposable elements called worf and spock.
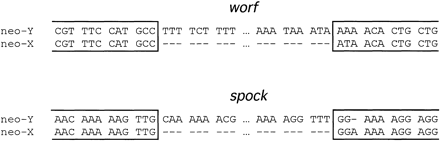
Alignment of the integration sites of the worf and spock transposons on the neo-Y chromosome. No target site duplications were detected
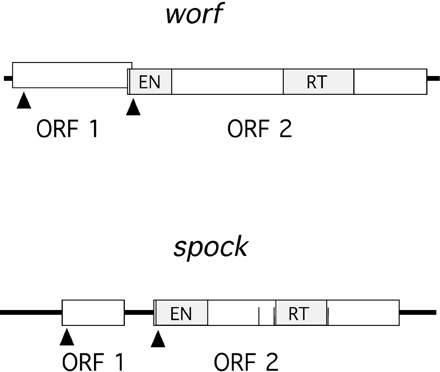
Scheme of worf and spock sequences. Open boxes represent ORFs. Arrows indicate the position of possible initiation methionines. Vertical filled boxes represent conserved protein domains. The three vertical lines in spock indicate stop codons
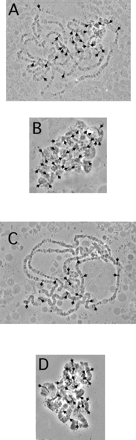
In situ hybridization of D. miranda for worf and spock. Arrows point to hybridization signals. The star indicates the neo-X chromosome. (A) Female nucleus of D. miranda (strain 0101.4). Hybridization of worf; 24 insertions were detected. (B) Neo-Y chromosome hybridized with worf; about 30 insertions were observed. (C) D. miranda female nucleus (strain MSH38). Hybridization of spock; 16 insertions were detected. (D) Neo-Y chromosome hybridized with spock; about 25 insertions were observed
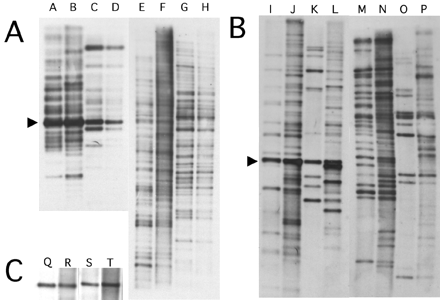
Southern blot hybridization of worf (A) and spock (B), using D. miranda strain MSH22. (A) Hybridization to the worf probe (lanes A–H). Lane A: D. miranda female DNA, HinfI; lane B: D. miranda male DNA, HinfI; lane C: D. pseudoobscura female DNA, HinfI; lane D: D. pseudoobscura male DNA, HinfI; lane E: D. miranda female DNA, PstI; lane F: D. miranda male DNA, PstI; lane G: D. pseudoobscura female DNA, PstI; lane H: D. pseudoobscura male DNA, PstI. (B) Hybridization to the spock probe (lanes I–P). Lane I: D. miranda female DNA, MscI; lane J: D. miranda male DNA, MscI; lane K: D. pseudoobscura female DNA, MscI; lane L: D. pseudoobscura male DNA, MscI; lane M: D. miranda female DNA, EcoRV; lane N: D. miranda male DNA, EcoRV; lane O: D. pseudoobscura female DNA, EcoRV; lane P: D. pseudoobscura male DNA, EcoRV. (C) Hybridization with the pic gene from D. melanogaster (as an external control for monitoring equal amounts of genomic DNA, lanes Q–T). Lane Q: D. miranda female DNA, EcoRI; lane R: D. miranda male DNA, EcoRI; lane S: D. pseudoobscura female DNA, EcoRI; lane T: D. pseudoobscura male DNA, EcoRI
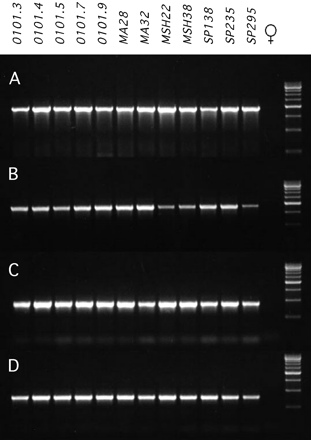
Fixation of the transposable elements on the neo-Y chromosome. Lanes 1 to 12 show the PCR product of the 12 different D. miranda strains investigated, using male genomic DNA. Lane 13 shows one representative female (note: no female amplified a PCR product) and lane 14 shows a size standard (500-bp ladder). (A) worf, 1.9-kb PCR product. (B) spock, 1.9-kb product. (C) TRIM, 890-bp product. (D) TRAM, 780 bp-product
I thank P. Andolfatto, B. Charlesworth, I. Gordo, and S. Wright for helpful discussions and comments on the manuscript. D.B. was supported by a European Molecular Biology Organization fellowship.
Literature Cited
Altschul, S. F., W. Gish, W. Miller, E. W. Myers, and D. J. Lipman.
Bachtrog, D., and B. Charlesworth.
Bachtrog, D., and B. Charlesworth.
Bartolomé, C., X. Maside, and B. Charlesworth.
Biemont, C., A. Tsitrone, C. Vieira, and C. Hoogland.
Bone, J. R., and M. I. Kuroda.
Carvalho, A. B., B. A. Dobo, M. D. Vibranovski, and A. G. Clark.
Carvalho, A. B., B. P. Lazzaro, and A. G. Clark.
Charlesworth, B.
Charlesworth, B., and D. Charlesworth.
Charlesworth, B., P. Jarne, and S. Assimacopoulos.
Charlesworth, B., and C. H. Langley.
Charlesworth, B., A. Lapid, and D. Canada.
Charlesworth, B., P. Sniegowski, and W. Stephan.
Cold Spring Harbor Laboratory.
Das, M., D. Mutsuddi, A. K. Duttagupta, and A. S. Mukherjee.
Dorer, D. R., and S. Henikoff.
Duret, L., G. Marais, and C. Biemont.
Finnegan, D. J.
Gall, G. J., E. H. Cohen, and M. L. Polan.
Gordo, I., and B. Charlesworth.
Hill, W. G., and A. Robertson.
Hilliker, A. J., R. Appels, and A. Schalet.
Junakovic, N., A. Terrinoni, C. Di Franco, C. Vieira, and C. Loevenbruck.
Kennison, J. A.
Kurek, R., A. M. Reugels, U. Lammermann, and H. Bünemann.
Langley, C. H., E. Montgomery, R. Hudson, N. Kaplan, and B. Charlesworth.
Luk, S. K., M. Kilpatrick, K. Kerr, and P. M. Macdonald.
Malik, H. S., W. D Burke, and T. H. Eickbush.
Marin, I., A. Franke, G. J. Bashaw, and B. S. Baker.
Maside, X., C. Bartolome, S. Assimacopoulos, and B. Charlesworth.
Montgomery, E. A., S. M. Huang, C. H. Langley, and B. H. Judd.
Pimpinelli, S., M. Berloco, L. Fanti, P. Dimitri, S. Bonaccorsi, E. Marchetti, R. Caizzi, C. Caggese, and M. Gatti.
Rice, W. R.
Rizzon, C., G. Marais, M. Gouy, and C. Biemont.
Sambrook, J., E. F. Fritsch, and T. Maniatis.
Steinemann, M.
Steinemann, M., and S. Steinemann.
Steinemann, M., and S. Steinemann.
Steinemann, M., and S. Steinemann.
Steinemann, M., and S. Steinemann.
Steinemann, M., S. Steinemann, and F. Lottspeich.
Steinemann, M., S. Steinemann, and B. M. Turner.
Steinemann, S., and M. Steinemann.
Steinemann, S., and M. Steinemann.
Tilford, C. A., T. Kuroda-Kawaguchi, H. Skaletsky, et al. (12 co-authors).
Virgin, J. B., and J. P. Bailey.
Yi, S., and B. Charlesworth.