-
PDF
- Split View
-
Views
-
Cite
Cite
Nicholas I. Mundy, Shelley Cook, Positive Selection During the Diversification of Class I Vomeronasal Receptor-like (V1RL) Genes, Putative Pheromone Receptor Genes, in Human and Primate Evolution, Molecular Biology and Evolution, Volume 20, Issue 11, November 2003, Pages 1805–1810, https://doi.org/10.1093/molbev/msg192
- Share Icon Share
Abstract
Vomeronasal receptors are the major receptors for pheromones in vertebrates, and five putative type 1 vomeronasal receptors (V1RL) have been identified in humans. The evolution of the V1RL1 gene in non-human primates, and patterns of selection on V1RL genes, were investigated. The presumed ortholog of V1RL1 was sequenced from 13 species of nonhuman primate, and in eight of these species V1RL1 was a pseudogene. Phylogenetic reconstructions reveal that V1RL1 pseudogene formation occurred independently in multiple primate lineages. Using maximum likelihood estimates of dN/dS ratios in PAML, we show that V1RL genes have evolved under neutral evolution in lineages in which they became a pseudogene. In contrast, among lineages in which V1RL genes contain an open reading frame, the majority of sites are under purifying selection and a minority are under significant positive selection. These results provide an interesting case where all three categories of selection can be teased apart in the same data set using maximum likelihood methods. The finding of positive selection on V1RL genes during primate evolution provides indirect support for the hypothesis that V1RL genes have a function in species-specific pheromone detection in primates.
Introduction
The vomeronasal system is a phylogenetically ancient chemosensory system of vertebrates that is anatomically and functionally distinct from the main olfactory system (reviewed in Keverne 1999). This system has a major role in the detection of pheromones, which are odorant molecules used in species-specific communication. Primary detection of odorants in the vomeronasal system occurs in the vomeronasal organ (VNO). At the molecular level, detection in the VNO is via vomeronasal receptors, which are part of the large superfamily of 7-transmembrane G protein–coupled receptors (Dulac and Axel 1995; reviewed in Dulac 1999). There are two classes of vomeronasal receptor, V1Rs and V2Rs (Type 1 and type 2 vomeronasal receptors), that have distinctive structures, G protein–coupling systems, and expression patterns in the VNO (Keverne 1999). Pheromone detection is important in most mammalian groups including rodents, and in mice there is a well-developed VNO and a high diversity of vomeronasal receptors, with at least 137 V1Rs and around 100 V2Rs expressed from separate genomic loci (Rodriguez et al. 2002).
During the evolution of higher primates the VNO decreased in size (reviewed in Martin 1990), and this has been interpreted as being due to a reduction in the importance of pheromone communication in these groups. In New World monkeys the VNO is small, whereas in Catarrhine primates (humans, apes, and Old World monkeys) it is vestigial, and probably nonfunctional. In spite of the apparent absence of a functional vomeronasal system in humans, interest in the role of pheromones in human biology is growing because of the increasing evidence for pheromonal effects in human mate choice (Wedekind et al. 1995; Jacob et al. 2002).
In humans, V1R diversity is low, and only five potentially functional vomeronasal receptor genes (V1R-like or V1RL genes) have been identified from human genome searches (V1RL1 to 5; Giorgi et al. 2000; Rodriguez et al. 2000; Kourso-Mehr et al. 2001; Rodriguez and Mombaerts 2002). The best characterized of these genes is V1RL1. Interestingly, V1RL1 mRNA is expressed not in the vestigial human VNO but in the main olfactory epithelium (Rodriguez et al. 2000). However, it is not known if a functional V1R1 receptor is produced.
There has been an acceleration in the number of examples of positive selection detected in diverse systems, which has been stimulated by the development of more powerful methods for its analysis (reviewed in Bielawski and Yang, 2002). As pheromones are species-specific and have important functions in social and reproductive behavior, pheromone receptors may be evolving rapidly, and we hypothesized that if V1RL genes code for functional pheromone receptors then they may be under positive Darwinian selection during primate evolution. To test this hypothesis we have sequenced the V1RL1 gene from anthropoid primates (monkeys and apes) and analyzed these data together with published human V1RL1 and recently described human V1RL2-5 gene sequences and marmoset V1R pseudogene sequences. The results provide evidence for the action of both positive and purifying selection on different sites in human and primate V1RLs. In addition, reconstruction of pseudogene formation of V1RL1 genes strongly suggests that it occurred independently in several lineages of great apes and New World monkeys.
Materials and Methods
Laboratory Work
Genomic DNA samples had previously been extracted from tissue samples of Anthropoid primates using Qiagen kits. The following samples were used (lab ID is given in parentheses): Apes: common chimpanzee, Pan troglodytes (Ptr4); western lowland gorilla: Gorilla gorilla gorilla (Ggo6); orangutan: Pongo pygmaeus (Ppy2); lar gibbon, Hylobates lar, (Hla1). Old World monkeys: olive baboon, Papio anubis (Pan1); ebony langur, Trachypithecus auratus (Tau1); De Brazza's monkey, Cercopithecus neglectus (Cne1) New World monkeys: common marmoset: Callithrix jacchus (Cja13); pygmy marmoset: Cebuella pygmaea (Cpy690); Goeldi's monkey: Callimico goeldii (Cgo20); night monkey: Aotus sp (Aot1); golden-headed lion tamarin: Leontopithecus chrysomelas (Lch2); saddle-backed tamarin: Saguinus fuscicollis (Sfu1); white-fronted capuchin: Cebus albifrons (Cal1); mantled howler: Alouatta palliata mexicana (Apm2); Bolivian red howler: Alouatta sara (Asa3); red howler: Alouatta seniculus (Ase2).
V1R genes contain a single coding exon of about 1 kb. External primer pairs designed to amplify a 735–744-bp portion of this exon of the V1RL1 gene were VOMR1 (5′-GGAGTTGGGATCCTGGGAAA-3′) and VOMR2 (5′-CTCATGATGAGGACAAAAGGGCT-3′) (annealing temperature of 52°−65°C), or VOMR5 (5′-TGGGAAATTCCTTTCTCCTTTG-3′) and VOMR6 (5′-GGGCTGAGTGCTGGRAAAC-3′) (annealing temperature of 55°−60°C). Internal sequencing primers were VOMR3 (5′-AATCGCTTGGAATCCATTGAG-3′) and VOMR4 (5′-TTTATGAGTTTGGGCTTCATGG-3′).
Polymerase chain reactions (PCR) were prepared in a 25 μl total volume containing: 0.1 μl Taq polymerase (Thermoprime Plus DNA polymerase 5U/μl, ABGene, Surrey, U.K.). 2.5 μl 10× reaction buffer, 1.5 μl 25 mM magnesium chloride, 0.05 μl of each dNTP (25 mM), 1 μl of each primer (10 μM), and 25–100 ng DNA. All PCRs were performed in a Techne Genius Cycler (Hybaid), with the following cycling parameters: 94°C for 2 min, 35×: (94°C for 30 s, annealing temperature for 45 s, 72°C for 90 s), 72°C for 5 min. The PCR products were directly sequenced on both strands by cycle sequencing using Big Dye Version 2 (PE Biosystems) under standard conditions, and run on an ABI 377 sequencer. Sequences were edited in Sequencher. The sequences have been deposited in GenBank (accession numbers AY314011–AY314023).
Data Analysis
Sequences were aligned with ClustalX. A 765-bp alignment of all primate V1R sequences was used in PAUP*, and a 744-bp alignment of V1RL1-4 sequences was used for PAML analyses (this alignment was shorter because it was based on more closely related sequences). To infer evolutionary relationships among primate V1R genes, phylogenetic reconstructions were performed using the Neighbor-Joining method with HKY85 distances and maximum likelihood with the HKY85 model of substitution in PAUP* Version 4.0b10 (Swofford 1999). Branch support using Neighbor-Joining was estimated with 1,000 bootstrap replicates.
Estimation of dN/dS ratios was carried out by maximum likelihood using a codon-based substitution model in PAML Version 3.13 (Yang 1997). Two series of analyses were performed: (1) lineage-specific models in which all codon sites are assumed to be under the same selective pressure in a particular lineage but the selective pressure can vary among different lineages, and (2) site-specific models in which selective pressure varies among different sites but the site-specific pattern is identical across all lineages. Several different site-specific models were implemented: model M0 (null model with a single dN/dS ratio among all sites), M1 (“neutral” model, with two categories of site with fixed dN/dS ratios of 0 and 1), M2 (“selection” model: three categories of site, two with fixed dN/dS ratios of 0 and 1, and a third estimated dN/dS ratio), M3 (“discrete” model: three categories of site, with the dN/dS ratio free to vary for each site), M7 (“Beta model”: eight categories of site, with eight dN/dS ratios in the range 0–1 taken from a discrete approximation of the beta distribution), and M8 (“Beta plus omega” model: eight categories of site from a beta distribution as in model M7 plus an additional category of site with a dN/dS ratio that is free to vary from 0 to greater than 1). PAML estimates the dN/dS ratios that are free to vary under these models, as well as the proportion of sites with each ratio. Likelihood ratio tests, to determine whether particular models provided a significantly better fit to the data than other nested models, were performed by comparing the likelihood ratio test statistic (−2[LogLikelihood1 − LogLikelihood2]) to critical values of the Chi square distribution with the appropriate degrees of freedom (Yang 1998). P values for sites potentially under positive selection were obtained using a Bayesian approach in PAML.
Results and Discussion
V1RL1 Genes in Non-human Primates
V1RL1 sequences were obtained for 13 species of non-human primates including great apes and several New World monkeys such as marmosets, tamarins, and howler monkeys (see fig. 1b for a full list of species). The region sequenced comprises about 80% of the predicted V1R protein, extending from the first transmembrane domain to the seventh transmembrane domain (fig 2). No PCR products within 300 bp of the expected size were amplified from any of the Old World monkey and gibbon species with three pairs of non-overlapping conserved forward and reverse primers, or combinations of these primers. As 1-kb amplicons from other loci (e.g., melanocortin-1 receptor gene) were easily obtained from these samples, we consider it most likely that V1RL1 is absent or highly modified in these species.
The V1RL1 sequences were aligned with all other available primate V1R sequences. Phylogenetic reconstructions using Neighbor-Joining and maximum likelihood methods produced similar topologies in which V1RL1 sequences from humans and all other primates formed a well-supported monophyletic clade, which was the sister group to a clade comprising human V1RL2, V1RL3, V1RL4 genes and the marmoset V1R pseudogene MsM11 (fig 1a). The V1RL1 gene phylogeny is generally consistent with the accepted primate species phylogeny. Together, these results strongly suggest that the V1R sequences obtained from non-human primates are orthologous to human V1RL1. Previous work has shown that human V1RL1-4 are distantly related to the 12 mouse V1R subfamilies and that a clade comprising these human V1RL1-4 is monophyletic with respect to the 137 mouse V1Rs (Rodriguez and Mombaerts 2002). We confirmed that the new primate V1RL1 sequences had no close matches among other V1R genes in GenBank. The clade containing all primate V1RL1 to V1RL4 genes has strong bootstrap support in our analysis, and we used this clade to investigate patterns of selection described below.
The primate V1RL1 sequences contained an open reading frame (ORF) in the region sequenced in only six of the 14 species: human, gorilla, pygmy marmoset, and three species of howler monkey (fig. 1b). In the remaining eight species sequenced, all from different genera, the V1RL1 sequence was clearly a pseudogene, resulting from point substitutions creating stop codons (common marmoset), from a large deletion of 242 bp (saddle-backed tamarin), or from one or two small frameshifting deletions of 1–7 bp (chimpanzee, orangutan, night monkey, capuchin, lion tamarin, Goeldi's monkey). During the course of the study, full-length V1RL1 sequences were independently isolated from chimpanzee, gorilla, and orangutan (Giorgi and Rouquier 2002). These sequences differ from our V1RL1 sequences for these species at 2, 0, and 2 nucleotide sites, respectively, and they confirm the presence of a V1RL1 ORF in gorilla and pseudogenes in chimpanzee and orangutan. Reconstruction of V1RL1 pseudogene formation over the independently established primate phylogeny (fig. 1b) suggests that it occurred independently several times during anthropoid evolution, in terminal lineages.
Patterns of Selection in Primate V1R Gene Evolution
The relative rates of synonymous (dS) and nonsynonymous (dN) substitutions indicate the nature of the selective forces that have shaped the evolution of a coding sequence. Most codons are under purifying selection most of the time (dN/dS less than 1), because the majority of nonsynonymous mutations are deleterious and are removed by selection. Under neutral evolution, for example in pseudogenes, the expected dN/dS is one. Of particular interest are cases where dN/dS is greater than one (i.e., dN is greater than dS), because the rapid fixation of nonsynonymous substitutions must be due to the action of positive selection at the codons involved, and this therefore provides unequivocal evidence for adaptive evolution. Estimates of dN and dS during primate V1RL1-4 evolution were obtained by maximum likelihood using a codon-based substitution model in PAML (Yang 1997; tables 1 and 2). Human V1RL5 was excluded from this analysis as it is highly divergent and its ancestry with human V1RL1-4 predates the split between primates and rodents (Rodriguez and Mombaerts 2002).
First, we were interested in whether it was possible to detect changes in selection pressure on V1RL genes before and after pseudogene formation. All of the sequences in figure 1b were used for this analysis. All codon sites were treated equivalently, and lineages in which V1RL1 became a pseudogene (“pseudogene lineages”—see fig 1b) were considered as a separate category from lineages in which V1RL1s and V1RL2-4 were reconstructed as potentially functional (“ORF lineages”; table 1). The overall estimated dN/dS ratio (known as ω in PAML) among pseudogene lineages (1.02) was close to 1, as expected for neutral evolution. In contrast, the estimated dN/dS ratio over all V1RL “ORF” lineages was 0.64. This value was significantly less than 1 in a likelihood ratio test (test I in table 3), providing evidence for purifying selection among primate V1RLs when all sites are treated equally. This lends support to the reconstruction of pseudogene evolution shown in figure 1b, and similar significant results were obtained using PAML analyses on V1RL1 sequences alone (not shown). This provides an example of the use of PAML to detect differences in selection pressure on functional genes and pseudogenes.
The finding of purifying selection among V1R “ORF” lineages when dN/dS ratios are averaged over all sites is not surprising, because even if certain sites are under positive selection this would be masked if purifying selection is occurring at the majority of sites. To investigate the nature of selection in the V1RL ORF lineages further, we carried out a separate series of analyses on the ORF lineages alone (see fig. 1b) in which the type of selection varies among different categories of codon site (Yang et al. 2000). Several different site-specific models of increasing complexity were implemented (see Materials and Methods for details). Three of these models (M2, M3, and M8) permit a proportion of sites to have a dN/dS ratio greater than 1, (i.e., they accommodate the possibility of positive selection). When applied to the V1R data, these three models all led to a category of sites in the V1R data with an estimated dN/dS ratio greater than 1 (2.22–4.75; table 1), suggesting that a proportion of codons (estimated to be 0.05–0.16; table 1) are under positive selection during V1R evolution in primates. Statistical support for this was obtained in likelihood ratio tests with nested simpler models (tests II to IV in table 2). Thus positive selection is present in primate V1RL gene evolution from the duplication events that gave rise to V1RL1 to V1RL4 through to diversification of V1RL1 in anthropoid primates. Analyses performed separately on V1RL1 sequences alone also gave a proportion of sites under positive selection, but in this case likelihood ratio tests for positive selection were not significant at the 5% level (not shown).
Given that there is a category of sites under positive selection, Bayesian inference can be used in PAML to identify specific codons that have a significant chance of being under positive selection. Results from all three models allowing positive selection (M2, M3, and M8) were compared to reduce the chance of false positives (Anisimova, Bielawski, and Yang 2001; Suzuki and Nei 2002). A single codon position (172, numbering from human V1RL1) has a greater than 95% probability of being under positive selection under all three models (fig 2). This residue is highly variable among the sequences studied (human and gorilla V1RL1s: serine; New World monkey V1RL1s: proline; human V1RL2: proline; human V1RL3: asparagine; human V1RL4: arginine). It is in the long third extracellular domain of the receptor, where it could potentially interact with odorant ligands, although it should be emphasized that the structure-function relationships of V1Rs in general are poorly understood.
The presence of positive selection on V1RL genes during human and non-human primate evolution is consistent with these genes having a role in species-specific pheromone detection in primates. The finding of V1RL1 polymorphism in humans (Rodriguez et al. 2000) suggests that diversification of V1RL1 function could be occurring within human populations, and it is intriguing that one of the two variable amino acid positions in humans (site 229—alanine or glutamate in humans) has also undergone nonsynonymous substitution in gorillas (threonine).
The V1RL genes join the growing number of genes that have been shown to be under positive selection in one or more primate lineages, as inferred by dN/dS ratios greater than 1 (e.g., MHC class I: Hughes and Nei 1988; lysozyme: Messier and Stewart 1997; SRY: Pamilo and O'Neill 1997; ribonuclease: Zhang, Rosenberg, and Nei 1998; alanine glyoxylate aminotransferase (AGT): Holbrook et al. 2000; BRCA1: Huttley et al. 2000; Protamine-1, Protamine-2, Tnp-2: Wyckoff, Wang, and Wu 2000; morpheus: Johnson et al. 2001; glycophorin A: Baum, Ward, and Conway 2002). The majority of these genes have roles in the immune system (MHC genes), male reproduction (SRY, protamine, Tnp-2), or digestion/metabolism (lysozyme, ribonuclease, AGT). V1RLs are one of the first examples of genes with putative functions in the sensory system that have been under directional selection during primate evolution. Another example are the opsin genes involved in color vision, which underwent positive selection following duplication in Catarrhine primates, and are under balancing selection in most New World monkey lineages (Shyue et al. 1995; Surridge and Mundy 2002).
V1R Gene Family Evolution in Primates
Overall, data on V1R gene evolution in primates are still rather scarce, but some important conclusions are beginning to emerge. Pseudogene formation is not confined to the human lineage, but has occurred independently in different lineages and, in marmosets at least, in several genes (Giorgi and Rouquier 2002). This is reminiscent of the pattern of evolution in the large olfactory receptor gene family in non-human primates (Rouquier, Blancher, Giorgi 2000; Whinnett and Mundy 2003). The pattern of V1RL1 pseudogene formation among hominoids is particularly notable—this is the only V1R gene known to be expressed in humans, but it is a pseudogene in our closest relative, the chimpanzee, an ORF in gorillas, and a pseudogene in orangutans. It is seems likely that the repertoire of functional V1R genes is highly lineage specific in primates.
The V1Rs and the VNO are extremely important in mice, and in this lineage there has been a rapid evolutionary turnover of V1R genes, involving both duplication and pseudogene formation (Lane et al. 2002; Rodriguez and Mombaerts 2002). It remains to be seen whether lineages of non-human primates with a functional VNO, such as marmosets, have had V1R diversification in addition to the pseudogene formation documented here.
Brian Golding, Associate Editor
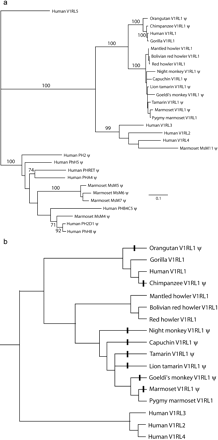
Phylogenetic analysis of V1RL sequences and reconstruction of V1RL1 pseudogene evolution in primates. Pseudogene sequences are labeled “ψ.” a. Maximum likelihood tree of all primate V1R sequences. Numbers on branches represent percentage bootstrap support using Neighbor-Joining. b. Reconstruction of V1RL1 pseudogene evolution over the known primate phylogeny obtained from independent data sets. These sequences and phylogeny were used in the first part of the PAML analyses. Bars across lineages indicate pseudogene formation. Lineages with a bar were defined as “pseudogene lineages” for the PAML analysis, with all other lineages being defined as “ORF lineages.” The second part of the PAML analyses using V1RL ORFs alone excluded the pseudogenes. GenBank accession numbers for published human and marmoset V1RL pseudogene sequences are as follows: Human V1RL1: AF255342; Human V1RL2: AF370359; Human V1RL3: AF336873; Human V1RL4: AY114733; Human V1RL5: AY114735; Human PH2: AF253312; Human PHB4C5: AF253314; Human PH2D1: AF253315; Human PhH8: U73853; Human PHRET: AF253316; Human PhH5: U73852; Human PHA4: AF253313; Marmoset MsM11: AF397897; Marmoset MsM4: AF397898; Marmoset MsM5: AF397899; Marmoset MsM6: AF397900; Marmoset MsM7: AF397901
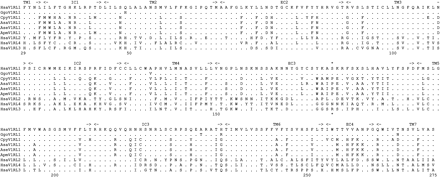
Alignment of V1RL ORF protein sequences. Numbering follows human V1RL1, and only the region sequenced in this study is shown. The position marked by an asterisk (172) is inferred to be under positive selection (see text for details). Species abbreviations are as follows: Hsa, human; Ggo, gorilla; Cpy, pygmy marmoset; Asa, Bolivian red howler; Ase, red howler; Apm, mantled howler
Maximum Likelihood Estimates of dN/dS Ratios (ω) Using PAML: Lineage-Specific Models Over All Sites (18 ORF + Pseudogene Lineages).
Model . | ω1 . | ω2 . | Log Likelihood . |
---|---|---|---|
A: One ratio | 0.69 | — | −4384.35 |
B: 2 ratios | |||
ωORF lineages, ωpseudogenelineages | 0.64 | 1.02 | −4382.17 |
C: 2 ratios | |||
ωORF lineages = 1, ωpseudogenelineages | (1.0)a | 1.03 | −4393.02 |
Model . | ω1 . | ω2 . | Log Likelihood . |
---|---|---|---|
A: One ratio | 0.69 | — | −4384.35 |
B: 2 ratios | |||
ωORF lineages, ωpseudogenelineages | 0.64 | 1.02 | −4382.17 |
C: 2 ratios | |||
ωORF lineages = 1, ωpseudogenelineages | (1.0)a | 1.03 | −4393.02 |
aValues in parentheses are fixed, not estimated.
Maximum Likelihood Estimates of dN/dS Ratios (ω) Using PAML: Lineage-Specific Models Over All Sites (18 ORF + Pseudogene Lineages).
Model . | ω1 . | ω2 . | Log Likelihood . |
---|---|---|---|
A: One ratio | 0.69 | — | −4384.35 |
B: 2 ratios | |||
ωORF lineages, ωpseudogenelineages | 0.64 | 1.02 | −4382.17 |
C: 2 ratios | |||
ωORF lineages = 1, ωpseudogenelineages | (1.0)a | 1.03 | −4393.02 |
Model . | ω1 . | ω2 . | Log Likelihood . |
---|---|---|---|
A: One ratio | 0.69 | — | −4384.35 |
B: 2 ratios | |||
ωORF lineages, ωpseudogenelineages | 0.64 | 1.02 | −4382.17 |
C: 2 ratios | |||
ωORF lineages = 1, ωpseudogenelineages | (1.0)a | 1.03 | −4393.02 |
aValues in parentheses are fixed, not estimated.
Maximum Likelihood Estimates of dN/dS Ratios (ω) Using PAML: Site-Specific Models Over All Lineages (9 ORF Lineages).
Model . | ω1 . | ω2 . | ω3 . | Log Likelihood . |
---|---|---|---|---|
M0 (one ratio) | 0.61 | — | — | −3571.10 |
M1 (neutral) | (0.00)a | (1.00)a | — | −3556.39 |
p1b = 0.17 p2 = 0.83 | ||||
M2 (selection) | (0.00)a | (1.00)a | 4.75c | −3551.06 |
p1 = 0.16 p2 = 0.78 p3 = 0.05 | ||||
M3 (discrete) | 0.00 | 0.56 | 2.22c | −3543.21 |
p1 = 0.11 p2 = 0.72 p3 = 0.16 | ||||
M7 (Beta) | pd = 0.60 q = 0.35 | −3548.57 | ||
M8 (Beta + ω) | p = 0.89 q = 0.66 p0 = 0.91 | −3543.60 | ||
ω = 2.73c p1 = 0.09 |
Model . | ω1 . | ω2 . | ω3 . | Log Likelihood . |
---|---|---|---|---|
M0 (one ratio) | 0.61 | — | — | −3571.10 |
M1 (neutral) | (0.00)a | (1.00)a | — | −3556.39 |
p1b = 0.17 p2 = 0.83 | ||||
M2 (selection) | (0.00)a | (1.00)a | 4.75c | −3551.06 |
p1 = 0.16 p2 = 0.78 p3 = 0.05 | ||||
M3 (discrete) | 0.00 | 0.56 | 2.22c | −3543.21 |
p1 = 0.11 p2 = 0.72 p3 = 0.16 | ||||
M7 (Beta) | pd = 0.60 q = 0.35 | −3548.57 | ||
M8 (Beta + ω) | p = 0.89 q = 0.66 p0 = 0.91 | −3543.60 | ||
ω = 2.73c p1 = 0.09 |
aValues in parentheses are fixed, not estimated.
b“p” indicates proportion of sites with that value of ω.
cValues in bold indicate positive selection.
d“p” and “q” under models M7 and M8 indicate parameters defining the beta function.
Maximum Likelihood Estimates of dN/dS Ratios (ω) Using PAML: Site-Specific Models Over All Lineages (9 ORF Lineages).
Model . | ω1 . | ω2 . | ω3 . | Log Likelihood . |
---|---|---|---|---|
M0 (one ratio) | 0.61 | — | — | −3571.10 |
M1 (neutral) | (0.00)a | (1.00)a | — | −3556.39 |
p1b = 0.17 p2 = 0.83 | ||||
M2 (selection) | (0.00)a | (1.00)a | 4.75c | −3551.06 |
p1 = 0.16 p2 = 0.78 p3 = 0.05 | ||||
M3 (discrete) | 0.00 | 0.56 | 2.22c | −3543.21 |
p1 = 0.11 p2 = 0.72 p3 = 0.16 | ||||
M7 (Beta) | pd = 0.60 q = 0.35 | −3548.57 | ||
M8 (Beta + ω) | p = 0.89 q = 0.66 p0 = 0.91 | −3543.60 | ||
ω = 2.73c p1 = 0.09 |
Model . | ω1 . | ω2 . | ω3 . | Log Likelihood . |
---|---|---|---|---|
M0 (one ratio) | 0.61 | — | — | −3571.10 |
M1 (neutral) | (0.00)a | (1.00)a | — | −3556.39 |
p1b = 0.17 p2 = 0.83 | ||||
M2 (selection) | (0.00)a | (1.00)a | 4.75c | −3551.06 |
p1 = 0.16 p2 = 0.78 p3 = 0.05 | ||||
M3 (discrete) | 0.00 | 0.56 | 2.22c | −3543.21 |
p1 = 0.11 p2 = 0.72 p3 = 0.16 | ||||
M7 (Beta) | pd = 0.60 q = 0.35 | −3548.57 | ||
M8 (Beta + ω) | p = 0.89 q = 0.66 p0 = 0.91 | −3543.60 | ||
ω = 2.73c p1 = 0.09 |
aValues in parentheses are fixed, not estimated.
b“p” indicates proportion of sites with that value of ω.
cValues in bold indicate positive selection.
d“p” and “q” under models M7 and M8 indicate parameters defining the beta function.
. | Hypothesis Tested . | Models Compared . | Likelihood Ratio Statistic: −2(L1−L2) . | df . |
---|---|---|---|---|
I | ωORF lineages < 1 | B versus C | 21.70** | 1 |
II | ω > 1 for a proportion of sites | M1 versus M2 | 10.66** | 2 |
III | ω > 1 for a proportion of sites | M1 versus M3 | 26.36** | 4 |
IV | ω > 1 for a proportion of sites | M7 versus M8 | 9.94** | 2 |
. | Hypothesis Tested . | Models Compared . | Likelihood Ratio Statistic: −2(L1−L2) . | df . |
---|---|---|---|---|
I | ωORF lineages < 1 | B versus C | 21.70** | 1 |
II | ω > 1 for a proportion of sites | M1 versus M2 | 10.66** | 2 |
III | ω > 1 for a proportion of sites | M1 versus M3 | 26.36** | 4 |
IV | ω > 1 for a proportion of sites | M7 versus M8 | 9.94** | 2 |
** Significant at the 1% level.
. | Hypothesis Tested . | Models Compared . | Likelihood Ratio Statistic: −2(L1−L2) . | df . |
---|---|---|---|---|
I | ωORF lineages < 1 | B versus C | 21.70** | 1 |
II | ω > 1 for a proportion of sites | M1 versus M2 | 10.66** | 2 |
III | ω > 1 for a proportion of sites | M1 versus M3 | 26.36** | 4 |
IV | ω > 1 for a proportion of sites | M7 versus M8 | 9.94** | 2 |
. | Hypothesis Tested . | Models Compared . | Likelihood Ratio Statistic: −2(L1−L2) . | df . |
---|---|---|---|---|
I | ωORF lineages < 1 | B versus C | 21.70** | 1 |
II | ω > 1 for a proportion of sites | M1 versus M2 | 10.66** | 2 |
III | ω > 1 for a proportion of sites | M1 versus M3 | 26.36** | 4 |
IV | ω > 1 for a proportion of sites | M7 versus M8 | 9.94** | 2 |
** Significant at the 1% level.
We thank Andrew Kitchener, Liliana Cortés-Ortiz, Alcides Pissinatti, Jim Dietz, and Mike Bruford for providing samples. Brian Golding and two anonymous reviewers made helpful comments that greatly improved the manuscript. This work was funded by the Biotechnology and Biological Sciences Research Council.
Literature Cited
Anisimova, M., J. P. Bielawski, and Z. Yang.
Baum, J., R. H. Ward, and D. J. Conway.
Bielawski, J. P., and Z. Yang.
Dulac, C., and R. Axel.
Giorgi, D., C. Friedman, B. J. Trask, and S. Rouquier.
Giorgi, D., and S. Rouquier.
Holbrook, J. D., G. M. Birdsey, Z. Yang, M. W. Bruford, and C. J. Danpure.
Hughes, A. L., and M. Nei.
Huttley, G. A., et al.
Jacob, S., M. K. McClintock, B. Zelano, and C. Ober.
Johnson, M. E., L. Viggiano, J. A. Bailey, M. Abdul-Rauf, G. Goodwin, M. Rocchi, and E. E. Eichler.
Kourso-Mehr, H., S. Pintchovski, J. Melnyk, Y. J. Chen, C. Frideman, B. Trask, and H. Shizuya.
Lane, R. P., T. Cutforth, R. Axel, L. Hood, and B. J. Trask.
Messier, W., and C.-B. Stewart.
Rodriguez, I., K. Del Punta, A. Rothman, T. Ishii, and P. Mombaerts.
Rodriguez, I., C. A. Greer, Y. Mok, and P. Mombaerts.
Rodriguez, I., and P. Mombaerts.
Rouquier, S., A. Blancher, and D. Giorgi.
Shyue, S. K., D. Hewett-Emmett, H. G. Sperling, D. M. Hunt, J. K. Bowmaker, J. D. Mollon, and W.-H. Li.
Surridge, A. K., and N. I. Mundy.
Suzuki, Y., and M. Nei.
Swofford, D.
Wedekind, C., T. Seebeck, F. Bettens, and A. J. Papepke.
Whinnett, A., and N. I. Mundy.
Wyckoff, G. J., W. Wang, and C.-I. Wu.
Yang, Z.
Yang, Z.
Yang, Z., Nielsen, R., Goldman, N., and Pedersen, A.-M. K.