-
PDF
- Split View
-
Views
-
Cite
Cite
Ana Rodríguez-Muñoz, Rebeca Martínez-Hernández, Ana M. Ramos-Leví, Ana Serrano-Somavilla, Roberto González-Amaro, Francisco Sánchez-Madrid, Hortensia de la Fuente, Mónica Marazuela, Circulating Microvesicles Regulate Treg and Th17 Differentiation in Human Autoimmune Thyroid Disorders, The Journal of Clinical Endocrinology & Metabolism, Volume 100, Issue 12, 1 December 2015, Pages E1531–E1539, https://doi.org/10.1210/jc.2015-3146
- Share Icon Share
Microvesicles (MVs) are emerging as important contributors to the development of inflammatory and autoimmune diseases. MVs can mediate immune modulation carrying genetic information, including microRNAs that can be transferred between cells.
We determined the plasma levels of annexin-V+ MVs derived from different immune cells and platelets in patients with autoimmune thyroid diseases (AITDs) and in healthy controls. T lymphocyte polarization assays were performed in the presence of MVs to evaluate their effect in T regulatory and T helper 17 cells differentiation. microRNA content into plasma MVs and their corresponding mRNA targets were evaluated by RT-PCR.
The percentage of platelet-derived MVs (CD41a+) was significantly increased in plasma samples from AITD patients compared with healthy controls. In contrast, patients with AITD showed a lower percentage of leukocyte and endothelial cell-derived MVs compared with controls. In addition, functional assays showed that MVs from AITD patients inhibited the in vitro differentiation of Foxp3+ T regulatory cells (11.35% vs 4.40%, P = .01) and induced the expression of interferon-γ by CD4+ lymphocytes (10.91% vs 13.99%, P = .01) as well as the differentiation of T helper 17 pathogenic (IL-17+interferon-γ+) cells (1.98% vs 5.13%, P = .03). Furthermore, in AITD patients, whereas miR-146a and miR-155 were increased in circulating MVs, their targets IL-8 and SMAD4 were decreased in peripheral blood mononuclear cells.
Our data indicate that circulating MVs seem to have a relevant role in the modulation of the inflammatory response observed in AITD.
Autoimmune diseases result from the failure of the immune system to develop tolerance toward self-antigens. In autoimmune thyroid diseases (AITDs), Hashimoto's thyroiditis (HT), and Graves' disease (GD), the immune regulation is compromised, leading to lymphocytic infiltration of the thyroid, production of antibodies against thyroid antigens, and thyroid dysfunction (1). Increasing evidence indicates a role of T regulatory (Treg) and T helper (Th)-17 cells in the immunopathogenesis of these diseases. In this regard, an altered number and/or function of Treg and Th17 lymphocytes have been reported in AITDs (2–4). However, the mechanisms involved in these processes are not clear.
Microvesicles (MVs), also known as microparticles or ectosomes, are a heterogeneous population of vesicles (0.1–1.0 μm), which are released via budding from the plasma membrane under different conditions (5). MVs are found in body fluids such as plasma, serum, or urine. Most MVs in peripheral blood derive from platelets, endothelial cells, and leukocytes, being the platelets' major source. The levels of circulating MVs have been found to be modified in several diseases including autoimmune disorders, cancer, or cardiovascular diseases (6), suggesting their potential as biomarkers. After having been considered for a long time as inert cell debris, recent studies have demonstrated that circulating MVs appear to be important for intercellular communication (7). In this regard, MVs retain certain membrane and cytoplasmic components, including cytosolic proteins, lipids, DNA, mRNA, and microRNAs (miRNAs) from their parental cells and are capable of transferring bioactive molecules to neighboring cells modulating biological processes including inflammatory and autoimmune disorders (8).
MVs are gaining importance as a source of miRNAs in systemic circulation. miRNA-mediated gene regulation is involved in several physiological processes such as cell proliferation, differentiation, apoptosis, or immune functions (9–11), and their dysregulation has been described in different pathological conditions (12). Currently it has become evident that miRNAs regulate many aspects of the immune response because patients with autoimmune disorders exhibit differential patterns of miRNA expression compared with healthy subjects, suggesting a relevant role in the pathogenesis of autoimmune diseases (12). Because MVs have been involved as mediators of different processes including the immune response, we decided to analyze the circulating levels of MVs and their miRNA content in AITD patients as well as their possible in vitro effect on T cell differentiation.
Materials and Methods
Patients and healthy subjects
Sixty-two AITD patients (33 GD and 29 HT) were studied. Diagnosis was established on commonly accepted clinical, laboratory, and histological criteria (1). Forty-five healthy subjects were included as controls. Serum free T4, TSH, and levels of antibodies against thyroglobulin, thyroperoxidase, and thyrotropin receptor were measured as previously described (13). Complete clinical and demographic data were registered in all subjects (Supplemental Table 1). All individuals signed a written informed consent, and this study was approved by the Internal Ethical Review Committee of the Hospital de La Princesa.
Microvesicles isolation
Blood for sampling of MVs was collected into tubes containing lithium heparin (BD Vacutainer). MVs were obtained by differential centrifugations. First, blood cells were removed by pelleting by centrifugation at 1500 × g for 20 minutes, and the supernatant obtained was recentrifuged at 1500 × g for 20 minutes to remove platelets. Then the resulting platelet-poor plasma was centrifuged at 18 500 × g for 30 minutes to collect the MVs. The size distributions of MV samples were determined by nanoparticle tracking analysis (NTA) using Nanosight LM10 and NTA 2.3 software (Nanosight).
Apoptosis induction and apoptotic bodies' isolation
To induce apoptosis, peripheral blood mononuclear cells (PBMCs) were treated with staurosporine 1 μM (Abcam) for 24 hours. Apoptotic bodies (ABs) were isolated from supernatants of PBMCs as described (14).
Protein extraction and immunoblotting analysis
MV and AB samples were examined by Western blot for detection of Histone H3. MVs and ABs were harvested and resuspended in radioimmunoprecipitation assay buffer containing a protease and phosphatase inhibitor cocktail (Roche Molecular Biochemicals). After 30 minutes on ice, lysates were centrifuged at 18 500 × g for 15 minutes and the supernatant was recovered. Then protein concentration was determined by the Bio-Rad protein assay (Bio-Rad Laboratories). Protein samples (30–50 μg) were separated and transferred to nitrocellulose membranes, which were blocked and incubated with antihistone H3 (Cell Signaling Technology) and anti-β-actin (Sigma-Aldrich). Membranes were then probed with respective secondary antibodies conjugated to horseradish peroxidase and visualized using a chemiluminescent detection reagent kit (ThermoScientific).
Microvesicles labeling and flow cytometry analysis
To analyze blood-MVs by flow cytometry, an MV pellet obtained from 1 mL of plasma was resuspended in annexin-V binding buffer (BD Pharmingen) and centrifuged at 18 500 × g for 30 minutes. The MV pellet was then resuspended in 1 mL of annexin-V binding buffer. A sample of MVs (50 μL) was incubated with allophycocyanin-conjugated annexin-V (BD Pharmingen) and one of the following monoclonal antibodies (mAbs): CD45, major histocompatibility complex (MHC) class II (Biolegend), CD14, CD41a (BD Pharmingen), or CD62E (eBioscience) (all of them PE conjugated) for 15 minutes in the dark. Isotype-matched irrelevant mAb phycoerythrin (PE)-conjugated (BD Pharmingen) was used as negative control. MVs incubated with annexin-V in a calcium-free buffer was included as negative control of annexin binding. All buffers and antibodies used were filtered through 0.2-μm pore-size filters to limit the background noise. The MV gate was set using 0.2-μm and 1-μm FluoSpheres beads (Life Technologies) in a forward-scatter and side-scatter dot plot with logarithm scale. Electronic background noise was excluded using the signal obtained with filtered buffer alone. Negative events for annexin-V were used to adjust the threshold in the corresponding fluorescence channel. Thus, MVs were identified as those events between 0.2 and 1 μm in size and positive staining for annexin-V. To calculate the number of MVs, 50 μL of SPHERO AccuCount fluorescent particles (Spherotech) was added to each sample immediately prior to acquisition in the FACSCanto flow cytometer (Becton Dickinson). The number of MVs per milliliter of plasma was estimated using the following formula: (A/B) × (C/D), where A is the number of events in the corresponding MVs gate, B is the number of events for the AccuCount particles, C is the number of AccuCount particles per 50 μL, and D is the volume of test sample. The acquisition was stopped when at least 1000 bead events were counted and each sample was run in the low flow rate mode. Data were analyzed using the FlowJo software version 8.7.3.
Purification of CD4+ T cells
Naïve CD4+ T lymphocytes were isolated from peripheral blood from healthy subjects by negative selection using magnetic beads (Miltenyi Biotec). Cell purity was always greater than 90%. Cells were resuspended in complete RPMI 1640 culture medium.
Th17 and Treg differentiation
Purified naïve CD4+ T cells (2 × 105) were cultured in a plate precoated with anti-CD3 (5 μg/mL) and anti-CD28 antibodies (2 μg/mL), and for Th17 differentiation, cells were incubated with IL-6 (10 ng/mL), IL-1β (10 ng/mL), TGF-β1 (1 ng/mL), neutralizing anti-IL-4 (1 μg/mL), and anti-interferon (IFN)-γ (1 μg/mL) in the presence or absence of circulating MVs corresponding to 1 mL of plasma. At 5 days, fresh medium with IL-2 (20 U/mL) and IL-23 (10 ng/mL) was added for an additional 5 days. Fully differentiated Th17 cells were stained with anti-IL-17 and anti-IFN-γ and analyzed in a FACSCalibur flow cytometer (Becton Dickinson). For differentiation of Treg cells, purified CD4+ lymphocytes were cultured during 5 days with TGF-β1 (5 ng/mL) and IL-2 (20 U/mL) in the presence or absence of circulating MVs corresponding to 1 mL of plasma in a plate previously coated with anti-CD3 (1 μg/mL) and anti-CD28 (1 μg/mL). At the end of differentiation, cells were labeled with anti-CD25 (Biolegend) and anti-Foxp3 (eBioscience). Samples were analyzed by flow cytometry.
Analysis of miRNAs by real-time quantitative RT-PCR (qRT-PCR)
miRNAs were extracted from MVs using an RNeasy minikit (QIAGEN), according to the manufacturer's protocols. cDNA was synthesized using a universal cDNA synthesis kit for RT-PCR (Exiqon), following the manufacturer's instructions. Expression of miR-146a-5p, miR-155–5p, miR-326, and miR-150–5p was performed using specific probes and a universal reverse transcription miRNA PCR ExiLENT SYBR Green master mix (both from Exiqon) with the CFX384 Touch real-time PCR detection system (Bio-Rad Laboratories). 5S and RNAU1A1 were used as reference genes. Gene expression levels were compared using 2-δδCt method.
miRNA targets expression
Expression of IL-8 and SMAD4 mRNA was determined in PBMCs from AITD patients and healthy controls. Total RNA was isolated from peripheral blood with NucleoSpin RNA blood (Macherey-Nagel). cDNA was synthesized from total RNA (500 ng) using a high-capacity cDNA reverse transcription kit with a ribonuclease inhibitor (Life Technologies), following the manufacturer's instructions. IL-8 and SMAD4 cDNAs were amplified using the Power SYBR Green PCR master mix (Life Technologies). Normalization was carried out with the internal standard hypoxanthine-guanine phosphoribosyl transferase.
Statistical analysis
Data were analyzed using the GraphPad Prism 4 software (GraphPad Software) and SPSS software version 16.0 (SPSS). Nonparametric tests were used to compare differences between groups. Data were expressed as the median and interquartile (Q1-Q3) range or mean ± SEM. Values of P < .05 were considered as statistically significant.
Results
Characterization of circulating MVs
MVs isolated from plasma were characterized according to size distribution by NTA using a Nanosight instrument. This analysis revealed that MV population size ranged from 50 to 600 nm in diameter (Figure 1A). Purity of MVs was confirmed by Western blot; histone H3 expression, a marker for apoptotic bodies, was absent in MV preparation (Figure 1B). MV isolation was also confirmed by flow cytometry using 0.2 and 1 μm FluoSpheres beads (Life Technologies). The upper-size limit was defined by using 1 μm beads in a logarithmic forward scatter-logarithmic side scatter dot plot, whereas the lower size was established with the noise signal obtained by calcium-free buffer alone and the 0.2-μm beads. Next, events positive for annexin-V binding were selected, and only those events included in these gates were analyzed for specific cell surface marker (Figure 1C).
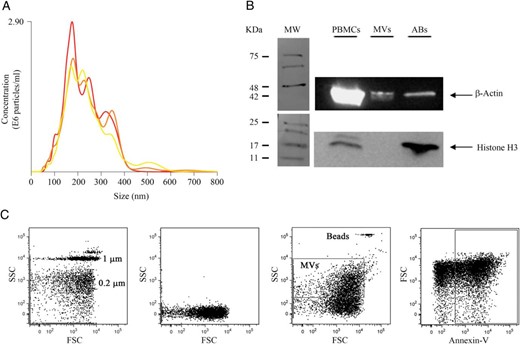
Characterization of circulating MVs.
MVs were isolated from plasma samples by serial centrifugations A, NTA used to determined particle size. B, Western blotting analysis of histone H3 in protein extracts obtained from ABs and MVs. Lysates from PBMCs were used as a positive control. C, Flow cytometry strategy showing the gating for annexin-V+ MVs. From left to right, plot of forward (FSC) and side scatter (SSC) using 0.2 and 1 μm FluoSpheres beads (Life Technologies), calcium-free buffer alone, gated MVs, and AccuCount fluorescent particles (Spherotech) used to their quantification and finally MVs stained with annexin-V.
Levels of circulating MVs are altered in AITD patients
Plasma levels of annexin-V+ MVs were assessed in 30 patients (18 GD and 12 HT), and 28 healthy subjects by flow cytometry. Figure 2A shows representative dot plots illustrating the gating strategy used. Although the number of MVs tended to be higher in AITD patients compared with controls, no significant differences were detected (Figure 2B). The percentage of MVs derived from different cellular sources was also analyzed, and a significant decrease in the proportion of circulating MVs derived from monocytes (CD14+), endothelial cells (CD62E+), leukocytes (CD45+), and antigen presenting cells (MHC-II+) was observed in AITD patients compared with controls (P = .0096, P = .020, P = .010, and P = .022, respectively, Figure 2, C–F). In contrast, the percentage of platelet-derived MVs (CD41a+) was increased in patients with AITD (P = .028, Figure 2G). When patients were classified as GD and HT, only samples from GD patients showed a significant decrease in leukocytes and antigen-presenting cell-derived MVs (P = .019 and P = .0039, respectively, Figure 2, E and F). On the other hand, HT patients were responsible for the increased levels of platelet-derived MVs (P = .030, Figure 2G) and decreased levels of those derived of monocytes and endothelial cells (P = .011 and P = .030, respectively, Figure 2, C and D) observed in AITD patients. No significant associations between the percentage of these subpopulations of MVs and the serum levels of free T4, TSH, or thyroid antibodies were found (data not shown).
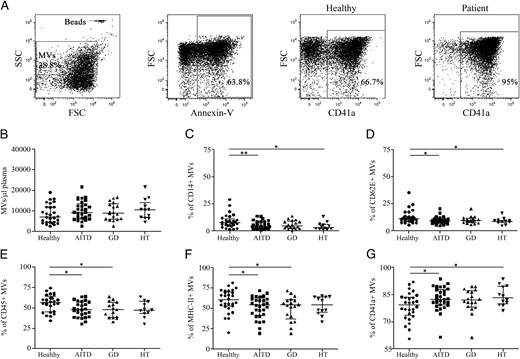
Levels of circulating MVs are altered in AITD patients.
Blood-MVs were isolated from plasma samples as indicated in Materials and Methods. A, Representative dot plots for the analysis of MVs derived from platelets. MVs were incubated with allophycocyanin-conjugated annexin-V and one of following PE-conjugated mAbs: CD14, CD62E, CD45, MHC class II, or CD41a as described in Materials and Methods. AccuCount fluorescent particles (Spherotech) were used for quantitative estimation. Levels of circulating MVs in AITD and healthy controls (B), monocytes (CD14+), (D) endothelial cells (CD62E+) (C), leukocytes (CD45+) (F), antigen-presenting cells (MHC-II+) (E), and platelet (CD41a+)-derived MVs (G) in AITD (HT+GD), HT, and GD patients and healthy subjects are shown. Differences between groups were determined by a Mann-Whitney U test. Results are from 30 AITD patients (18 GD and 12 HT) and 28 healthy subjects. *, P < .05; **, P < .01.
MVs modulate the differentiation of Treg and Th17 cells
Next, we analyzed the effect of circulating MVs on human CD4+ T cell in vitro differentiation. For this purpose, human Foxp3+ Treg and Th17 cells were induced in the presence or absence of MVs isolated from plasma of AITD patients and control subjects. Our results showed that circulating MVs from AITD patients were able to inhibit Treg cell differentiation (P = .019, Figure 3). In contrast, although MVs from healthy subjects seemed to reduce the induction of CD25+Foxp3+ cells, this difference was not significant (Figure 3, A and B). Thus, a significant difference in the percentage of CD25+Foxp3+ cells was found in AITD patients when compared with healthy subjects (P = .041, Figure 3B). Classification of patients with GD and HT demonstrated that only MVs from HT patients significantly inhibited Treg cell differentiation (P = .010). The MV effect was also tested on Th17 lymphocyte differentiation (Figure 4). MVs from both AITD patients and healthy controls induced a significant increase in the percentage of IL-17-producing cells (P = .025 and P = .016, respectively, Figure 4B), and no significant differences between patients and controls were detected. However, MVs isolated from AITD patients induced an increment of IFN-γ+ cells compared with MVs obtained from healthy subjects, which did not show any effect on IFN-γ expression (P = .015, Figure 4C). Classification of patients with GD and HT indicated that GD patients were responsible only for the difference in IFN-γ+ cells observed between AITD and healthy subjects (P = .049, Figure 4C). Interestingly, only MVs from AITD patients induced a significant differentiation of IL-17+IFN-γ+ double-positive cells (P = .030, Figure 4D), which very likely correspond to the pathogenic Th17 lymphocytes (15–17). The induction of this double-positive subpopulation was significant only in GD patients (P = .036).
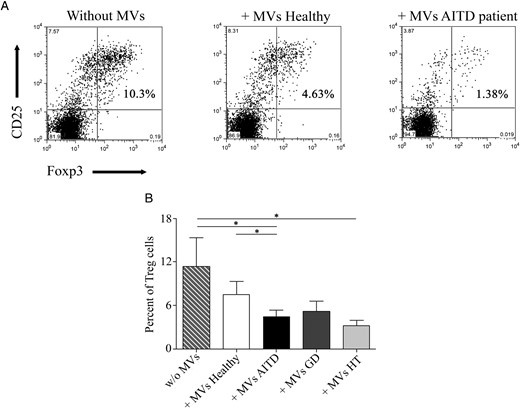
MVs from AITD patients regulate CD25+Foxp3+ Treg cell differentiation.
Naïve CD4+ T cells from healthy donors were incubated under Treg differentiation conditions in the presence of absence of circulating MVs corresponding to 1 mL of plasma. A, Representative flow cytometry dot plot analysis showing the percentage of CD25+Foxp3+ cells in Treg polarization cultures with or without MVs obtained from healthy subjects and AITD patients. B, Percentage of CD25+Foxp3+ Treg cells as in panel A. Data are from 21 patients with AITD (13 GD and eight HT) and 15 healthy controls. Significant differences were determined by a Mann-Whitney U test. Results are expressed as the mean ± SEM. *, P < .05.
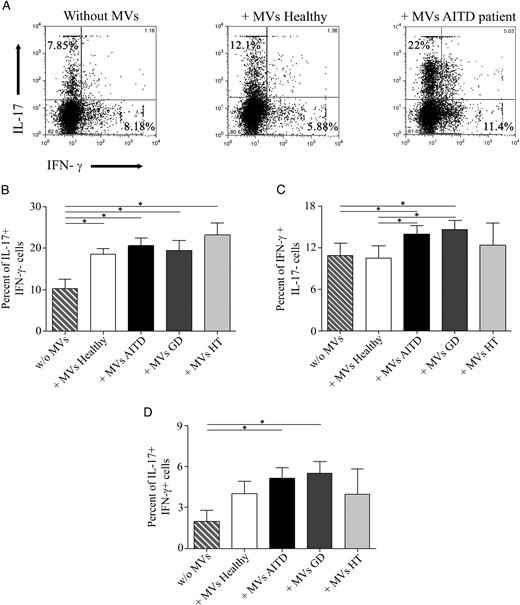
MVs increase the levels of IL-17+ and IFN-γ cells.
Effect of plasma MVs was evaluated in Th17 differentiation assays. A, Flow cytometry dot plot in which the expression of IL-17 and IFN-γ without MVs or with MVs isolated from one healthy subject and AITD patient is shown. Percentage of IL-17+IFN-γ− (B), IFN-γ+IL-17− (C), and IL-17+IFN-γ+ cells (D) in 24 AITD patients (17 GD and seven HT) and 19 healthy subjects. Differences between groups were determined by a Mann-Whitney U test. Results are expressed as the mean ± SEM. *, P < .05.
MVs from AITD patients are enriched in miRNA-155 and miRNA-146a
Recently the role of MVs as carriers of genetic material and miRNAs as regulators of the immune response has emerged (8). Thus, we evaluated the expression of miRNAs that are involved in different functions of the immune system. Expression of miR-146a, miR-155, miR-326, and miR-150 was evaluated in circulating MVs from 15 AITD patients (eight with GD and seven with HT) and 13 healthy subjects. We found that miR-146a was significantly up-regulated in patients with AITD in both GD and HT patients compared with controls (P = .0016 and P = .0009, respectively, Figure 5A), whereas the levels of miR-155 were higher in HT patients compared with healthy subjects (P = .047, Figure 5B). No significant differences were found in the expression of miR-326 and miR-150 (Figure 5, C and D).
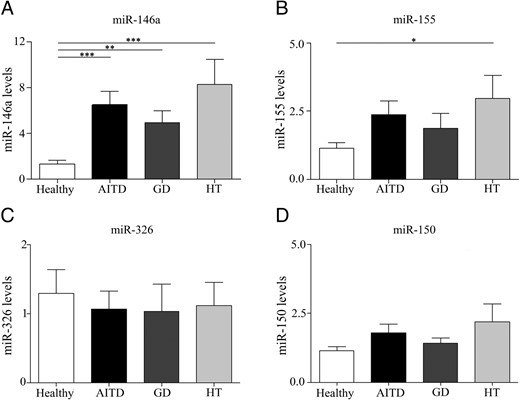
MVs from AITD patients are enriched in miRNA-155 and miRNA-146a.
A–D, miRNA expression from MVs obtained from 15 AITD patients (eight GD and seven HT) and 13 healthy individuals was determined by qRT-PCR as described in Materials and Methods. Each experiment was performed in triplicate and results were analyzed normalizing with RNA 5S and RNAU1A1 using 2-δδCt method. Data are the mean ± SEM. *, P < .05; **, P < .01; ***, P < .001.
Frequencies of IL-8 and SMAD4 are decreased in AITD patients
We next assessed the genetic expression of miR-155 and miR-146a targets in PBMCs from patients and controls. Thus, we conducted a qRT-PCR analysis of IL-8 and SMAD4 expression on peripheral blood samples from 20 AITD patients (10 GD and 10 HT) and eight healthy controls. We found a decreased expression of IL-8 and SMAD4 in patients with AITD compared with healthy individuals (P = .034 and P = .015, respectively, Figure 6, A and B). In the case of SMAD4, the decrease was found only in HT patients (P = .0014, Figure 6B).
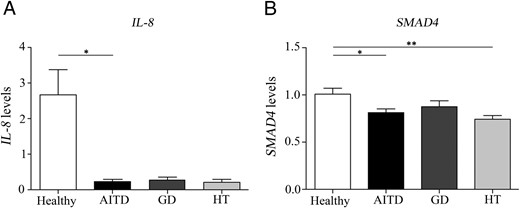
Frequencies of IL-8 and SMAD4 are decreased in AITD patients.
A and B, mRNA expression of IL-8 and SMAD4 were studied in PBMCs from 20 patients with AITD (10 GD and 10 HT) and eight healthy individuals by qRT-PCR. Each experiment was performed in triplicate and data were analyzed normalizing with hypoxanthine-guanine phosphoribosyl transferase mRNA expression using the 2-δδCt method. Data are the mean ± SEM. *, P < .05; **, P < .01.
Discussion
In this study, we demonstrate that circulating MVs from AITD patients have a functional role and can inhibit Treg differentiation but favor IFN-γ production by CD4+ T cells.
Here we assessed the levels of different types of MVs by flow cytometry. Although many studies have evaluated the MV levels in the plasma of patients with different autoimmune disorders, our knowledge about MVs in AITD is scarce. In a recent publication, elevated values of MVs have been described in patients with GD, even after treatment with thiamazole (18). In contrast, our data indicate that the number of total of MVs is similar in AITD patients and controls. These results could be partly explained by differences in the stage of the disease, analytical variables, and/or the lack of standardization methods for MV analysis.
Both GD and HT are organ-specific autoimmune diseases, with different thyroid antigens as target for the immune response. However, the effect of extracellular vesicles (EVs) in AITD is probably not antigen specific because their role in the immune response has been described in other autoimmune diseases, mainly those classified as systemic disorders (19). We have previously described an altered proportion and a defective function of Treg cells in both the peripheral blood and thyroid gland from patients with AITD (2, 3) as well as enhanced levels of Th17 cells and Th17 cytokines (4). For this reason, we explored whether MVs could have a significant effect on T cell differentiation toward these two lymphocyte subpopulations. Although we found that MVs from AITD patients are able to inhibit Treg cell differentiation (a phenomenon not detected in MVs from healthy subjects), MVs from patients and controls show a similar in vitro effect on the expression of IL-17. However, only MVs from AITD induced IFN-γ expression as well as the differentiation of IL-17+IFN-γ+ double-positive lymphocytes. In this regard, it has been demonstrated that the administration of EVs enhances the in vivo production of Th1 and Th17 cytokines in a model of airway immune dysfunction (20). Our functional assays support the role of MVs in the regulation of the immune response that could be involved in homeostasis maintenance. We consider that the results obtained in the in vitro functional assays performed are very relevant because, on the one hand, it is evident that Treg cells have a key role on the suppression of autoimmune reactivity, and, on the other hand, pathogenic (IL-17+IFN-γ+) Th17 cells have an important effect in the inflammatory phenomenon seen in different autoimmune diseases.
Recent studies have identified the intriguing possibility that cells can alter the expression of genes in neighboring and distant cells by transferring genetic information (7). We postulated that specific miRNAs carried by MVs could be implicated in Treg and Th17 differentiation and found that the levels of miR-146a and miR-155 were increased in MVs from AITD patients compared with healthy subjects. Recent reports have confirmed significant variations of miRNA 146a, miRNA 200a, and miRNA155 in CD4+ and CD8+ T cells of patients with GD and HT (21). miR-146a and miR-155 are emerging as important modulators of the differentiation and function of cells of the innate and adaptive immunity. Both miRNAs are highly expressed in Treg cells and are critical for function and development of Treg cells (22). Recent studies have demonstrated that miR-155 also promotes the development of inflammatory T cells including Th17 cells (23) and contributes to Th17 cell function (24).
miRNAs regulate the immune response by targeting the mRNAs of genes involved in T-cell development, proliferation, differentiation, and function (25). IL-8 and SMAD4 are transcripts subject to posttranscriptional control by miRNAs, among which are miR-146a and miR-155 (26, 27). Consequently, we decided to explore the frequencies of these genes in AITD and found a significant decrease of both genes in AITD patients compared with controls. Previous studies have demonstrated that SMAD4-deficient Th cells showed reduced Foxp3 expression compared with wild-type T cells (28). In addition, SMAD4 deficiency in T cells leads to the Th17-associated development of premalignant gastroduodenal lesions in mice (29). The in vivo function of EVs containing miRNAs (EV-miRNAs) is currently an important field of research, and there has been debate about the ability of these molecules to mediate effects at a distance. However, increasing evidence suggest the regulatory role of EVs-miRNAs in vivo (30). It is conceivable that miR-146a and miR-155 in circulating MVs from AITD down-regulate the expression of SMAD4 in PBMCs. In turn, deficiency of SMAD4 may be responsible of the Treg/Th17 imbalance observed in these patients.
In summary, our data indicate that MVs may have a relevant role in AITD as modulators of the immune response, mainly through the inhibition of Treg cell differentiation and the induction of Th17 cells. miRNAs contained within MVs may be involved in this immune process by targeting specific targets. These findings represent a meaningful advance in the field of autoimmunity and contribute to a better characterization of the pathophysiology of MVs and AITD.
Acknowledgments
This work was supported by Proyectos de Investigación en Salud Grants PI13–01414 and PIE-0041/BIOIMID (supported by the Instituto de Salud Carlos III) and Grant S2011/BMD-2328 TIRONET (supported by the Comunidad de Madrid) (to M.M.); and Ayuda Predoctoral de Formación en Investigación en Salud Grant FI11/00668 (supported by the Instituto de Salud Carlos III) (to A.R.-M).
Disclosure Summary: The authors have nothing to disclose.
H.d.l.F. and M.M. contributed equally to this work.
Abbreviations
- AB
apoptotic body
- AITD
autoimmune thyroid disease
- EV
extracellular vesicle
- GD
Graves' disease
- HT
Hashimoto's thyroiditis
- IFN
interferon
- mAb
monoclonal antibody
- MHC
major histocompatibility complex
- miRNA
microRNA
- MV
microvesicle
- NTA
nanoparticle tracking analysis
- PBMC
peripheral blood mononuclear cell
- PE
phycoerythrin
- qRT-PCR
quantitative RT-PCR
- Th
T helper
- Treg
T regulatory.