-
PDF
- Split View
-
Views
-
Cite
Cite
Paul M. Beringer, Timothy J. Bensman, Henry Ho, Melissa Agnello, Nicole Denovel, Albert Nguyen, Annie Wong-Beringer, Rosemary She, Dat Q. Tran, Samuel M. Moskowitz, Michael E. Selsted, Rhesus θ-defensin-1 (RTD-1) exhibits in vitro and in vivo activity against cystic fibrosis strains of Pseudomonas aeruginosa, Journal of Antimicrobial Chemotherapy, Volume 71, Issue 1, January 2016, Pages 181–188, https://doi.org/10.1093/jac/dkv301
- Share Icon Share
Abstract
Chronic endobronchial infections with Pseudomonas aeruginosa contribute to bronchiectasis and progressive loss of lung function in patients with cystic fibrosis. This study aimed to evaluate the therapeutic potential of a novel macrocyclic peptide, rhesus θ-defensin-1 (RTD-1), by characterizing its in vitro antipseudomonal activity and in vivo efficacy in a murine model of chronic Pseudomonas lung infection.
Antibacterial testing of RTD-1 was performed on 41 clinical isolates of P. aeruginosa obtained from cystic fibrosis patients. MIC, MBC, time–kill and post-antibiotic effects were evaluated following CLSI-recommended methodology, but using anion-depleted Mueller–Hinton broth. RTD-1 was nebulized daily for 7 days to cystic fibrosis transmembrane conductance regulator (CFTR) F508del-homozygous mice infected using the agar bead model of chronic P. aeruginosa lung infection. In vivo activity was evaluated by change in lung bacterial burden, airway leucocytes and body weight.
RTD-1 exhibited potent in vitro bactericidal activity against mucoid and non-mucoid strains of P. aeruginosa (MIC90 = 8 mg/L). Cross-resistance was not observed when tested against MDR and colistin-resistant isolates. Time–kill studies indicated very rapid, concentration-dependent bactericidal activity of RTD-1 with ≥3 log10 cfu/mL reductions at concentrations ≥4× MIC. No post-antibiotic effect was observed. In vivo, nebulized treatment with RTD-1 significantly decreased lung P. aeruginosa burden (mean difference of −1.30 log10 cfu; P = 0.0061), airway leucocytes (mean difference of −0.37 log10; P = 0.0012) and weight loss (mean difference of −12.62% at day 7; P < 0.05) when compared with controls.
This study suggests that RTD-1 is a promising potential therapeutic agent for cystic fibrosis airway disease.
Introduction
Chronic airway infection and inflammation are hallmarks of cystic fibrosis (CF) and contribute significantly to the morbidity and mortality in the CF population. Pseudomonas aeruginosa is the most common pathogen, chronically present in the lungs of nearly 80% of adults with CF.1 Epidemiological studies demonstrate a strong association between airway infection with mucoid P. aeruginosa and the ensuing progressive loss of lung function and shortened survival in these patients.2,3 Treatment of P. aeruginosa in patients with CF is challenging due to the development of resistance and adaptations that favour persistence over time within the lung environment of these patients. In particular, P. aeruginosa forms biofilms within the airways, which confer resistance to host defences and many antibiotics.4 In addition, resistance to existing antibiotics is of growing concern considering the lack of novel therapies for treatment of this organism. Approximately 25% of adults with CF are chronically infected with MDR P. aeruginosa.1 While chronic inhaled therapy with colistin or tobramycin has been shown to decrease hospitalization for acute pulmonary exacerbations, the emergence of P. aeruginosa strains resistant to these agents underscores the need for new drugs that are active against MDR P. aeruginosa without cross-resistance to existing antibiotics.5–7
θ-Defensins are cyclic cationic peptides present in leucocytes of Old World monkeys. Because of a premature termination mutation in the θ-defensin gene that appeared before the evolutionary appearance of hominids, θ-defensin expression is limited to Old World monkeys.8 Thus, it has been suggested that θ-defensins expressed in non-human primates might be exploited for use as therapeutics for human diseases.9 Rhesus θ-defensin-1 (RTD-1) exhibits potent microbicidal activity broadly against many bacteria, fungi, herpes simplex virus and HIV type 1.10,11 Of particular relevance to CF is the potent activity of RTD-1 against MRSA and P. aeruginosa.12 Systemic administration of RTD-1 in vivo demonstrated significantly improved survival in pre-clinical models of peritonitis and sepsis.13 Previously published data demonstrate RTD-1 does not cause haemolysis or cytotoxic effects against fibroblasts.11 Based on these promising data, the aim of the current study was to test the hypothesis that RTD-1 has therapeutic potential in CF airway infection. To accomplish this, the antipseudomonal activity of RTD-1 was characterized in vitro and its efficacy was evaluated in vivo through the use of a CF transmembrane conductance regulator (CFTR) F508del-homozygous murine model of chronic Pseudomonas lung infection.
Materials and methods
Peptide synthesis
The synthesis of RTD-1 (>98%) was performed using Fmoc chemistry as previously described.11 Stock solutions of RTD-1 were prepared in 0.01% acetic acid (AcOH) for in vitro assays or in 0.85 M NaCl for administration to animals.
Susceptibility testing
Study strains included a total of 41 strains of mucoid and non-mucoid P. aeruginosa from patients with CF, of which 29 were from patients at the Keck Medical Center of the University of Southern California and 12 were colistin-resistant strains from patients in Denmark.6,7 Agar disc diffusion susceptibility results for the 29 isolates were provided by the Keck Clinical Microbiology Laboratory. The Institutional Review Boards of the University of Southern California and Massachusetts General Hospital approved the use of clinical materials for this study. The colistin-resistant strains have been well characterized with respect to the mechanism of resistance, including phoPQ and/or pmrAB mutations.6,7 Control strains were PAO1 (supplied by Dr Edith Porter, California State University Los Angeles) and RP73 (supplied by Dr Alessandra Bragonzi, San Raffaele Scientific Institute). MICs and MBCs of RTD-1 were determined by broth microdilution assays according to the guidelines of the CLSI (formerly NCCLS) with some modifications as described below. The components of Mueller–Hinton broth (MHB) (e.g. beef extract and casein) have substantially greater concentrations of negatively charged amino acids relative to the protein composition of human tissues.14 Thus, positively charged antimicrobial peptides such as RTD-1 (+5) can be complexed and inactivated by long-chain polyanions present in MHB. In order to provide a more physiological environment to facilitate in vitro investigations of cationic antimicrobial peptide activity, anion-exchange columns have been used to remove these polyanions.14 CAMHB (BBL 212322; Becton, Dickinson and Company, Sparks, MD, USA) was prepared according to the manufacturer's instructions. Anion-depleted MHB (ADMHB) was prepared by pumping 20 mL of CAMHB through a series of three Sep-Pak Plus NH2 anion-exchange cartridges (WAT020535; Waters, Milford, MA, USA) as previously described,14 followed by passage through a 0.22 μm filter (Whatman, Clifton, NJ, USA). Both CAMHB and ADMHB supported luxuriant growth of P. aeruginosa (data not shown). Bacteria were grown overnight at 37°C at 200 rpm in CAMHB and were then diluted with additional CAMHB to a turbidity equivalent to that of a 0.5 McFarland standard as measured by spectrophotometry at 600 nm. Bacteria were further diluted 1 : 200 in either CAMHB or ADMHB and 0.1 mL of a standardized inoculum was dispensed into 96-well plates in triplicate (corresponding to 0.5–1 × 105 cfu/well). Experiments were performed using MASTERBLOCK 96-well deep-well polypropylene microplates (Greiner Bio-One, Frickenhausen, Germany) to reduce potential binding of RTD-1 to the plasticware. RTD-1 concentrations ranging from 0.5 to 256 mg/L at serial 2-fold dilutions were then added to the plates. The antipseudomonal colistin sulphate (Sigma, St Louis, MO, USA) was used as a reference antibiotic. Since most clinical strains tended to grow slower, strains that did not show visible turbidity in the negative control wells were incubated for another 24 h and reinspected. The MBC was determined by placing 10 μL from each well onto Pseudomonas isolation agar (PIA) plates and incubating at 37°C for 24–48 h. The MBC was defined as the lowest concentration at which no visible colonies were observed (≥3 log10 reduction relative to input inoculum).
Time–kill kinetics
The kinetics of RTD-1 microbicidal activity was evaluated by broth macrodilution against PAO1 using ADMHB as described above. Briefly, an inoculum of 1 × 105 cfu/mL was exposed to a range of RTD-1 concentrations (0.25×, 1×, 4×, 16× and 64× MIC) and incubated at 37°C. Aliquots were taken following RTD-1 exposure for 0, 0.25, 0.5, 1, 1.5, 2, 3, 4 and 24 h, then serially diluted and plated onto PIA. The plates were incubated at 37°C and cfu were counted after 24 h. Killing curves were constructed by plotting the log10 cfu/mL versus time.
Post-antibiotic effect (PAE)
The in vitro PAE was determined using PAO1 by standard methods.15 Briefly, PAO1 in logarithmic growth phase was exposed for 15 min in ADMHB to RTD-1 at concentrations of 0.25×, 1×, 4×, 16× and 64× MIC. Unbound RTD-1 was removed by twice centrifuging the suspension at 3000 g for 10 min, decanting the supernatant and resuspending bacteria in pre-warmed broth. Viable counts were performed at 0, 1, 2, 3, 4, 5, 6, 7 and 24 h on PIA. A growth control was performed similarly, without exposure to RTD-1. The colonies were counted after 24 h of incubation at 37°C. PAE was defined as the difference in time required for a 1 log increase in cfu between RTD-1-exposed and control curves.
Biofilm growth and scanning electron microscopy
We investigated the antibacterial effects of RTD-1 on Pseudomonas biofilms using PAO1 in a static colony biofilm assay. Biofilm growth and susceptibility testing was performed as described previously with some modifications.16 Briefly, for biofilm formation, M63 (Amresco, Solon, OH, USA), a nutritionally deficient medium, was prepared according to the manufacturer's instructions and supplemented with 0.5% (w/v) casamino acids after autoclaving. For solid M63 medium, sterile agarose solution (15 g/L) with 0.25% Tween 20 was added to the M63 broth. Biofilms were grown on sterile 25 mm diameter, 0.22 μm pore size polycarbonate membrane filters. For biofilm growth, PAO1 was inoculated from frozen stock into LB broth and grown overnight, then subcultured 1 : 100 into M63 broth and incubated at 37°C for ∼3 h, until OD600 = 0.2. Filters (shiny side up) were gently pressed onto the surface of M63 agarose plates and 10 μL of the culture was dropped onto the centre of each membrane. Membranes were allowed to dry before inverting and incubating at 37°C. After 24 h, filters were aseptically transferred to a fresh plate and incubated for an additional 24 h.
Biofilms were imaged by scanning electron microscopy at the USC Norris Comprehensive Cancer Center Cell and Tissue Imaging Core as previously described.16 Briefly, bacteria were harvested and fixed in half-strength Karnovsky's fixative, post-fixed in 2% OsO4, followed by ethanol dehydration and hexamethyldisilazane drying. Air-dried specimens were mounted on specimen stubs using silver paste and sputter-coated with gold-palladium according to standard procedures. Specimens were visualized by scanning electron microscopy on a JEOL JSM-6390LV instrument (JEOL, MA, USA) operated at 10 kV accelerating voltage.
Biofilm susceptibility
For susceptibility testing, RTD-1 was added to the M63 agarose solution to prepare plates containing 16, 32, 64 or 128 mg/L. After 48 h of growth on M63 agarose alone, biofilms were transferred to the RTD-1-containing plates and incubated for 24 h, then transferred to a fresh RTD-1-containing plate and incubated for an additional 24 h. Biofilms were aseptically removed, placed in 10 mL of sterile PBS and subjected to sonication for 10 min in a Solid State Ultrasonic FS-9 sonicator (Fisher Scientific, Pittsburg, PA, USA) at 4°C for 10 min with an additional 2 min of vortexing, until complete removal of biofilms from the filters was achieved. The solution was then serially diluted and plated onto PIA to obtain cfu counts, calculated as the number of cfu per cm2 of membrane.
Pharmacokinetic study design
All animal studies were conducted with approval of the University of Southern California Institutional Animal Care and Use Committee (protocol #20157). Animals were cared for in accordance with the National Research Council recommendations. Sixteen uninfected C57BL/6N male mice (Charles River, Holister, CA, USA), 8–12 weeks old, were administered RTD-1 (10 mg/mL) in 0.83 M NaCl over 1 h via jet nebulization (6 L/min) using a BANG nebulizer coupled to a 16-port nose-only inhalation exposure system (CH Technologies, Westwood, NJ, USA). The nebulizer produces a relatively small particle size (1 μm) to allow for maximum penetration into the airways and enables simultaneous and uniform aerosol delivery to multiple mice.17 The delivered dose to the mouse was calculated to be 167 μg/kg using standard methods.18 At timed intervals (0.25, 0.5, 1 and 8 h), cohorts of four mice were sacrificed for bronchoalveolar lavage fluid (BALF) sample collection. BALF samples were stored acidified with 5% AcOH at 4°C until analysis. The BALF RTD-1 concentration was determined by UPLC-MS/MS (see below) with correction using the BALF urea concentration as previously described.19
BALF sample preparation and LC-MS/MS quantification
BALF samples from RTD-1-exposed animals and controls (1.5 mL) were acidified with 5% AcOH and spiked with 10 μL of internal standard. After vortexing for 1 min, mixtures were loaded onto C18 SPE (Waters) columns. The columns were hydrated with 100% MeCN and equilibrated with 5% AcOH. After loading, desalting of the sample was performed with 5% MeOH and 1% AcOH in water. Elution was performed with 70% MeCN and 5% AcOH in water. Samples were collected in silicon-coated serum top tubes (Becton, Dickinson and Company, Franklin Lakes, NJ, USA) and vacuum concentrated overnight, washed with 1 mL of water, frozen and lyophilized using a VirTis Freezemobile 25EL lyophilizer (SP Scientific, Warminster, PA, USA). Samples were resuspended in 100 μL of 10% AcOH and 5% MeCN and RTD-1 concentrations were determined by LC-MS using an ACQUITY UPLC system (Waters) connected to a Micromass Quattro Ultima mass spectrometer (Micromass, Manchester, UK). Samples were resolved using a linear gradient of water/acetonitrile/1% formic acid from 85/5/10 to 35/55/10 (v/v/v) over 10 min on a C18 X-Bridge BEH column (2.5 μm, 2.1 × 150 mm XP; Waters) fitted with a VanGuard Pre-Column BEC C18 (1.7 μm; Waters). RTD-1 was analysed in positive ionization mode with declustering potential and collision energy optimized for RTD-1. The interassay precision in BALF ranged from 4.4% to 11.1% for calibration standards. The precision and accuracy in BALF for a quality control sample were 6% and 106%, respectively.
Murine chronic P. aeruginosa lung infection model
The chronic murine model of agar bead-coated P. aeruginosa infection was used to assess the effect of RTD-1 on lung bacterial burden, airway inflammatory cells and weight loss. Chronic infection was established in 8–12-week-old CFTR F508del-homozygous gut-corrected mice [B6.Tg(FABPhCFTR)-CftrtmUth1; Case Western University] by intratracheal instillation of a mucoid strain of P. aeruginosa (RP73) embedded within agarose beads as previously described.20 This model establishes chronic P. aeruginosa airway infection with stable colony counts of 104–105 cfu/lung 7 days following instillation.2 Animal body weight was assessed daily during the study period. Mice were euthanized on day 7 and bronchoalveolar lavage was performed. Total and differential cell counts were determined and lungs were processed for quantitative culture.
Aerosol administration of RTD-1
RTD-1 (10 mg/mL) in 0.83 M NaCl or saline alone was administered daily over 1 h via jet nebulization for 7 days starting 24 h after pulmonary challenge with agar bead-coated bacteria (see above). This RTD-1 dose was selected based on preliminary pharmacokinetic and dose-finding studies in vivo.
Phagocytosis assay
We conducted in vitro experiments designed to evaluate the effect of RTD-1 on phagocytosis as prior studies have shown that defensins reduce bacterial load in mouse models of infection by enhancing macrophage phagocytosis.13,21 THP-1 human monocytic cells were grown in RPMI-1640 medium (Lonza, Walkersville, MD, USA) with 0.05 mM 2-mercaptoethanol (Amresco, Solon, OH, USA), 1% penicillin/streptomycin (Sigma) and 10% FBS (Lonza) at 37°C and 5% CO2. For experiments, THP-1 cells were resuspended in complete cell culture medium with 100 nM phorbol 12-myristate 13-acetate (Sigma) and incubated for 72 h to allow differentiation into macrophages. Cells were washed and replenished with complete cell culture medium for 48 h to return to a resting state. Effects on phagocytosis were measured colorimetrically using the CytoSelect™ assay kit (Cell Biolabs, San Diego, CA, USA) containing pre-labelled zymosan particles according to the manufacturer's instructions as previously described.22,23 Briefly, THP-1 macrophage cells at 5 × 105 cells/mL were pre-incubated for 15 min with RTD-1 over several logs of concentration (0.01–10 mg/L), cytochalasin D (phagocytosis inhibitor) or no treatment. Zymosan particles were added in duplicate and the samples were incubated for 2 h. Samples were washed, fixed with formaldehyde, blocked and permeabilized with multiple wash steps in-between. An enzyme-labelled detection reagent was added followed by colorimetric substrate. Absorbance was measured at 405 nm and the results are presented as the percentage relative to no treatment.
Pharmacokinetic analysis
Pharmacokinetic parameters were derived from the concentration–time profiles using non-compartmental analysis in accordance with statistical moment theory and the linear trapezoidal rule.
Statistical analysis
Data that follow a normal distribution are reported as mean ± SD. Log10-transformed data were found to be near normal by measures of central tendency as well as skewness and kurtosis and are reported as geometric mean ± 95% CI. Comparisons between two groups of normally distributed data were made using the unpaired two-sample Student's t-test or two-way ANOVA. A two-sided P value of ≤0.05 was considered significant. The Bonferroni post hoc test was used when appropriate. All statistics were performed with GraphPad Prism 6.0 (San Diego, CA, USA).
Results
RTD-1 exhibits potent in vitro antibacterial activity against P. aeruginosa isolates from patients with CF
Susceptibility testing was performed on 41 clinical strains of P. aeruginosa. For 29 of the isolates, the phenotype (mucoid or non-mucoid) as well as agar disc diffusion susceptibility results were available from the Keck Clinical Microbiology Laboratory (Table 1). About half (55%) of the isolates were mucoid; 38% were MDR (defined as resistance to agents belonging to two of the three major classes of antipseudomonal antibiotics: meropenem, tobramycin and ciprofloxacin). The antimicrobial activity of RTD-1 against the panel of clinical isolates was ∼16-fold lower using CAMHB compared with ADMHB; MIC50 and MIC90 values were 64 and 128, and 4 and 8 mg/L using CAMHB versus ADMHB, respectively. In contrast, only modest media-specific effects were observed with colistin: MIC50 and MIC90 values were >256 and >256, and 64 and 256 mg/L when using CAMHB versus ADMHB, respectively. RTD-1 exhibited antibacterial activity against clinical strains of P. aeruginosa regardless of mucoid phenotype (MIC90 8 mg/L for mucoid isolates versus 4 mg/L for non-mucoid isolates) or MDR phenotype (MIC90 8 mg/L for MDR isolates versus 8 mg/L for all isolates), demonstrating a lack of cross-resistance to existing classes of antipseudomonal antibiotics. The MBC values of RTD-1 for the clinical strains were on average within 1 tube dilution of the MIC indicating RTD-1 is bactericidal with a mean MBC : MIC ratio of ≤4.24 Additionally, RTD-1 activity was tested in ADMHB against 12 strains of P. aeruginosa that showed high-level colistin resistance caused by mutations in phoPQ and/or pmrAB,6,7 which confer cross-resistance to other cationic antimicrobial peptides25 (Table 2). RTD-1 exhibited excellent antibacterial activity against the colistin-resistant isolates with MIC50 and MIC90 values of 3 and 4 mg/L, respectively, in the same range as those for the colistin-susceptible isolates (MIC50 and MIC90 values of 4 and 8 mg/L, respectively).
. | All (n = 29) . | M/NM (n = 16/13) . | CIP R (n = 13) . | MEM R (n = 13) . | TOB R (n = 5) . | MDR (n = 11) . |
---|---|---|---|---|---|---|
MIC50 (mg/L) | 4 | 4/4 | 4 | 4 | 4 | 4 |
MIC90 (mg/L) | 8 | 8/4 | 6.4 | 8 | 8 | 8 |
MICrange (mg/L) | 2–8 | 2–8/2–4 | 2–8 | 2–8 | 4–8 | 2–8 |
MBC50 (mg/L) | 4 | 4/4 | 4 | 4 | 4 | 4 |
MBC90a (mg/L) | 16 | 30/13 | 42 | 42 | 8 | 53 |
MBCrange (mg/L) | 2–64 | 2–64/2–16 | 2–64 | 2–64 | 4–8 | 2–64 |
MBC/MICb | 1.8 | 1.8/1.8 | 2.1 | 2.1 | 1.1 | 2.2 |
. | All (n = 29) . | M/NM (n = 16/13) . | CIP R (n = 13) . | MEM R (n = 13) . | TOB R (n = 5) . | MDR (n = 11) . |
---|---|---|---|---|---|---|
MIC50 (mg/L) | 4 | 4/4 | 4 | 4 | 4 | 4 |
MIC90 (mg/L) | 8 | 8/4 | 6.4 | 8 | 8 | 8 |
MICrange (mg/L) | 2–8 | 2–8/2–4 | 2–8 | 2–8 | 4–8 | 2–8 |
MBC50 (mg/L) | 4 | 4/4 | 4 | 4 | 4 | 4 |
MBC90a (mg/L) | 16 | 30/13 | 42 | 42 | 8 | 53 |
MBCrange (mg/L) | 2–64 | 2–64/2–16 | 2–64 | 2–64 | 4–8 | 2–64 |
MBC/MICb | 1.8 | 1.8/1.8 | 2.1 | 2.1 | 1.1 | 2.2 |
M, mucoid; NM, non-mucoid; CIP, ciprofloxacin; MEM, meropenem; TOB, tobramycin; R, resistance (determined by agar-based disc diffusion according to CLSI criteria); MIC50 and MIC90, MIC inhibiting 50% and 90% of the strains tested, respectively; MBC50 and MBC90, MBC killing 50% and 90% of the strains tested, respectively.
aOne isolate (mucoid, meropenem resistant and ciprofloxacin resistant) exhibited an MBC of 64 mg/L.
bMedian.
. | All (n = 29) . | M/NM (n = 16/13) . | CIP R (n = 13) . | MEM R (n = 13) . | TOB R (n = 5) . | MDR (n = 11) . |
---|---|---|---|---|---|---|
MIC50 (mg/L) | 4 | 4/4 | 4 | 4 | 4 | 4 |
MIC90 (mg/L) | 8 | 8/4 | 6.4 | 8 | 8 | 8 |
MICrange (mg/L) | 2–8 | 2–8/2–4 | 2–8 | 2–8 | 4–8 | 2–8 |
MBC50 (mg/L) | 4 | 4/4 | 4 | 4 | 4 | 4 |
MBC90a (mg/L) | 16 | 30/13 | 42 | 42 | 8 | 53 |
MBCrange (mg/L) | 2–64 | 2–64/2–16 | 2–64 | 2–64 | 4–8 | 2–64 |
MBC/MICb | 1.8 | 1.8/1.8 | 2.1 | 2.1 | 1.1 | 2.2 |
. | All (n = 29) . | M/NM (n = 16/13) . | CIP R (n = 13) . | MEM R (n = 13) . | TOB R (n = 5) . | MDR (n = 11) . |
---|---|---|---|---|---|---|
MIC50 (mg/L) | 4 | 4/4 | 4 | 4 | 4 | 4 |
MIC90 (mg/L) | 8 | 8/4 | 6.4 | 8 | 8 | 8 |
MICrange (mg/L) | 2–8 | 2–8/2–4 | 2–8 | 2–8 | 4–8 | 2–8 |
MBC50 (mg/L) | 4 | 4/4 | 4 | 4 | 4 | 4 |
MBC90a (mg/L) | 16 | 30/13 | 42 | 42 | 8 | 53 |
MBCrange (mg/L) | 2–64 | 2–64/2–16 | 2–64 | 2–64 | 4–8 | 2–64 |
MBC/MICb | 1.8 | 1.8/1.8 | 2.1 | 2.1 | 1.1 | 2.2 |
M, mucoid; NM, non-mucoid; CIP, ciprofloxacin; MEM, meropenem; TOB, tobramycin; R, resistance (determined by agar-based disc diffusion according to CLSI criteria); MIC50 and MIC90, MIC inhibiting 50% and 90% of the strains tested, respectively; MBC50 and MBC90, MBC killing 50% and 90% of the strains tested, respectively.
aOne isolate (mucoid, meropenem resistant and ciprofloxacin resistant) exhibited an MBC of 64 mg/L.
bMedian.
Activity of RTD-1 against colistin-resistant clinical strains of P. aeruginosa with known resistance mutations in phoPQ and/or pmrAB
Isolate . | Colistin MIC (mg/L) . | RTD-1 . | |
---|---|---|---|
MIC (mg/L) . | MBC (mg/L) . | ||
1019 | 4 | 4 | 4 |
1565 | 8 | 4 | 4 |
1995 | 8 | 2 | 4 |
1033 | 16 | 2 | 4 |
1603 | 32 | 4 | 4 |
1571 | 64 | 1 | 2 |
1581 | 64 | 2 | 4 |
1582 | 64 | 2 | 4 |
1016 | 128 | 4 | 4 |
1018 | 128 | 2 | 4 |
1020 | >256 | 4 | 4 |
1597 | 256 | 4 | 4 |
Isolate . | Colistin MIC (mg/L) . | RTD-1 . | |
---|---|---|---|
MIC (mg/L) . | MBC (mg/L) . | ||
1019 | 4 | 4 | 4 |
1565 | 8 | 4 | 4 |
1995 | 8 | 2 | 4 |
1033 | 16 | 2 | 4 |
1603 | 32 | 4 | 4 |
1571 | 64 | 1 | 2 |
1581 | 64 | 2 | 4 |
1582 | 64 | 2 | 4 |
1016 | 128 | 4 | 4 |
1018 | 128 | 2 | 4 |
1020 | >256 | 4 | 4 |
1597 | 256 | 4 | 4 |
Activity of RTD-1 against colistin-resistant clinical strains of P. aeruginosa with known resistance mutations in phoPQ and/or pmrAB
Isolate . | Colistin MIC (mg/L) . | RTD-1 . | |
---|---|---|---|
MIC (mg/L) . | MBC (mg/L) . | ||
1019 | 4 | 4 | 4 |
1565 | 8 | 4 | 4 |
1995 | 8 | 2 | 4 |
1033 | 16 | 2 | 4 |
1603 | 32 | 4 | 4 |
1571 | 64 | 1 | 2 |
1581 | 64 | 2 | 4 |
1582 | 64 | 2 | 4 |
1016 | 128 | 4 | 4 |
1018 | 128 | 2 | 4 |
1020 | >256 | 4 | 4 |
1597 | 256 | 4 | 4 |
Isolate . | Colistin MIC (mg/L) . | RTD-1 . | |
---|---|---|---|
MIC (mg/L) . | MBC (mg/L) . | ||
1019 | 4 | 4 | 4 |
1565 | 8 | 4 | 4 |
1995 | 8 | 2 | 4 |
1033 | 16 | 2 | 4 |
1603 | 32 | 4 | 4 |
1571 | 64 | 1 | 2 |
1581 | 64 | 2 | 4 |
1582 | 64 | 2 | 4 |
1016 | 128 | 4 | 4 |
1018 | 128 | 2 | 4 |
1020 | >256 | 4 | 4 |
1597 | 256 | 4 | 4 |
RTD-1 exhibits rapid, concentration-dependent bactericidal activity against PAO1
Based on the observation that RTD-1 exhibits bactericidal activity, we sought to further characterize its pharmacodynamic properties by performing time–kill and PAE experiments. Results of the time–kill studies indicated very rapid, concentration-dependent bactericidal activity of RTD-1 against PAO1 (Figure 1). Greater than or equal to a 3 log10 cfu/mL reduction was achieved with RTD-1 at concentrations ≥4× MIC (MIC = 2 mg/L). No appreciable PAE was observed, since bacterial regrowth ensued within 60 min following short exposures to all concentrations tested (data not shown).
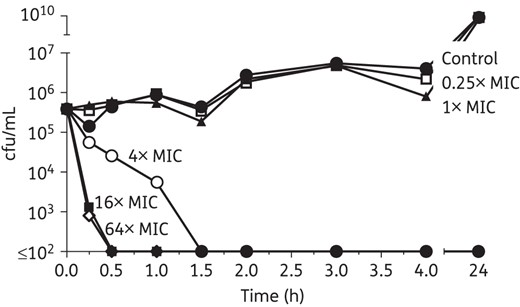
Concentration-dependent killing of RTD-1 against PAO1. After overnight growth in CAMHB, the bacterial suspension (PAO1 RTD-1 MIC = 2 mg/L) was diluted 1 : 200 in ADMHB and incubated with RTD-1 at various concentrations. Aliquots were taken at specified times, serially diluted and incubated for 24 h at 37°C for cfu counting.
Activity of RTD-1 against P. aeruginosa biofilms
P. aeruginosa is known to adapt to the lung environment in patients with CF and resist host defences as well as the action of antibiotics through the formation of biofilm aggregates.4 Several cationic peptides have demonstrated activity against P. aeruginosa biofilms when tested at concentrations exceeding the MIC for planktonic bacteria.26 We therefore tested the activity of RTD-1 against PAO1 biofilms using a colony biofilm assay. Aggregates of P. aeruginosa on polycarbonate membranes were visible by scanning electron microscopy (Figure 2). Treatment of established biofilms with RTD-1 demonstrated a modest, but statistically significant, reduction in cfu when compared with the negative control at all concentrations tested (Figure 2). RTD-1 exerted maximum effect at 32 mg/L, decreasing biofilm abundance by an average of 74.2% compared with the control. However, there was no increase in cfu with increasing concentrations (64 and 128 mg/L, respectively).
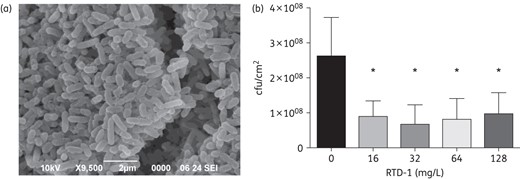
Activity of RTD-1 against PAO1 biofilms. Biofilms were grown on polycarbonate membranes placed on M63 agarose plates as described in the Materials and methods section. (a) Electron microscopy image of PAO1 aggregates on polycarbonate membranes. After 48 h of growth, the membranes were transferred to fresh agarose plates containing RTD-1. (b) Activity of RTD-1 against PAO1 biofilms. After exposure to RTD-1 at various concentrations (8–64× MIC) for 48 h, biofilms on the membranes were resuspended in sterile PBS, diluted and plated to determine cfu. Values represent an average of three independent experiments ± SEM. *P < 0.05 for comparison of each concentration of RTD-1 with the control.
Pharmacokinetics of aerosolized RTD-1
The epithelial lung fluid (ELF) concentration–time plot is shown in Figure 3. The area under the ELF concentration–time curve of RTD-1 over 8 h (AUC0–8) was 200.9 ± 16.1 mg/L × h, with a maximum concentration (Cmax) in ELF of 50.64 ± 10.49 mg/L at 30 min post-nebulization. The AUC0–8 : MIC was 50.2 ± 4.0 and the peak ELF concentration to MIC was 12.7 ± 2.6. The half-life of RTD-1 was 3.2 ± 0.7 h in the airways with the time above the MIC estimated to be 11.8 ± 3.1 h.
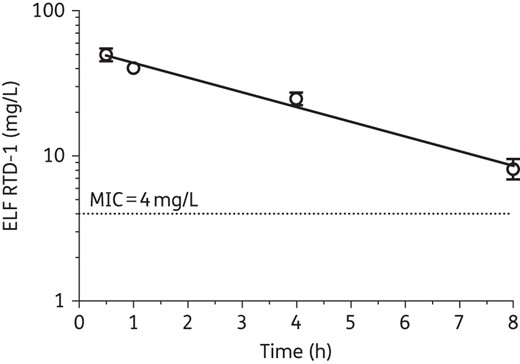
RTD-1 concentrations in ELF versus time after a single aerosolized dose of RTD-1 in non-infected C57BL/6N mice. Data represent mean ± SEM from mice (n = 4 for each timepoint) with a linear regression line. The broken line indicates the in vitro MIC for the P. aeruginosa isolate (RP73) used in the chronically infected mice.
Aerosolized RTD-1 is efficacious in a murine model of chronic P. aeruginosa lung infection
The agar bead model of chronic P. aeruginosa airway infection has been used for evaluating the activity of antibacterial agents for potential application in the treatment of CF lung disease.2,3 This model was predictive of the therapeutic failure of a leukotriene B4 antagonist in patients with CF and has been recommended as a pre-clinical screening tool for potential anti-inflammatory therapies in CF.27 A comparator group of 10 CFTR F508del-homozygous mice was exposed to aerosolized saline. A significant reduction in log10 cfu of P. aeruginosa was observed when compared with the control at the end of a 7 day treatment (mean difference of −1.30, P = 0.0061; 95% CI: −2.18 to −0.42) (Figure 4a). These results are consistent with the in vitro activity of RTD-1 in ADMHB against the infecting strain RP73 (RTD-1 MIC = 4 mg/L). RTD-1 treatment also produced a significant reduction in airway leucocytes (mean log10 difference of −0.37 cells, P = 0.0012; 95% CI: −0.57 to −0.16) (Figure 4b) as well as significantly less weight loss at days 6 (mean difference of −12.25%; P < 0.05) and 7 (mean difference of −12.62%; P < 0.05) (Figure 4c). Mortality was not observed in this chronic infection model.
![Aerosolized RTD-1 significantly reduced lung cfu in a murine model of chronic P. aeruginosa lung infection. Total lung cfu were counted 7 days after intratracheal inoculation with P. aeruginosa (RP73 MIC = 4 mg/L) in CFTR F508del-homozygous mice. Nebulized treatment with a delivered dose of 167 μg/kg RTD-1 or saline began 24 h post-inoculation. Data were pooled from two independent experiments (saline, n = 10; and ΔF508, n = 12). Treatment with aerosolized RTD-1 was associated with a significant reduction in (a) log10 cfu of P. aeruginosa [mean difference of −1.30 (95% CI: −2.18 to −0.42); **P = 0.0061], (b) log10 airway leucocytes [mean difference of −0.37 (95% CI: −0.57 to −0.16); **P = 0.0012], data represent mean ± SEM, and (c) weight loss at days 6 (mean difference of −12.25%; *P < 0.05) and 7 (mean difference of −12.62%; *P < 0.05), data represent mean ± SD, when compared with the control. Independent two-sample t-test with α = 0.05.](https://oup.silverchair-cdn.com/oup/backfile/Content_public/Journal/jac/71/1/10.1093_jac_dkv301/2/m_dkv30104.jpeg?Expires=1750495919&Signature=wWrv2692Vgfn6elB4N1SqYaREdNkDlDLf1BIkeFTHmayylj7cnVmCPbU5HQrqbMdXXtE936ACMt2SPhZSAQwUy-Yxt~8aYE8zxiLTZFwtQGqjVfotSyPMPRxpeR83lPpEXApGyAX6neruS3d1fPPIeTNpnPHaljpKstLIm8RZJdCTBVVu8pQwOcYbl3Nc3Y5l5mxCVe-QC5yA3vmlbyNfss-RtwMpAI~vjCgxMLf5MGxXuz3ScsosLKLK8Ve4Q7RCnQxjhqBT3OhPUgPmat~HsPL1IffJV9xozDAW5W8XuAW7TJfi~fkfuGcwV8FRimTbg7GZS686WLwIGo0bFjBwg__&Key-Pair-Id=APKAIE5G5CRDK6RD3PGA)
Aerosolized RTD-1 significantly reduced lung cfu in a murine model of chronic P. aeruginosa lung infection. Total lung cfu were counted 7 days after intratracheal inoculation with P. aeruginosa (RP73 MIC = 4 mg/L) in CFTR F508del-homozygous mice. Nebulized treatment with a delivered dose of 167 μg/kg RTD-1 or saline began 24 h post-inoculation. Data were pooled from two independent experiments (saline, n = 10; and ΔF508, n = 12). Treatment with aerosolized RTD-1 was associated with a significant reduction in (a) log10 cfu of P. aeruginosa [mean difference of −1.30 (95% CI: −2.18 to −0.42); **P = 0.0061], (b) log10 airway leucocytes [mean difference of −0.37 (95% CI: −0.57 to −0.16); **P = 0.0012], data represent mean ± SEM, and (c) weight loss at days 6 (mean difference of −12.25%; *P < 0.05) and 7 (mean difference of −12.62%; *P < 0.05), data represent mean ± SD, when compared with the control. Independent two-sample t-test with α = 0.05.
Phagocytosis
Cationic peptides have been reported to enhance phagocytosis of bacteria in vivo. Therefore, we conducted experiments designed to determine whether RTD-1 has an effect on phagocytosis, which could account for the reduction in lung bacterial counts in the murine model of chronic P. aeruginosa infection. Using an in vitro model with zymosan particles, we found that treatment of THP-1 macrophages with RTD-1 did not have any appreciable effect on phagocytosis, which suggests a direct bactericidal activity of RTD-1 (Figure 5).
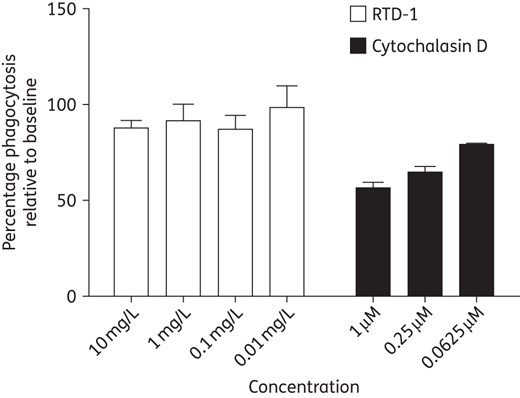
Phagocytosis of zymosan particles by THP-1 macrophages. THP-1 macrophage cells at 5 × 105 cells/mL were pre-incubated with RTD-1 (10–0.01 mg/L), the known phagocytosis inhibitor cytochalasin D or no treatment for 15 min. Zymosan particles were added in duplicate and the samples were incubated for 2 h. An enzyme-labelled detection reagent was added followed by colorimetric substrate. Absorbance was measured at 405 nm and the results are presented as the percentage relative to baseline.
Discussion
The relatively high prevalence of antibiotic resistance in P. aeruginosa and adaptations, including the propensity to form biofilm aggregates in CF lung disease, highlights the critical need for novel therapies to combat this pathogen. θ-Defensins are cationic macrocyclic antimicrobial peptides isolated from leucocytes of Old World monkeys including rhesus macaques and olive baboons.28 The peptides are not expressed in humans, great apes or other hominids. Six rhesus macaque θ-defensin isoforms have been identified, with RTD-1 being the most abundant, accounting for nearly 50% of the RTD content in polymorphonuclear neutrophils.10 The cyclic backbone structure of θ-defensins is unique among peptides produced in the animal kingdom and provides remarkable stability (e.g. heat and low pH) and increased microbicidal activity when compared with their acyclic versions.13,28 Importantly, the cyclic structure confers stability to proteases (P. M. Beringer and T. J. Bensman, unpublished data), which is relevant since the airways of patients with CF are known to contain high concentrations of serine and metalloproteases.
RTD-1 exhibits potent broad-spectrum microbicidal activity against Escherichia coli, Staphylococcus aureus and Candida albicans.10,11 Of particular relevance to CF is the potent activity of RTD-1 against clinical isolates of MRSA and fluoroquinolone-resistant P. aeruginosa.12 The mechanism of the antibacterial effect of RTD-1 was investigated in studies on S. aureus and E. coli and is attributed to peptide-induced permeabilization/impairment of the cytoplasmic membrane of both organisms.11,29
In this study, we evaluated the antibacterial activity of RTD-1 against CF clinical isolates of P. aeruginosa, including isolates with mucoid, MDR and colistin-resistant phenotypes. RTD-1 was bactericidal against a panel of antibiotic-resistant clinical isolates including several strains with phoPQ and/or pmrAB mutations that confer high-level resistance to colistin. In vitro activity was present only when tested in ADMHB, consistent with other studies that have evaluated the antibacterial activities of cationic antimicrobial peptides.14,30 ADMHB, described by Turner et al.14 and Yu et al.,31 eliminates RTD-1 binding polyanions from CAMHB, but still allows for luxuriant bacterial growth. Importantly, the antipseudomonal activities of RTD-1 in vitro in ADMHB correlated with efficacy of the peptide in vivo.
The low MBC/MIC ratio and rapid bactericidal activity in the time–kill experiments demonstrate that RTD-1 exhibits highly potent antipseudomonal activity. These properties are characteristic of several cationic antimicrobial peptides in vitro, which exert their antimicrobial activity by disrupting the bacterial cell membrane and gaining access to critical intracellular functions. In particular, the rapid concentration-dependent bactericidal activity observed with RTD-1 very closely mirrors that of colistin with nearly complete bacterial killing within 90 min at RTD-1 concentrations as low as 4× MIC.15
RTD-1 did not demonstrate any appreciable PAE in vitro. This contrasts with the aminoglycosides and colistin, which exhibit in vitro PAEs.15,32 Knowledge of these pharmacodynamic characteristics suggests that dosing of RTD-1 should be optimized by maximizing the peak concentration while minimizing the length of time that concentrations are below the MIC. Ongoing pharmacokinetic studies will help define the optimal dose and frequency of administration to maximize antibacterial activity.
The antibacterial activity of RTD-1 was compared with colistin, a cationic peptide currently in clinical use for treatment of infections involving MDR P. aeruginosa in CF. Colistin exerts its antibacterial activity through binding to LPS, the major constituent of the Gram-negative outer membrane, resulting in membrane permeabilization. Intracellularly, colistin disrupts cellular respiration resulting in cell lysis and death. Mutations in phoPQ and pmrAB confer high-level resistance to colistin as a result of modifications to the phosphate groups within the lipid A and core oligosaccharide moieties of LPS, leading to reduced binding.6,7 Due to widespread use of chronic inhaled colistin in patients with CF, it is not surprising that resistance has emerged to this agent.6,7 RTD-1 retained excellent activity against CF clinical isolates that are colistin-resistant phoPQ and/or pmrAB mutants as well as those with an MDR phenotype, indicating no cross-resistance to existing antipseudomonal therapies. The MICs for all isolates were between 2 and 8 mg/L with no outliers indicative of resistance. Consistent with these findings, RTD-1 was shown to have little affinity to LPS, especially compared with polymyxin B, an analogue of colistin (polymyxin E).13
To further assess the therapeutic potential of RTD-1 for CF, we determined its activity against P. aeruginosa biofilms. Chronic infections within the CF airway occur in part due to bacterial adaptations, specifically the formation of biofilm aggregates. The presence of biofilms is significant due to their resistance to antibiotics and host defences.33 Several cationic peptides have demonstrated activity against P. aeruginosa biofilms in vitro.26,34–36 Using a static colony biofilm assay, we found that RTD-1 exhibits moderate activity against P. aeruginosa biofilms. Significant activity was demonstrated starting at concentrations of 8× MIC; however, higher concentrations did not result in greater killing. The basis for this plateau phenomenon is not known, but potentially reflects phenotypic variability among organisms within the biofilm. Studies are underway to delineate the mechanistic basis for the observed plateau in the dose–response against biofilm bacteria.
We evaluated the efficacy of RTD-1 in vivo using a CFTR F508del-homozygous murine model of chronic P. aeruginosa lung infection. RTD-1 was administered via nebulization to maximize concentrations within the airways. Seven day treatment of infected mice with nebulized RTD-1 significantly reduced lung infection burden, the number of airway neutrophils and weight loss when compared with the saline control. Pharmacokinetic studies demonstrated achievement of therapeutic concentrations in the airways for ∼12 h. RTD-1 administered via aerosol represents an attractive therapeutic option for CF patients due to the potential of achieving high local lung concentrations.
The results of the phagocytosis assay indicate that RTD-1 has no effect on phagocytosis. These data suggest the killing activity of RTD-1 against P. aeruginosa in vitro and in vivo is a direct antibacterial effect.
In summary, this study demonstrates that RTD-1 possesses rapid and potent in vitro bactericidal activity against P. aeruginosa CF isolates and that microbicidal activity spans mucoid, non-mucoid and MDR phenotypes. Of note, cross-resistance was not observed with RTD-1 and colistin, a cationic peptide antipseudomonal agent. The excellent in vitro activity of RTD-1 was confirmed in a murine model in which nebulized treatment with RTD-1 significantly reduced lung P. aeruginosa burden, while also reducing neutrophilic infiltration and animal weight loss. These data suggest that RTD-1 or its analogues may present a novel therapeutic approach for treatment of CF airway disease.
Funding
Research was supported in part by research grants from the Cystic Fibrosis Foundation (BERING14GO) and Cystic Fibrosis Research Inc. (P. M. B.), the National Institute of Dental and Craniofacial Research (5T90DE021982) and the National Institute of General Medicine (F31GM109729) (T. J. B.), RO1AI22931 and R01DE 021341 (M. E. S.) and the National Cancer Institute (P30CA014089).
Transparency declarations
M. E. S. has an equity interest in Oryn Therapeutics, LLC, an entity that seeks to commercialize θ-defensins as therapeutics. All other authors: none to declare.
Disclaimer
The content is solely the responsibility of the authors and does not necessarily represent the official views of the National Institute of Dental and Craniofacial Research, the National Cancer Institute or the National Institutes of Health.
Acknowledgements
Presented in part at the Thirty-seventh European CF Conference, Gothenburg, Sweden, 2014 (Abstract WS7.2).
References
- antibiotics
- pseudomonas aeruginosa
- lung
- weight reduction
- colistin
- cystic fibrosis
- agar
- biofilms
- cystic fibrosis transmembrane conductance regulator
- defensins
- homozygote
- leukocytes
- macaca mulatta
- peptides
- pseudomonas
- infections
- mice
- lung infections
- anti-bacterial agents
- airway device
- postantibiotic effect
- f508del-cftr