-
PDF
- Split View
-
Views
-
Cite
Cite
Monica Bortolin, Elena De Vecchi, Carlo Luca Romanò, Marco Toscano, Roberto Mattina, Lorenzo Drago, Antibiofilm agents against MDR bacterial strains: is bioactive glass BAG-S53P4 also effective?, Journal of Antimicrobial Chemotherapy, Volume 71, Issue 1, January 2016, Pages 123–127, https://doi.org/10.1093/jac/dkv327
- Share Icon Share
Abstract
The treatment of bone and joint infections is challenging due to the presence of bacterial biofilm and the increasing emergence of multiresistant strains. BAG-S53P4 is a bone substitute that is characterized by osteoconductive and antimicrobial properties. The aim of this study was to assess the effectiveness of BAG-S53P4 against biofilm produced in vitro by multiresistant bacterial strains.
Multiresistant Staphylococcus epidermidis, Acinetobacter baumannii and Klebsiella pneumoniae isolated from bone and joint infections were used in this study. Titanium discs covered by bacterial biofilm were incubated with BAG-S53P4 or inert glass as a control. The amount of biofilm on each titanium disc was evaluated after 48 h of incubation by means of confocal laser scanning microscopy.
Significantly lower total biomass volumes were observed for all strains after treatment with BAG-S53P4 when compared with controls. Moreover, the percentage of dead cells was significantly higher in treated samples than in controls for all the tested strains.
BAG-S53P4 is able to reduce the biofilm produced by multiresistant S. epidermidis, A. baumannii and K. pneumoniae on titanium substrates in vitro, probably by interfering with cell viability. Owing to its osteoconductive, antibacterial and antibiofilm properties, the use of BAG-S53P4 may be a successful strategy for the treatment of bone and prosthetic joint infections.
Introduction
The treatment of prosthetic joint infections (PJIs) is a challenging and expensive procedure, often requiring bone grafting to reconstruct bone defects and prolonged cycles of antibiotic therapy to fight infection. PJIs are often complicated by the presence of biofilm, which protects bacteria from the host immune system and antibacterial agents. Biofilm-related infections are more difficult to treat as they are characterized by chronicity, frequent recurrence, antibiotic resistance, difficult diagnosis, complex and prolonged treatment, poor prognosis and high socioeconomic costs.
Bioactive glass BAG-S53P4 (BonAlive® Biomaterials, Turku, Finland) is a bone substitute characterized by good osteoconductive and mechanical properties,1,2 which has been successfully used in the treatment of osteomyelitis and bone fractures.3–9 In addition, it possesses proangiogenic and antimicrobial properties.6,10–12 The antimicrobial activity of bioactive glass is due to the release of ions from its surface after immersion in body fluids, resulting in an increase of osmotic pressure and pH, which makes the surrounding environment unsuitable for microbial growth.13,14
While many studies evaluated the osteoconductive and antimicrobial properties of BAG-S53P4, only two investigated its antibiofilm activity, both showing promising results.15,16
The aim of this study was to get further insight into the antibiofilm activity of BAG-S53P4, evaluating its effectiveness against the biofilm produced in vitro by MDR bacterial strains isolated from PJIs.
Materials and methods
Bacterial strains
Three multiresistant strains isolated from PJIs at the microbiology laboratory of IRCCS Galeazzi Orthopaedic Institute were used. In particular, the following were used: one strain of Klebsiella pneumoniae producing ESBLs and carbapenemases; one strain of Acinetobacter baumannii resistant to aminoglycosides, fluoroquinolones, cephalosporins and β-lactams and susceptible to colistin; and one strain of methicillin- and glycopeptide-resistant Staphylococcus epidermidis. All strains were screened before the beginning of the study in order to assess their ability to produce biofilm according to the spectrophotometric assay described by Christensen et al.17
BAG-S53P4 preparation and conditioning
BAG-S53P4 (granules of 0.5–0.8 mm diameter) was prepared at a final concentration of 400 mg/mL in tryptic soy broth (TSB; bioMérieux, Marcy-l'Étoile, France). Conditioning was obtained through the incubation of bioactive glass in TSB at 37°C for 48 h. pH values were measured at regular intervals. A pH value ≥10 was considered suggestive of optimal conditioning. Once optimal conditioning was reached, the bioactive glass was ready to use.
Biofilm formation
Sterile sandblasted titanium discs with a diameter of 25 mm and thickness of 5 mm (Adler Ortho, Cormano, Italy) were used as substrate for biofilm formation. Six discs were used for each bacterial strain (3 for inert glass and 3 for BAG-S53P4), for a total of 18 discs. Briefly, bacterial overnight cultures were suspended in TSB at a final density of 1.0 × 108 cfu/mL and 200 μL of each microbial suspension was inoculated into sterile 6-well polystyrene plates (Jet Biofil, Guangzhou, China) containing titanium discs and 4.8 mL of TSB. After incubation at 37°C in aerobic atmosphere for 24 h, the growth medium was removed together with non-adherent bacteria and 5 mL of new broth was added. Plates were incubated for an additional 48 h, until a visible biofilm was obtained.
BAG-S53P4 treatment
Titanium discs covered by bacterial biofilm were placed into new sterile 6-well polystyrene microplates containing the conditioned bioactive glass. Negative controls were performed by adding inert glass of the same granule size (R1350 Iittala clear; Iittala, Finland), which has been shown not to have antibacterial or antibiofilm properties.6,15,16 The amount of biofilm on each titanium disc was evaluated after 48 h of incubation.
Confocal laser scanning microscopy (CLSM) analysis
CLSM analysis was carried out following a method we previously developed for quantitative analysis of biofilm.16,18 Microbial biofilm was stained with a fluorescent stain (Filmtracer™ LIVE/DEAD® Biofilm Viability Kit; Life Technologies, Carlsbad, CA, USA) containing a mixture of two dyes: SYTO® 9 (green stain) and propidium iodide (red stain), 3 μL of each component in 1 mL of saline solution. SYTO® 9 generally labels all bacteria in a population, while propidium iodide penetrates only bacteria with damaged membranes. Thus, bacteria with intact cell membranes (i.e. live) stain fluorescent green, whereas bacteria with damaged membranes (i.e. dead) stain fluorescent red. Discs were incubated with fluorescent stains at room temperature for 15 min in the dark. In order to minimize the air contact and maintain constant sample moisture conditions, a coverslip was placed on the specimen. Stained biofilm was examined with a confocal microscope (Leica model TCS SP5; Leica Microsystems, Mannheim, Germany) using a ×20 dry objective (HC PL FLUOTAR 20×/0.50 dry) plus a ×2 electronic zoom. A 488 nm laser line was used to excite SYTO® 9, while the fluorescent emission was detected at 500–540 nm. Propidium iodide was excited with a 561 nm laser line, while its fluorescent emission was detected at 600–695 nm. In order to avoid false colocalization between the fluorescent stains due to bacterial movement, a simultaneous acquisition mode of the two channels was adopted, in which the laser beam scans the visual field at a frequency of 700 Hz. Moreover, using a third laser line (633 nm) in reflection mode, it was possible to determine both titanium disc (starting acquisition point) and coverslip (ending acquisition point) reflecting surfaces. Images from five randomly selected fields were acquired for each titanium disc. Sequential optical sections of 2 μm were collected along the z-axis over the complete thickness of the biofilm. The resulting stacks of images were quantified using an image processing package (Fijy, ImageJ 1.47 q; National Institute of Health, USA) and subsequently rendered into three-dimensional mode using image analysis software (Imaris 7.2.3, Bitplane, Zurich, Switzerland).
Statistical analysis
Results are expressed as mean ± SD. Comparisons were performed using an unpaired Student's t-test. A value of P ≤ 0.05 was used as the significance level.
Results
Three-dimensional reconstructions of the images of controls and BAG-S53P4-treated samples acquired with CLSM are shown in Figure 1. The corresponding quantifications obtained through the image analysis software are displayed in Figures 2 and 3.
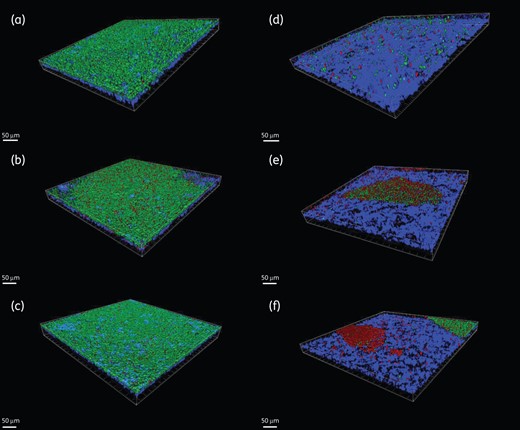
Three-dimensional reconstruction of microbial biofilms. The left-hand panels (a, b and c) represent control samples, while the right-hand panels (d, e and f) represent BAG-S53P4-treated samples of S. epidermidis (a and d), A. baumannii (b and e) and K. pneumoniae (c and f). Green = viable cells. Red = dead cells. Blue = titanium. Scale bar = 50 µm.
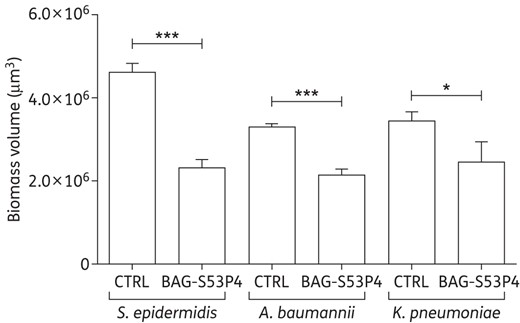
Total biomass volume, expressed in µm3, of the biofilm developed by MDR strains after incubation with 400 mg/mL BAG-S53P4 or inert glass (CTRL) for 48 h. Data are expressed as mean ± SD. ***P ≤ 0.001. *P ≤ 0.05.
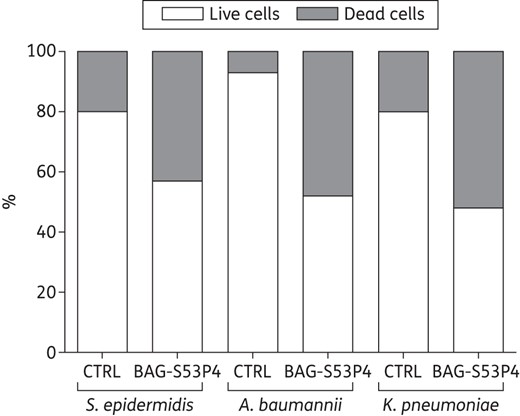
Percentage composition, in terms of live cells (white bars) and dead cells (grey bars), of the biofilm developed by MDR strains after incubation with 400 mg/mL BAG-S53P4 or inert glass (CTRL) for 48 h.
The total biomass volume was estimated for each disc (Figure 2). After incubation with BAG-S53P4, the total biofilm biomass volume was significantly lower than controls for all MDR strains tested. In particular, reductions of 50%, 35% and 26% versus controls were observed for S. epidermidis (P = 0.0001), A. baumannii (P = 0.0002) and K. pneumoniae (P = 0.03), respectively.
The volume of biofilm occupied by bacterial cells was also quantified, together with the percentage of live and dead cells (Figure 3). For all bacteria, a significantly higher percentage of dead cells was observed in treated samples (P = 0.0001 for S. epidermidis, P = 0.0002 for A. baumannii and P = 0.03 for K. pneumoniae versus controls).
Discussion
Over 65% of all human infections are estimated to be biofilm related,19,20 representing a significant burden on the healthcare system. Biofilm-related infections are very common in orthopaedic practice, since the surfaces of commonly used materials for the manufacturing of orthopaedic components (e.g. titanium and its alloys, stainless steel, cobalt-chromium, various polymeric biomaterials and polymethylmethacrylate cement) are susceptible to colonization by biofilm-forming bacteria.21 Eradication of biofilm-related infections is of concern because, thanks to their adapted ability to survive in adverse environmental conditions, bacterial biofilms are recalcitrant to antibiotic therapies and immune clearance.22 The main therapeutic strategy is the use of antibiotics, which, due to adaptive resistance, are often insufficient to clear biofilm infections. Thus, novel antibiofilm therapies are required.
The aim of this study was to evaluate the in vitro antibiofilm activity of BAG-S53P4 against MDR strains isolated from PJIs. The evaluation was carried out by means of CLSM analysis, adopting a well-established method developed by our research group.16,18
Advances in confocal microscopy allowed replacing old semi-quantitative colorimetric methods (e.g. crystal violet assay, developed by Christensen et al.17 in 1985) for biofilm evaluation with a technology characterized by higher sensitivity and specificity. While colorimetric methods only allow the measurement of the dry biomass, CLSM analysis supplies more detailed information as it permits the three-dimensional visualization of living biofilms.
In order to mimic the materials used in orthopaedics, sandblasted titanium discs were used as substrate for the development of biofilm. Granule size, the concentration of BAG-S53P4 and the incubation period were chosen on the basis of previous experiments.8,16
Before use, we activated ion release from bioactive glass through a conditioning step of 48 h. Such a long period was due to the experimental conditions used (e.g. restricted surface of contact between the growth medium and the bioactive glass and the buffering ability of the growth medium), which might slow down ion release in comparison with the in vivo situation. For this reason, in order to achieve optimal efficacy in vitro, BAG-S53P4 needs an activation step that is not required in vivo.
When compared with controls, significantly lower total biomass volumes were observed for all strains after treatment with BAG-S53P4, with percentage reductions ranging from 26% to 50% depending on the strain under evaluation. Similar values were observed for Pseudomonas aeruginosa (36%) and Staphylococcus aureus (47%) strains in a previous study performed under the same experimental conditions.16 However, in previous studies, only SYTO® 9 was used for staining of biofilm for CLSM analysis, so that a distinction could not be made between live and dead cells.
The rate of dead cells was significantly higher in treated samples than in controls for all the tested strains, suggesting that a possible mechanism of action by which BAG-S53P4 is able to reduce the biofilm developed by MDR bacterial strains is interference with cell viability. This observation is supported by previous experiments showing a bactericidal activity of BAG-S53P4 against the same strains tested in this study and against other bacterial strains.6,23
In addition to bacterial cells, biofilm is composed of extracellular polymeric substances (EPS) made of various substances such as polysaccharides, proteins, nucleic acids and lipids. The EPS constitutes an envelope embedding bacterial cells and almost certainly has essential roles in defining the cohesiveness and other physical properties of biofilm.24 A limitation of this study is that a quantitative evaluation of the EPS was not performed, as the LIVE/DEAD kit only stains bacterial cells. Further studies might be performed with stains specific for EPS components, in order to assess the effect of BAG-S53P4 on the biofilm matrix.
As mentioned above, MDR strains were used in this study. At present, the correlation between biofilm production and multidrug resistance is unclear. Strains capable of forming biofilm might be selected under antibiotic pressure or, conversely, they might acquire resistance to multiple drugs within biofilm communities. In either event, the high colonizing capacity of biofilm-forming strains combined with their resistance to multiple drugs will contribute to microorganism survival and further dissemination.25 In this setting, the use of BAG-S53P4, which is able to reduce biofilm formation while bypassing drug resistance, may be the best strategy.
In conclusion, the results of this study show that BAG-S53P4 is able to reduce the biofilm produced by multiresistant S. epidermidis, A. baumannii and K. pneumoniae on titanium substrates in vitro, probably by interfering with bacterial cell viability. Thanks to the combination of its osteoconductive, antibacterial and antibiofilm properties, BAG-S53P4 may be successfully used as a bone substitute for the treatment of bone and PJIs. Further studies will be designed to investigate the ability of BAG-S53P4 to inhibit bacterial adhesion, so as to prevent biofilm formation on prosthetic surfaces.
Funding
This work was supported by the European Commission within the 7th Framework Program (Project I.D.A.C.—Grant Agreement HEALTH-2011-277988).
Transparency declarations
None to declare.