-
PDF
- Split View
-
Views
-
Cite
Cite
Lan Liu, Yongyan Wu, Wenshan Duan, Hongbo Song, Fang Geng, Jinqiu Wang, Qun Huang, Shaofeng Wei, Mechanism of heat-induced thinning of thick egg white in weak alkaline condition: molecular aggregation, functional and structure properties analysis, International Journal of Food Science and Technology, Volume 58, Issue 10, October 2023, Pages e26–e36, https://doi.org/10.1111/ijfs.16273
- Share Icon Share
Abstract
The thinning of thick egg white (KEW) was simulated by weak alkaline (pH 10.0) combined with mild heat (50 °C) treatment. The molecular aggregation, functional and structural characteristics changes in simulated KEW thinning process were comprehensively studied. It was found that KEW molecule entered “molten pellet (MG)” state with decline of apparent viscosity of KEW. At this time, particle size increased by 40.9%, surface hydrophobicity and solubility declined 11.3% and 8.9%, respectively. However, with more mild heating time, KEW structure gradually unfolded, ovomucin (OVM) degraded, particle size diminished significantly. Surface hydrophobicity and solubility increased 6.6% and 19.7%, respectively. Functional properties indicated that thermal gel of KEW gradually became soft and tough, water holding capacity (WHC) advanced by 10%, emulsifying property significantly improved by 40%. Moreover, correlation analysis indicated that tertiary structure was correlated with particle size (P < 0.01), secondary structure related to hardness and WHC (P < 0.05). Through the results of SDS-PAGE and comparison with KEW simple mild heating, it was concluded that rise of pH was intrinsic driving force of KEW thinning. Therefore, this study provided a new insight for revealing the thinning mechanism of KEW and a scientific basis for its industrial application.
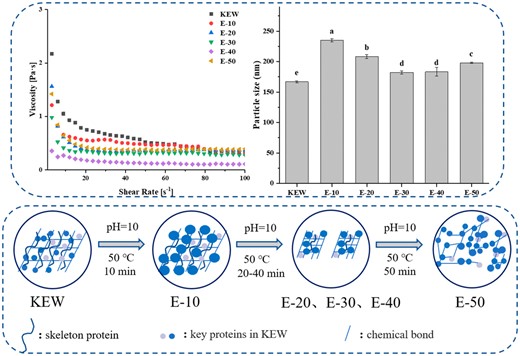
Introduction
As a kind of protein with low price and high nutritional value, egg white protein also has excellent foaming, emulsifying and gel properties, so it has been widely used in the food industry. While eggs would naturally thin after long-term storage, mainly manifested in the thinning of thick egg white (KEW), rise of pH value and drop of moisture content. Huang et al. (2022a) conducted a comparative study on differences in functional and molecular structure characteristics of thick and thin egg white, and concluded that KEW played a dominant role in the thinning of egg white. Wang et al. (2022) further proposed that ovomucin (OVM) as a skeleton protein could maintain the gel network structure of KEW. The results of quantitative proteomics identification indicated that the decline of β-OVM abundance might be directly related to egg white thinning, and the change of OVM abundance possibly influenced the interaction with lysozyme. Nevertheless, variations of these proteins were results of egg white thinning, and the intrinsic driving force of egg white thinning has not been fully analysed. Therefore, it is of great significance to focus on KEW and simulate its thinning process by weak alkaline combined with mild heat treatment.
During simulated thinning of KEW, egg white proteins form a “molten pellet (MG)” state (Alaei et al., 2021). Studies have shown that when globular proteins are treated with appropriate acids, bases, heat treatments or mild denaturants, a MG state between unfolding and folding can be formed. The state has a secondary structure similar to natural state and a tertiary structure determined by the side chain. The protein structure is more compact, rotation radius is 10–30% larger than natural state (Alaei et al., 2021). Since the functional properties of proteins were closely bound up with their specific spatial conformation, and the relationship between function and structure was related to specific application of proteins. There are many studies on modification of protein gel and emulsifying properties from this perspective (Liu et al., 2015; Yu et al., 2021). Some researchers also found that pH value of egg white would gradually shift to alkaline accompanied by egg white thinning during storage, and the maximum could reach 9.7 (Huang et al., 2022a). Smith & Back (1965) showed that ovalbumin (OVA) would be converted to S-OVA with better resistance to heat denaturation when pH rose to 9–10. Hence, the thinning of egg white might be related to change of pH, but current studies have not fully linked the two.
This study focused on KEW thinning and simulated the thinning process of KEW by adjusting pH to 10 combining with mild heat treatment. Combined with changes of functional properties and molecular structure during thinning process, the mechanism of weak alkaline combined with mild heat treatment KEW was explored, and compared with alone heating thinning and natural thinning of egg white. It aimed to fully analyse and explore the natural and artificial phenomenon of “egg white thinning”, and provide theoretical support for the efficient utilisation of egg white.
Materials and methods
Preparation of KEW samples
Fresh eggs (egg weight: 50.0 ± 3.0 g) were provided by Fujian Guangyang Egg Industry Co., Ltd (Fujian, China). After a certain amount of egg white was taken out, thin egg white was removed through 20-mesh sieve (850 μm) to obtain KEW. The pH of KEW was adjusted to 10.0 by 1 mol/L NaOH, and it was balanced at room temperature (RT) for 1 h. The collected KEW was placed in a digital display constant temperature water bath water (HH-4, Guohua Electric Appliance Co., Ltd, China) at 50 °C and heated for a certain time to obtain samples for subsequent experimental analysis. Namely: 10 min (E-10), 20 min (E-20), 30 min (E-30), 40 min (E-40), 50 min (E-50), 60 min (E-60).
Rheological measurement
The steady-state rheology of different KEW samples was measured according to the method in reference with slightly modification (Ai & Jiang, 2021; Lin et al., 2021; Huang et al., 2022b). Parallel plate PP50 geometry of the Physical MCR 301 Rheometer (Anton Paar, Germany) was used for measurement. The gap width was set as 1 mm and steady-state rheological viscosity within shear rate range of 1–100 s−1 was recorded.
Measurement of particle size and ζ-potential
The determination of particle size and ζ-potential were referred to method of previous study with a few modifications (Huang et al., 2022c). The freeze-dried powder of protein samples was dispersed to 10 mg/mL in phosphate buffer (pH 7.4) for determination of ζ-potential (ZS90, Malvern Zetasizer Nano, UK). The sample solution was further diluted 10 times for particle size determination at RT.
Measurement of surface hydrophobicity
Add 200 μL of bromophenol blue solution (1 mg/mL) to 1 mL of diluted protein solution. After 10 min of RT stirring, mixture was centrifuged at 8000 × g for 15 min. After supernatant had been diluted, the absorbance was measured at 595 nm and recorded as A. The protein solution was replaced by deionised water as a blank sample, and absorbance was denoted as A0. Surface hydrophobicity was calculated by eq. (1):
Protein solubility
The phosphate buffer was applied to dissolve each sample to a concentration of 5 mg/mL. Take 5 mL of solution and centrifuged at 4 °C and 8000 × g for 10 min. The biuret method was used to measure the protein concentration in the supernatant. Solubility was expressed as the percentage of protein concentration m1 of the supernatant after centrifugation to that of m0 before centrifugation (Huang et al., 2021b). Solubility was calculated according to formula (2):
Texture profile analysis (TPA)
The determination of texture was referred to method of previous study with a few modifications (Liu et al., 2020b; Luo et al., 2021; Wu et al., 2021; Luo et al., 2022). The protein samples were placed in a constant temperature water bath at 90 °C for 30 min, and cut into 1.5 × 1.5 cm of the same size, and then placed overnight at 4 °C. After naturally recovering to RT, the gels' texture profile was assessed by SMSTA TA.XT Plus texture analyser (Stable Micro Systems Co., Ltd., UK). The probe was P/36R, test parameters were set as follows: pre-testing and post-testing speed were 2 mm/s, testing speed was 1 mm/s, contact point pressure was 5 g, and deformation degree was 40%.
Water holding capacity (WHC)
According to the method of Huang et al. (2021a) with slight modifications. The protein gel balanced to RT was about 3 g and its mass was accurately called M1. It was wrapped with double filter paper and placed in a 50 mL centrifuge tube, centrifuged at 5000 × g for 10 min at 4 °C. The mass after centrifugation was recorded as M2. WHC was calculated by formula (3):
Measurement of emulsifying property
Mix the protein sample with Tris–HCl buffer solution (pH 7.4) and 3 mL corn oil. This was then homogenised with a digital ultra turrax (T25, IKA company, Germany) at 10 000 r/min for 1 min. Then 40 μL of emulsion was quickly removed and mixed with 5 mL SDS solution (0.1%, w/v). The absorbance was measured as A0 at 500 nm with an ultraviolet–visible spectrophotometer (UV-1780PC, Shimadzu Co. Ltd, Suzhou, China), indicating emulsifying activity (EAI). After standing for 10 min, and the absorbance value was determined as A10 by the same method. The emulsion stability index (ESI) was calculated according to eq. (4):
SDS-PAGE
SDS-PAGE was performed according to previous method (Wang et al., 2021). The protein samples were dissolved to 6 mg/mL, and mixed with loading buffer in proportion, then boiled for 10 min. Samples were cooled to RT, centrifuged at 10 000 × g for 10 min, and then 5 μL was added to each sample hole. The voltage of electrophoresis was set as 100 V. After electrophoresis, gel sheet was stained with 0.2% Coomassie Bright Blue G-250, destained with deionised water and photographed with a gel imager.
Fourier transform infrared spectrum (FT-IR)
Fourier transform infrared spectrum (NicoletiS5, ThermoFisher Scientific, Waltham, USA) was investigated according to the (Li et al., 2021). The entire band in 4000−400 cm−1 range, second derivative of amide I band (1700−1600 cm−1) of original data was performed using OMNIC6.0 data processing software. Then changes of protein samples absorption peaks were analysed.
Fluorescence spectroscopy
The fluorescence intensity of different KEW was measured by RF-5301 fluorescence spectrophotometer (Shimadzu, Japan). Samples were diluted to 0.1 mg/mL in phosphate buffer. Excitation light wavelength was 285 nm and scanning wavelength was 300–450 nm. Excitation and emission slits were 3 nm and 5 nm, respectively (Geng et al., 2021).
Statistical analysis
All experiments were conducted in triplicate and presented as the mean ± standard deviation. The statistical analysis was analysed using SPSS 24.0.0 (SPSS Inc., Chicago, IL, USA), and results were considered statistically significant at P < 0.05 (Duncan multiple tests).
Results and discussion
Effects on molecular aggregation of KEW protein treated with mild heating under weak alkaline condition
Rheological property analysis
Rheological property of proteins is closely connected with structural and molecular characteristics, and can also represent morphological behaviour of physical cross-linking, so it is widely used in the shear response of gels (Luo et al., 2022). Figure 1 revealed the dependence of KEW viscosity on shear rate under weak alkaline combined with mild heat treatment. At the shear rate of 0–10 s−1, apparent viscosity of KEW descended rapidly with the increase of shear rate, indicating the KEW fluid exhibited pseudoplasticity. This phenomenon was often interpreted as the result of insufficient polymer entanglement. When the shear rate was greater than 20 s−1, apparent viscosity of KEW gradually closed to a straight line, suggesting that gel elasticity of KEW had been destroyed completely and gradually approached Newtonian fluid. It could be seen that apparent viscosity of KEW declined after mild heat treatment under weak alkaline condition, which indicated that it was reasonable to use this method to simulate thinning of KEW. With the extension of heating time, apparent viscosity presented a downward trend and E-40 reached the minimum value. Gao et al. (2020a) illustrated that treatment of protein under extreme pH conditions facilitated protein structural expansion to achieve MG state with similar viscosity to initial state. When high shear viscosity tended to a straight line in the later stage, the viscosity of samples treated with mild heating under weak alkaline condition were greater than KEW. It manifested the intermediate state formed by KEW weak alkaline combined with mild heat treatment had a stronger internal force. Luo et al. (2022) studied KEW with mild heating and proposed that the degree of change in KEW viscosity depended on the size of aggregates formed during heat treatment. Therefore, the structure of KEW protein was gradually opened in the process of weak alkaline combined with mild heat treatment. However, the viscosity raised with extension of heating time, which might be due to the cross-linking and aggregation of proteins caused by prolonged heating time.
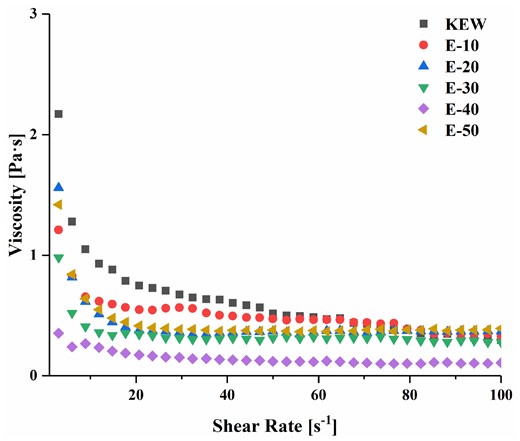
Effect on rheology of KEW protein treated with mild heat under weak alkaline condition. Note: 10 min (E-10), 20 min (E-20), 30 min (E-30), 40 min (E-40), 50 min (E-50), 60 min (E-60).
Particle size and ζ-potential analysis
Since the change of pH would lead to dynamic change of surface charge and electrostatic repulsion of proteins, the particle size and ζ-potential of proteins in solution were measured to evaluate dispersion and aggregation state (Gao et al., 2021). As seen in Fig. 2a, particle size of KEW significantly increased by 40.9% of the original size after weak alkaline combined with mild heat treatment. The particle size of KEW declined significantly with the extension of mild heating time. It meant that structure of KEW protein gradually unfolded after mild heating, the interactions within proteins were destroyed by weak alkaline combined with mild heat treatment. It was consistent with the descend of apparent viscosity in rheology, and keep in with the change of particle size in natural thinning of egg white during storage. Nevertheless, when the time was continuously extended, particle size of E-50 presented an increasing trend, which might be due to the aggregation of protein molecules caused by long-term thermal exposure of the protein. Meanwhile, it could be seen that particle size of KEW in the simulated thinning process was larger than the original sample, which further indicated the change of alkaline environment caused sample to form MG state (Alaei et al., 2021).
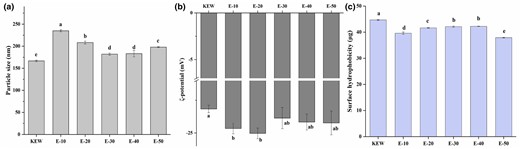
Particle size (a), ζ-potential (b) and surface hydrophobicity (c) changes of KEW protein treated with mild heat under weak alkaline condition.
ζ-potential can reflect the repulsion between charged particles in solution and is directly related to stability of protein dispersion system. It was generally believed that the higher the absolute ζ-potential value, the more stable solution system (Liu et al., 2022a). Figure 2b showed that ζ-potential of samples were all negative, manifesting that molecular surface of KEW protein was dominated by alkaline amino acids. The stability of samples treated with mild heating under weak alkaline condition was remarkably improved. Objectively proving that although the molecular particle size of MG state was prominently enhanced, dispersibility and stability of protein in solution were not damaged. When it was in an alkaline environment, because KEW itself had a negative charge, the repulsion between protein molecules reinforced. It was beneficial to reduce the aggregation of molecules and improved the stability of the solution. The better molecular stability, the stronger gel capability (Gao et al., 2018), which still needed to be further verified the gel property by TPA. Hence, the molecular particle size of KEW gradually diminished in the process of simulated thinning by weak alkaline combined with mild heat treatment. The better dispersion, the better stability of this system.
Surface hydrophobicity analysis
Surface hydrophobicity can reflect unfolding situation of proteins and exposure degree of hydrophobic groups, which is in connection with hydrophobic interaction between proteins and functional properties of proteins. When more hydrophobic groups were exposed, more bromophenol blue bound to protein molecules. It could be known from Fig. 2c that surface hydrophobicity of KEW first presented a downward trend after weak alkaline combined with mild heat treatment. This might be due to denaturation of protein by the base shift, resulting in the MG state. The particle size was strikingly increased, and the surface hydrophobic groups were embedded to a certain extent. With the prolongation of heating time, surface hydrophobicity rose and reached the maximum value at E-40. This indicated that mild heating treatment promoted gradual unfolding of protein structure, and hydrophobic groups hidden in the molecule were exposed. Thus, it was conducive to the formation of chemical bonds between protein molecules, and finally form a denser gel network structure (Li et al., 2020a). The interaction between samples continued to strengthen after continuous heating, which was easy to form aggregates, thus reducing surface hydrophobicity.
Effects on functional properties of KEW protein treated with mild heating under weak alkaline condition
Protein solubility analysis
Protein solubility is strongly linked to the functional properties of proteins. Solubility of KEW first presented a downward trend after weak alkaline combined with mild heat treatment (Fig. 3a). It might be related to formation of MG state and larger particle size of KEW treated with mild heating under weak alkaline condition. With the extension of heating time, solubility showed a trend of first increasing and then decreasing, illustrating that protein structure gradually unfolded and particle size reduced in this process. These were beneficial to dispersion of protein and the solubility conspicuously improved. The continuous heating made protein reunite, hydrophilic groups were wrapped in protein molecules, and stability of this system declined. Meanwhile, the variation trend of solubility was agreement with surface hydrophobicity, revealing that hydrophilic and hydrophobic groups might be exposed simultaneously when the protein structure was opened (Sheng et al., 2018). The solubility changes of KEW thinning induced by weak alkaline combined with mild heating were in keeping with that of natural egg white thinning. While the surface hydrophobicity was different, suggesting that solubility was closely related to depolymerization of internal structure of egg white and descend of particle size. It was in line with the theory proposed by Zhou & Yang (2020) that there was an equilibrium relationship between the enhancement of protein particle size, decrease of surface hydrophobicity and solubility in a system.

Solubility (a), WHC (b), emulsifying property (c) changes of KEW protein treated with mild heat under weak alkaline condition.
Texture profile analysis (TPA)
Texture can simulate the masticatory process of two extrusion deformation cycles, and is often used as an important index to evaluate gel. Table 1 exhibited specific changes of hardness, springiness, cohesiveness and chewiness of KEW at different time of mild heating under weak alkaline condition. As the maximum force when being extruded for the first time, hardness can characterise the strength of thermal gel. It could be concluded from Table 1 that hardness of KEW was the highest, and the hardness of egg white declined significantly after shifting pH. This was contrary to the increasing trend of thermal gel that naturally egg white thinning during storage. The results manifested that gel strength of natural egg white was not only affected by pH, but also closely relevant to the interaction of egg white protein molecules. Meanwhile, it also proved that the elevation in hardness of egg white thermal gel during storage might be correlated to water loss (Huang et al., 2022a). As the heating time prolonged, hardness of KEW gel decreased first and then raised, the minimum hardness of E-40 was 454.070 g. E-50 presented an increasing trend, demonstrating that KEW possibly accumulated and cross-linked due to prolonged heating time. This was consistent with the results of surface hydrophobicity and ζ-potential above.
Samples . | Hardness (g) . | Springiness . | Cohesiveness . | Chewiness . |
---|---|---|---|---|
KEW | 572.248 ± 4.713a | 0.949 ± 0.001c | 0.775 ± 0.004d | 413.625 ± 6.273a |
E-10 | 467.248 ± 2.309c | 0.961 ± 0.002a | 0.843 ± 0.002b | 387.876 ± 12.819cd |
E-20 | 476.201 ± 4.800c | 0.962 ± 0.002a | 0.854 ± 0.001a | 397.037 ± 2.837bc |
E-30 | 455.039 ± 8.613d | 0.956 ± 0.007ab | 0.836 ± 0.004c | 367.293 ± 3.643 e |
E-40 | 454.070 ± 6.960d | 0.962 ± 0.003a | 0.857 ± 0.005a | 377.678 ± 5.239de |
E-50 | 510.233 ± 0.402b | 0.954 ± 0.005bc | 0.842 ± 0.003bc | 408.995 ± 6.898ab |
Samples . | Hardness (g) . | Springiness . | Cohesiveness . | Chewiness . |
---|---|---|---|---|
KEW | 572.248 ± 4.713a | 0.949 ± 0.001c | 0.775 ± 0.004d | 413.625 ± 6.273a |
E-10 | 467.248 ± 2.309c | 0.961 ± 0.002a | 0.843 ± 0.002b | 387.876 ± 12.819cd |
E-20 | 476.201 ± 4.800c | 0.962 ± 0.002a | 0.854 ± 0.001a | 397.037 ± 2.837bc |
E-30 | 455.039 ± 8.613d | 0.956 ± 0.007ab | 0.836 ± 0.004c | 367.293 ± 3.643 e |
E-40 | 454.070 ± 6.960d | 0.962 ± 0.003a | 0.857 ± 0.005a | 377.678 ± 5.239de |
E-50 | 510.233 ± 0.402b | 0.954 ± 0.005bc | 0.842 ± 0.003bc | 408.995 ± 6.898ab |
Different superscript letters in the same column indicate significant differences at P < 0.05.
Samples . | Hardness (g) . | Springiness . | Cohesiveness . | Chewiness . |
---|---|---|---|---|
KEW | 572.248 ± 4.713a | 0.949 ± 0.001c | 0.775 ± 0.004d | 413.625 ± 6.273a |
E-10 | 467.248 ± 2.309c | 0.961 ± 0.002a | 0.843 ± 0.002b | 387.876 ± 12.819cd |
E-20 | 476.201 ± 4.800c | 0.962 ± 0.002a | 0.854 ± 0.001a | 397.037 ± 2.837bc |
E-30 | 455.039 ± 8.613d | 0.956 ± 0.007ab | 0.836 ± 0.004c | 367.293 ± 3.643 e |
E-40 | 454.070 ± 6.960d | 0.962 ± 0.003a | 0.857 ± 0.005a | 377.678 ± 5.239de |
E-50 | 510.233 ± 0.402b | 0.954 ± 0.005bc | 0.842 ± 0.003bc | 408.995 ± 6.898ab |
Samples . | Hardness (g) . | Springiness . | Cohesiveness . | Chewiness . |
---|---|---|---|---|
KEW | 572.248 ± 4.713a | 0.949 ± 0.001c | 0.775 ± 0.004d | 413.625 ± 6.273a |
E-10 | 467.248 ± 2.309c | 0.961 ± 0.002a | 0.843 ± 0.002b | 387.876 ± 12.819cd |
E-20 | 476.201 ± 4.800c | 0.962 ± 0.002a | 0.854 ± 0.001a | 397.037 ± 2.837bc |
E-30 | 455.039 ± 8.613d | 0.956 ± 0.007ab | 0.836 ± 0.004c | 367.293 ± 3.643 e |
E-40 | 454.070 ± 6.960d | 0.962 ± 0.003a | 0.857 ± 0.005a | 377.678 ± 5.239de |
E-50 | 510.233 ± 0.402b | 0.954 ± 0.005bc | 0.842 ± 0.003bc | 408.995 ± 6.898ab |
Different superscript letters in the same column indicate significant differences at P < 0.05.
The springiness and cohesiveness of KEW showed a trend of significant improvement after weak alkaline combined with mild heat treatment, which indicated that gel could maintain its structural integrity well under the action of external force. It meant that KEW protein molecules would be partially expanded and denatured with the process of weak alkaline combined with heat treatment. In addition, the denaturation rate was higher than aggregation rate, so that its elasticity and cohesion were fully exploited (Wang et al., 2015). Heating for too long would damage its performance and lead to degradation. Studies have shown that highly elastic gel networks could be formed when the protein denaturation rate was higher than aggregation rate. This trend was inconsistent with the reported of hardness increase and springiness decrease of egg white after weak alkali treatment alone (Gao et al., 2020b). It was possible that when a large amount of NaOH was added to egg white, it would directly denature and form a gel state. In this paper, the condition of weak alkaline combined with mild heating of KEW was mild, which was more conducive to the unfold of gel network structure. Chewiness represented the energy required to simulate chewing food, and it was affected by hardness, springiness, and cohesiveness. Since hardness was a large order of magnitude, the variation trend was similar. It could be seen that KEW gel changed from hard and brittle to soft and tough after weak alkaline combined with mild heat treatment. The gel property of KEW outstandingly improved.
WHC analysis
Water holding capacity usually depends on the degree of interaction between protein and water, gel structure and water distribution, which is a critical characteristic of gel (Marin et al., 2018). Figure 3b showed that WHC of KEW gel elevated markedly after weak alkaline combined with mild heat treatment. E-30 had the best WHC, it increased by 10% compared with KEW. This was in conformity with the results of TPA, indicating that three-dimensional network structure of soft and tough thermal gel after weak alkaline combined with mild heat treatment was denser, and more water molecules could be embedded (Huang et al., 2021a). Cui et al. (2020) proposed that heat-induced gel might be due to the formation of aggregates by protein molecules through hydrophobic interactions and disulfide bonds. The size and density of aggregates would affect WHC of gel. Nieuwland et al. (2016) proposed that stretched small aggregates were easier to fuse than dense large aggregates, which could effectively fix water and had better WHC of gel. With the extension of heating time, the crosslinking degree and hardness of KEW protein strengthened and hardness improved, but the effect on WHC was not significant. The internal structure of insoluble aggregates was loose, which accelerated the release of water molecules, so the WHC would not enhance continuously.
Emulsifying property analysis
Emulsifying property is usually described in terms of EAI (the ability of proteins adsorbed at the interface between water and oil droplets in an emulsion to prevent gravity separation and flocculation) and ESI (the stability of emulsified system over a predetermined time period). Figure 3c showed that EAI enhanced significantly after weak alkaline combined with mild heat treatment and reached a maximum value of 0.623 at E-50 (increased by 49.7% compared with KEW). It demonstrated that this treatment enhanced protein-oil phase or protein–protein interactions. Wang et al. (2018) reported that exposure of hydrophobic amino acid side chain groups would allow more strong oil adsorption, thus improving EAI. Meanwhile, EAI might also be related to the significant improvement in protein solubility. ESI trend showed a similar trend to EAI with a significant increase of 49.1% over KEW. This might be related to pH deviation of system from KEW isoelectric point. At this time, the adsorbed proteins on the oil–water interface increased, the interface stability improved, the protein molecules were less likely to aggregate, and the dispersibility and solubility were excellent, thereby enhancing the ESI. Li et al. (2020b) declared that the decline of protein particle size would enhance flexibility and stretching ability of molecules around oil droplets, resulting in a denser and thicker oil–water interface.
Effect on structure characteristics of KEW protein treated with mild heating under weak alkaline condition
SDS-PAGE analysis
SDS-PAGE was used to investigate the specific changes of protein in the process of simulated KEW thinning by weak alkaline combined with mild heat treatment. Figure 4 presented that molecular weight in egg white from large to small can be divided as follows: OVM (β-OVM with high glycated degree, α2-OVM and α1-OVM with low glycated degree), ovotransferrin, ovoinhibitor, ovalbumin and lysozyme. With the extension of heating time, the most significant change was in OVM. This trend coincided with the previous study (Huang et al., 2022a). The bands with the largest molecular weight were β-OVM in KEW, E-10 and E-20. While β-OVM was gradually degraded in the samples after E-30, meaning that β-OVM played a dominant role in the thinning of egg white (Liu et al., 2022b). Luo et al. (2022) showed that treatment at 30–60 °C did not affect the distribution of KEW protein, and further improved the temperature only aggravated aggregation degree of egg white protein. However, with the prolonged heating time under weak alkaline condition, the molecular weight of three subunits in OVM exhibited a declining trend in this study, which was like to the changes in egg white electrophoretic bands under natural thinning. It was strongly demonstrated that intrinsic driving force of OVM degradation was the gradual alkalization of pH environment of egg white protein. The gradual descend of molecular weight might also be related to the change of particle size. Johnson & Zabik (1981) investigated the denaturation temperatures of each protein in egg white, and illustrated that OVM still failed to present gel state when heated at 90 °C for 2 h. It could be shown objectively that there were multiple proteins or subunits interactions in the whole egg protein sol system, which might be conducive to formation of gel and reduce the denaturation temperature of OVM. When the pH was gradually alkalised, the interactions between proteins changes, especially the interactions related to OVM (Cotterill & Winter, 1955). It possibly led to depolymerization of skeleton protein in microscopic state and thinning in egg white macroscopic state. Thus, it was verified that pH was the intrinsic driving force to promote egg white thinning.
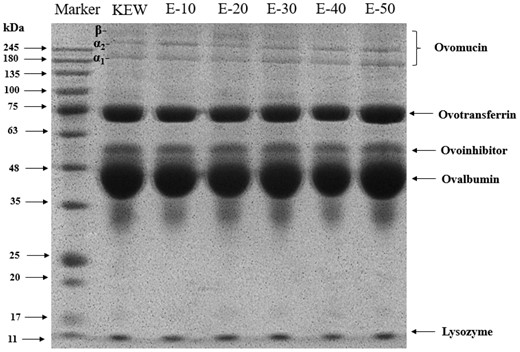
SDS-PAGE changes of KEW protein treated with mild heat under weak alkaline condition.
FT-IR analysis
The infrared spectrum of samples was shown in Fig. 5a. There was little change in peak shape and peak position among different samples, suggesting that the primary structure of KEW has no significant change after weak alkaline combined with mild heat treatment. Therefore, the amide I band at 1600–1700 cm−1 was integrated to obtain the change rule of secondary structure (α-helix, β-sheet, β-turn and random coil) of KEW after weak alkaline combined with mild heat treatment. It could be observed from Fig. 5b that β-sheet and β-turn of KEW account for about 65% of secondary structure, which was consistent with previous studies (Huang et al., 2022a). This value declined prominently after weak alkaline combined with mild heat treatment, mainly due to the remarkable downward trend of β-sheet. At the same time, both β-turn and random coil exhibited a trend of outstanding enhancement, illustrating that secondary structure of KEW gradually unfolded at this time, and fully stretched at E-40. The α-helix also had a slight upward trend with the thinning of KEW by weak alkaline combined with mild heat treatment. β-sheet, as a more stable secondary structure, displayed a more dramatic downward trend, demonstrating that the order of secondary structure was fully destroyed (Gao et al., 2020b). Hu et al. (2013) reported that β-sheet content was also associated with hydrophobic interaction of proteins, and its decrease reflected that exposure of hydrophobic sites within molecules, which in keeping with the result of surface hydrophobicity. With the progress of heat treatment, the secondary structure of samples tended to develop in the direction of stretched and disordered. The original rigid structure of KEW was destroyed and molecular flexibility improved. Thus, the results of the emulsifying property above were verified.
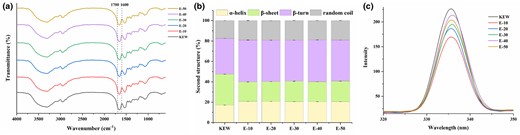
Changes in FT-IR (a), secondary structure (b) and fluorescence spectroscopy (c) of KEW protein treated with mild heat under weak alkaline condition.
Fluorescence spectroscopy analysis
Fluorescence spectrum is mainly derived from tryptophan (Try) residues that are sensitive to microenvironmental changes, and can characterise the changes of tertiary structure of proteins. It could be observed from Fig. 5c that the peak of samples before and after weak alkaline combined with mild heat treatment occurred at 336 nm. Compared with KEW, the fluorescence intensity of E-10 was lower, it might be responsible for pH change of KEW had a great influence on the microenvironment spatial conformation of its chromophore. The polarity of the fluorophore microenvironment was enhanced, thereby reducing fluorescence intensity. With the gradual extension of mild heat treatment time, fluorescence intensity exhibited a upward trend, suggesting the tertiary structure of protein gradually unfolded and Try residue was exposed. This result was in line with the study of Liu et al. (2020a). It demonstrated that mild heat treatment contributed to exposure of chromophore, and the hydrophobic interactions within protein molecules were destroyed, exposing more hydrophobic regions (consistent with the surface hydrophobicity result). This trend was also in fit with change in fluorescence intensity of natural thinning of KEW during storage, manifesting that essence of thinning was the unfolding of protein structure and depolymerization of skeleton protein. As the heating time continued to extend, protein molecules aggregated and the fluorescence intensity descended again.
Correlation analysis
The molecular aggregation, functional properties were closely related to structure of KEW protein treated with mild heating treatment under alkaline conditions. Table 2 presented fluorescence intensity was significantly (P < 0.01) negative correlation with particle size with a correlation coefficient of 0.953. And particle size showed a remarkable negative correlation (P < 0.05) with ζ-potential. It indicated that protein was easy to disperse in the system when particle size was small, which made the system stable. Meanwhile, more hydrophobic regions can be exposed and fluorescence intensity increased. The secondary structure of protein was significantly correlated with hardness and WHC of gel (P < 0.05), among which β- sheet was positively correlated with hardness and negatively correlated with WHC, while other secondary structures were opposite. This strongly proved that gel property of protein was related to its secondary structure. With the gradual stretching of secondary structure, aggregation of protein molecules decreased, and small aggregates were easier to fuse, which can effectively fix water, thus possessing excellent WHC, and the gels show was soft and tough at this time.
Correlation analysis of molecular aggregation, function properties and structure in KEW protein treated with mild heat under weak alkaline condition
. | particle size . | ζ-potential . | Hardness . | Springness . | WHC . | EAI . | ESI . | α-helix . | β-sheet . | β-turn . | random coil . | flourescence intensity . |
---|---|---|---|---|---|---|---|---|---|---|---|---|
Particle size | 1 | |||||||||||
ζ-potential | −0.821* | 1 | ||||||||||
Hardness | −0.453 | 0.635 | 1 | |||||||||
Springness | 0.674 | −0.867* | −0.634 | 1 | ||||||||
WHC | 0.348 | −0.614 | −0.790 | 0.390 | 1 | |||||||
EAI | −0.024 | −0.354 | −0.606 | 0.269 | 0.882* | 1 | ||||||
ESI | 0.140 | −0.333 | −0.436 | 0.122 | 0.855* | 0.903* | 1 | |||||
α-helix | 0.636 | −0.808 | −0.889* | 0.647 | 0.927** | 0.712 | 0.690 | 1 | ||||
β-sheet | −0.633 | 0.806 | 0.905* | −0.679 | −0.912* | −0.710 | −0.674 | −0.998** | 1 | |||
β-turn | 0.638 | −0.806 | −0.914* | 0.720 | 0.884* | 0.698 | 0.648 | 0.988** | −0.996** | 1 | ||
Random coil | 0.590 | −0.766 | −0.884* | 0.575 | 0.951** | 0.732 | 0.715 | 0.995** | −0.988** | 0.972** | 1 | |
Flourescence intensity | −0.935** | 0.794 | 0.594 | −0.581 | −0.450 | −0.006 | −0.113 | −0.699 | 0.686 | −0.674 | −0.679 | 1 |
. | particle size . | ζ-potential . | Hardness . | Springness . | WHC . | EAI . | ESI . | α-helix . | β-sheet . | β-turn . | random coil . | flourescence intensity . |
---|---|---|---|---|---|---|---|---|---|---|---|---|
Particle size | 1 | |||||||||||
ζ-potential | −0.821* | 1 | ||||||||||
Hardness | −0.453 | 0.635 | 1 | |||||||||
Springness | 0.674 | −0.867* | −0.634 | 1 | ||||||||
WHC | 0.348 | −0.614 | −0.790 | 0.390 | 1 | |||||||
EAI | −0.024 | −0.354 | −0.606 | 0.269 | 0.882* | 1 | ||||||
ESI | 0.140 | −0.333 | −0.436 | 0.122 | 0.855* | 0.903* | 1 | |||||
α-helix | 0.636 | −0.808 | −0.889* | 0.647 | 0.927** | 0.712 | 0.690 | 1 | ||||
β-sheet | −0.633 | 0.806 | 0.905* | −0.679 | −0.912* | −0.710 | −0.674 | −0.998** | 1 | |||
β-turn | 0.638 | −0.806 | −0.914* | 0.720 | 0.884* | 0.698 | 0.648 | 0.988** | −0.996** | 1 | ||
Random coil | 0.590 | −0.766 | −0.884* | 0.575 | 0.951** | 0.732 | 0.715 | 0.995** | −0.988** | 0.972** | 1 | |
Flourescence intensity | −0.935** | 0.794 | 0.594 | −0.581 | −0.450 | −0.006 | −0.113 | −0.699 | 0.686 | −0.674 | −0.679 | 1 |
Values denoted with an asterisk is significant at the 0.05 level (P < 0.05). Values denoted with two asterisk is significant at the 0.01 level (P < 0.01).
Correlation analysis of molecular aggregation, function properties and structure in KEW protein treated with mild heat under weak alkaline condition
. | particle size . | ζ-potential . | Hardness . | Springness . | WHC . | EAI . | ESI . | α-helix . | β-sheet . | β-turn . | random coil . | flourescence intensity . |
---|---|---|---|---|---|---|---|---|---|---|---|---|
Particle size | 1 | |||||||||||
ζ-potential | −0.821* | 1 | ||||||||||
Hardness | −0.453 | 0.635 | 1 | |||||||||
Springness | 0.674 | −0.867* | −0.634 | 1 | ||||||||
WHC | 0.348 | −0.614 | −0.790 | 0.390 | 1 | |||||||
EAI | −0.024 | −0.354 | −0.606 | 0.269 | 0.882* | 1 | ||||||
ESI | 0.140 | −0.333 | −0.436 | 0.122 | 0.855* | 0.903* | 1 | |||||
α-helix | 0.636 | −0.808 | −0.889* | 0.647 | 0.927** | 0.712 | 0.690 | 1 | ||||
β-sheet | −0.633 | 0.806 | 0.905* | −0.679 | −0.912* | −0.710 | −0.674 | −0.998** | 1 | |||
β-turn | 0.638 | −0.806 | −0.914* | 0.720 | 0.884* | 0.698 | 0.648 | 0.988** | −0.996** | 1 | ||
Random coil | 0.590 | −0.766 | −0.884* | 0.575 | 0.951** | 0.732 | 0.715 | 0.995** | −0.988** | 0.972** | 1 | |
Flourescence intensity | −0.935** | 0.794 | 0.594 | −0.581 | −0.450 | −0.006 | −0.113 | −0.699 | 0.686 | −0.674 | −0.679 | 1 |
. | particle size . | ζ-potential . | Hardness . | Springness . | WHC . | EAI . | ESI . | α-helix . | β-sheet . | β-turn . | random coil . | flourescence intensity . |
---|---|---|---|---|---|---|---|---|---|---|---|---|
Particle size | 1 | |||||||||||
ζ-potential | −0.821* | 1 | ||||||||||
Hardness | −0.453 | 0.635 | 1 | |||||||||
Springness | 0.674 | −0.867* | −0.634 | 1 | ||||||||
WHC | 0.348 | −0.614 | −0.790 | 0.390 | 1 | |||||||
EAI | −0.024 | −0.354 | −0.606 | 0.269 | 0.882* | 1 | ||||||
ESI | 0.140 | −0.333 | −0.436 | 0.122 | 0.855* | 0.903* | 1 | |||||
α-helix | 0.636 | −0.808 | −0.889* | 0.647 | 0.927** | 0.712 | 0.690 | 1 | ||||
β-sheet | −0.633 | 0.806 | 0.905* | −0.679 | −0.912* | −0.710 | −0.674 | −0.998** | 1 | |||
β-turn | 0.638 | −0.806 | −0.914* | 0.720 | 0.884* | 0.698 | 0.648 | 0.988** | −0.996** | 1 | ||
Random coil | 0.590 | −0.766 | −0.884* | 0.575 | 0.951** | 0.732 | 0.715 | 0.995** | −0.988** | 0.972** | 1 | |
Flourescence intensity | −0.935** | 0.794 | 0.594 | −0.581 | −0.450 | −0.006 | −0.113 | −0.699 | 0.686 | −0.674 | −0.679 | 1 |
Values denoted with an asterisk is significant at the 0.05 level (P < 0.05). Values denoted with two asterisk is significant at the 0.01 level (P < 0.01).
Conclusion
In this study, the aggregation state was described by rheology, particle size, and surface hydrophobicity. The functional properties were characterised by solubility, TPA and WHC, and structural changes of KEW were characterised by SDS-PAGE and spectroscopy during weak alkaline combined with mild heat treatment. The results showed that KEW first formed MG state during the thinning process, particle size increased significantly, surface hydrophobicity and solubility decreased conspicuously. With the extension of heating time, KEW protein structure gradually unfolded. The particle size of protein markedly descended, suggesting that protein could be more evenly dispersed in solution system, and solubility improved accordingly. Meanwhile, surface hydrophobicity enhanced with the unfolding of protein structure. The stretching of structure significantly improved gel property, WHC and emulsifying properties of proteins. The natural thinning of KEW during storage and alone heating thinning was compared, SDS-PAGE results elucidated that KEW thinning was closely in connection with OVM degradation, alkalization of pH was the indispensable intrinsic driving force of KEW thinning. This study was helpful to fully analyse the natural phenomenon of KEW thinning and provide theoretical basis for egg white storage and application.
Acknowledgments
This study was financially supported through grants from the National Natural Science Foundation of China (No. 31871732), the Key Project of Natural Science Foundation of Guizhou Province (No. KY [2022] key 036), the Open Foundation for Key Laboratory of Environmental Pollution Monitoring and Disease Control, Ministry of Education (No. KY [2022] 382).
Author contributions
Liu Lan: Conceptualization (equal); formal analysis (equal); writing – original draft (equal); writing – review and editing (equal). Yongyan Wu: Writing – review and editing (equal). Wenshan Duan: Investigation (equal). Hongbo Song: Conceptualization (equal); software (equal). Fang Geng: Supervision (equal); validation (equal). Jinqiu Wang: Investigation (equal); methodology (equal). Qun Huang: Project administration (equal); supervision (equal). Shaofeng Wei: Formal analysis (equal); supervision (equal); validation (equal).
Conflict of interest
The authors declare that they have no conflict of interest.
Ethical approval
Ethics approval was not required for this research.
Data availability statement
Research data are not shared.
References
Note: Gao et al. studied the effects of of extreme acid-induced soybean glycinin molten globules state on foaming ability and structure, which provided a reference for experimental methods and theoretical background analysis for this study.
Note: Huang et al. studied the mechanism of mulberry polyphenols inhibiting oxidation of beef myofibrillar protein, which was valuable for the experimental method and writing of this paper.
Note: Sheng et al. explored the relationship between surface hydrophobicity and solubility, which had important guiding significance for this paper.
Note: Zhou et al. found that there was an equilibrium relationship between the enhancement of protein particle size, decrease of surface hydrophobicity and solubility in a system. This provided a theoretical support for writing of this paper.
Author notes
Authors contributed equally to this work.