-
PDF
- Split View
-
Views
-
Cite
Cite
Mi Tang, Hongjie Dai, Liang Ma, Yong Yu, Tingwei Liu, Xin Feng, Weijie Hu, Yuan Li, Yuhao Zhang, Degradation of structural proteins and their relationship with the quality of Mandarin fish (Siniperca chuatsi) during post-mortem storage and cooking, International Journal of Food Science and Technology, Volume 55, Issue 4, April 2020, Pages 1617–1628, https://doi.org/10.1111/ijfs.14421
- Share Icon Share
Abstract
This study was carried out to investigate the changes in the microstructure and structural proteins (titin, nebulin, desmin and tropomyosin (TPM)) of mandarin fish muscle as well as the relationship between the structural proteins and the quality characteristics of fish muscle during post-mortem storage and after cooking. During post-mortem storage, titin was degraded initially, accompanied with an increase of the I-band length. Subsequently, the degradation of desmin induced a gradual breakage of the myofibrils. The following degradation of nebulin accelerated the destruction of N2-lines, which occurred with a slightly fuzzy Z-disc. Finally, TPM began to degrade, and the remaining desmin was also degraded further. Principal component analysis (PCA) and correlation analysis indicated that the changes in the quality characteristics of fish muscle were intimately related to the degradation of structural proteins. Hence, these structural proteins could be the biomarkers to monitor the quality characteristics of fish muscle.
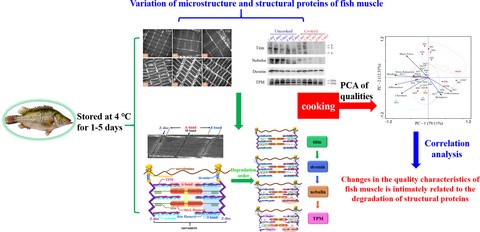
Introduction
Mandarin fish (Siniperca chuatsi), a popularly cultured freshwater species in China, is widely favored by the Chinese due to its good taste, few intermuscular fish bones, fast growth and high economic value (Mo et al., 2019). The price of this fish in the market is approximately 4–10 times as much as that of traditional carps, and it is always sold as the chilled prepared fish (stored at 4 °C). However, it still fails to fully utilise its economic value because of quality problems, and the existing problems are mainly attributed to the changes of chemical compositions and the degradation of muscle proteins (Aussanasuwannakul et al., 2010). Generally, myosin and action are the major proteins in myofibrillar proteins directly regulating the muscle contraction (Xiong, 2018), which played an important role in the textural quality of fish during post-mortem storage and processing (Subbaiah et al., 2015; Yu et al., 2018).
However, our previous study proved that there is no obvious degradation of myosin and actin in mandarin fish over the shelf life period (Sun et al., 2018a), which is inconsistent with the results of other researches (Ramirez-Suarez et al., 2008; Yang et al., 2014). Some published reports indicated that the quality characteristics of fish muscle might be influenced by structural proteins, regardless of myosin and actin (Ahmed et al., 2015; Singh & Benjakul, 2018). The changes in connective tissue and myofibrillar proteins correspond to the texture deterioration of fish muscle during post-mortem and thermal processing. These changes may include the weakening of myofibrils, breakdown of costameres and desmin, degradation of the Z-disc, proteolysis of titin and nebulin and TPM degradation (Ahmed et al., 2015). Ultimately, these changes would lead to the softening and tenderization of fish muscle. Therefore, the structural proteins, which are responsible for regulating the contraction of sarcomeres and maintaining the integrity of muscle tissues, are correlated to the variation of the myofibrillar structure. Some published reports have indicated that post-mortem proteolysis could influence the degradation of large structural proteins such as titin and nebulin, which are abundant in the myofibrils and contribute to meat quality (Kasamatsu et al., 2004; Huff Lonergan et al., 2010; Wu et al., 2014a). Titin accounts for approximately 30% of the total elasticity of myofibrils, and one end with nebulin is attached to the Z-disc and parallel to the titin filament (Ahmed et al., 2015). These two proteins are involved in maintaining and regulating the structure of myofibrils, whose degradation is correlated with the endogenous proteases and storage conditions, finally resulting in changes in the muscle quality (Caballero et al., 2009; Ge et al., 2018; Yang et al., 2019).
Additionally, our previous study has demonstrated that the low-abundance proteins of mandarin fish mainly include desmin and TPM by proteomics analysis, whose degradation could impact the quality of fish muscle (Sun, 2018b). Desmin is crucial to retaining the functional integrity of myofibrils as a distinct member of the intermediate filament family (Wojtysiak & Połtowicz, 2006). Its degradation can be observed in both ageing and freezing storage and corresponds to textural deterioration, leading to the disruption of the myofibril network structure and an adverse change in the water mobility and distribution (Cheng et al., 2014). TPM is associated with actin in the thin filaments, whose degradation would influence the meat tenderization, reflected by the weakening of the thin filaments and/or interaction between actin and myosin filaments (Delbarre-Ladrat et al., 2006). To date, there is still a lack of systematic research to elucidate the differences in the quality characteristics of fish muscle with different storage times during cooking, and the relationship between the degradation of structural proteins and the changes in the quality of fish muscle (before and after cooking) has not yet been reported.
The aim of this paper was to investigate the degradation of structural proteins (titin, nebulin, desmin and TPM) in mandarin fish using Western blotting and transmission electron microscopy (TEM) during post-mortem storage and cooking as well as speculate on their degradation mechanism. The variation of fish quality is analysed by the texture profile analysis (TPA), cooking loss, shear force and stress relaxation. Furthermore, the relationship between the degradation of structural proteins and the changes in the quality of fish muscle (before and after cooking) is analysed by PCA and correlation analysis.
Materials and methods
Preparation of mandarin fish blocks
The fresh mandarin fish with a mean weight 700 ± 50 g were purchased from the Tiansheng aquatic market in Chongqing, China and immediately killed using stunning by trained personnel. After being washed with tap water, the fish were stored at 4 °C for 3 h, and the dorsal muscle was cut into blocks (approximately 30 × 20 × 10 mm). Then, the fish blocks were packed in a crisper at 4 °C and sampled at different post-mortem times (1–5 days) according to Sun et al. (2018a). After being steamed for 5 min, the cooked fish blocks were obtained for a comparative study.
TEM
Uncooked and cooked fish blocks (steaming for 5 min) were cut into small cubes (5 × 5 × 5 mm) and soaked in 2.5% glutaraldehyde for fixation at 4 °C for 3 days. The TEM examination of the muscle structure was conducted as described by Wang et al., (2018) using a New Bio-TEM H-7650 (Hitachi, Tokyo, Japan).
Preparation of SDS-PAGE samples of whole muscle
The whole muscle samples were prepared by the reported method of Mitsuhashi et al., (2002) with a slight modification. The sample mixture was homogenised at 1600 g and then heated at 50 °C for 20 min. Then, it was further centrifuged at 1600 g for 5 min at 25 °C to remove the traces of insoluble components. The resulting supernatant was combined with the sample buffer (4% SDS, 20% glycerol, 10% β-mercaptoethanol, 0.125 M Tris, pH = 6.8) at a ratio of 1:2 (v/v) and heated at 100 °C for 5 min.
Western blotting
The electrophoresis was performed using a Bio-Rad Mini-Protein system (Bio-Rad Laboratories, Hercules, CA, USA). To detect the changes in titin and nebulin, 5% separating gel (acrylamide:N,N′-bis-methylene acrylamide = 37.5:1 (w/w), 10% (w/v) SDS, 98% TEMED, 10% (w/v) APS and 1.5 M Tris–HCl, pH = 8.8) and 4% stacking gel (acrylamide:N,N′-bis-methylene acrylamide = 37.5:1 (w/w), 10% (w/v) SDS, 98% TEMED, 10% (w/v) APS and 1.0 M Tris–HCl, pH = 6.8) were used under a constant 10 mA current. To detect the changes in the whole muscle proteins (desmin and TPM), 12% separating gel (acrylamide:N,N′-bis-methylene acrylamide = 37.5:1 (w/w), 10% (w/v) SDS, 98% TEMED, 10% (w/v) APS and 1.5 M Tris–HCl, pH = 8.8) and 5% stacking gel (acrylamide:N,N′-bis-methylene acrylamide = 37.5:1 (w/w), 10% (w/v) SDS, 98% TEMED, 10% (w/v) APS and 1.0 M Tris–HCl, pH = 6.8) were used under a constant 15 mA current.
After electrophoresis, the gels were soaked in semi-dry transfer buffer solutions (48 mM Tris, 39 mM glycine, 20% methanol, 1% SDS [only for titin and nebulin]) for 10 min. Then, the gels were transferred to PVDF membranes through a transfer apparatus (Bio-Rad Laboratories, Hercules, CA, USA), choosing the standard transfer mode for different times (titin, nebulin, desmin and TPM were transferred for 90, 60, 30 and 20 min, respectively). The membranes were blocked for 1 h at room temperature, and the blocking buffer and TBST were prepared according to the recommended protocols from Abcam. After being blocked, the electroblotted membranes were rinsed three times (5 min per rinse) in TBST. The saturated membranes were later probed with diluted primary antibody overnight at 4 °C. Antibodies and dilutions used were as follows: mouse monoclonal anti-titin antibody (Sigma T-9030, diluted 1: 1000); mouse monoclonal anti-nebulin antibody, (Sigma N-9891, diluted 1:400); rabbit polyclonal antibody to desmin (Abcam 86083, diluted 1:500); and rabbit polyclonal antibody to TPM1 (Thermo Fisher PA5-41996, diluted 1:800).
After overnight incubation, the membranes were repeatedly washed 5 times with TBST to remove excess antibody. Subsequently, the membranes were incubated with diluted secondary antibody, either anti-mouse (Sigma A-3688; diluted 1:1000) or anti-rabbit (Sigma R-5506; diluted 1:2000), for 2 h at room temperature. Finally, the membranes were washed again, and images of the corresponding blots were captured by ECL Western blotting substrate (QG218145, Thermo, Pierce, IL, USA) through FUSION Solo S (Vilber Lourmat, Paris, France). The Evolution-Capt was used to quantify the intensities of bands in each lane.
DSC analysis
Muscle samples (about 15 mg each) were placed in a sample cell of a DSC 4000 & Autosampler (PerkinElmer Ltd., Waltham, Massachusetts, USA) to follow the major endothermic transitions in the mandarin fish. The scans were recorded from 20 to 80 °C with a heating rate of 1 °C/min.
Determination of the thermal temperature curve
The fish blocks were steamed for 5 min, and the digital temperature probe was used to record the centre temperature during the heating process.
Assessment of the quality of mandarin fish
The TPA, shear force and stress relaxation were all evaluated with a TA.XT plus Texture Analyzer (Stable Microsystems Ltd., Surrey, England) with the corresponding programs.
TPA
Prior to texture analysis, the muscle samples were taken out from the refrigerator and blot-dried with a paper towel. The TPA parameters were set according to our previous studies (Sun et al., 2018a), each sample was carried out using a 50 mm cylinder probe (type TA25), test speed 1.0 mm/s at 80 % strain, compressing the samples twice with a 60 s interval between the two compressions and the final data came from five replicates.
Cooking loss and shear force
After the heating and cooling process, the muscle samples were removed from the sealing bag, and the cooking loss was immediately measured according to Tomaszewska-Gras et al., (2011). The weighed samples were taken for shear force analysis, which was also carried out on a TA.XT plus Texture Analyzer with an A-MORS attachment. The shear force was measured by the method described by Zang et al., (2017). Each muscle sample was cut parallel to the muscle fibres at a constant speed of 1.0 mm s-1 with a trigger force 10 g, and the compress was set as 100 % of the thickness of samples.
Stress relaxation
The parameters of the stress-relaxation tests were set as follows: samples were compressed by 30% with a 50 mm cylinder probe at a crosshead speed of 1 mm/s; the compression was kept constant for 45 s and the trigger force was 5 g; and the data acquisition rate was 500 pps.
Statistical analysis
All experiments were replicated three times, and mean values were expressed as the mean ± standard error (SE). Origin2019 was used for the nonlinear fitting. The sarcomere length was calculated by Nano Measurer (1.2). SPSS 19.0 software was used for statistical analysis. Results were considered significant at P < 0.05 and highly significant at P < 0.01, and PCA analysis was performed on Canoco software, version 5.0.
Results and discussion
Changes in the sarcomere
Fig. 1(A) depicts the TEM images of uncooked and cooked fish muscle stored at 4 °C for 1, 3 and 5 d, respectively. As shown in Fig. 1(A)a–c, no obvious change in uncooked muscles was observed within 5 days of storage, showing a clear M-band. However, the Z-disc became slightly fuzzy after 3 of days storage, implying its disruption. Figure S1 shows the changes in the sarcomere and I-band lengths during post-mortem storage. The sarcomere length significantly decreased from 1 to 3 days of storage (P < 0.05), and then evidently increased at the 5th day of storage (P < 0.05). Battaglia et al., (2016) reported that the sarcomere length of muscle was positively related to meat tenderness, which indicated that the tenderness of the muscle on day 3 was better than on the other days.
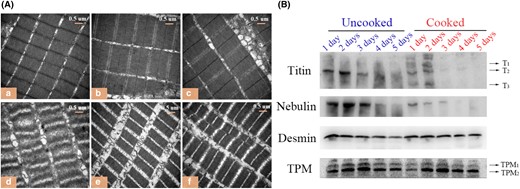
(A) TEM images of uncooked (a, b, c) and cooked (d, e, f) muscle stored at 4 °C for 1, 3 and 5 d. (B) Western blotting analysis for titin, nebulin, desmin and TPM in uncooked and cooked muscles.
However, as can be observed from Fig. 1(A)d-f, the ultrastructure of cooked fish muscle stored for 1 day changed more drastic than others. The possible explanation could be that the uncooked muscle stored for 1 day has a relatively intact sarcomere structure, in which the structural proteins scaffolding for the filaments have not yet degraded, thus the destruction to the original sarcomere structure was more obvious during the heating contraction process (Caballero et al., 2009; Wu, et al., 2014a).
Degradation of structural proteins
Titin
Titin is a molecular spring of myofibrils, having a molecular weight of 3000 kDa (T1), which spans an entire half of sarcomere and connects the M-band to Z-disc (Eckels et al., 2018; Herzog, 2018). When titin is degraded, it first degrades to 2400 kDa (T2) and then degrades to a molecular weight of 1200 kDa (T3) (Wu et al., 2014b). As illustrated in Fig. 1(B), titin primarily displayed as T2 for the uncooked samples on day 1. The uncooked muscles were stored for 3 h at 4 °C before sampling, and hence, the u-calpain was activated and T1 was degraded to T2 during this time (Koohmaraie & Geesink, 2006). Furthermore, T2 was found to be degraded at 1–3 days post-mortem and disappeared after 3 days post-mortem. For cooked muscles, titin was not only degraded into small molecular fragments but also formed macromolecular substances. The macromolecular substances might be the cross-linkers of titin components produced by the protein oxidation during cooking (Sobral et al., 2018). All components in cooked samples were undetectable after 2 days post-mortem, suggesting that titin was completely degraded during cooking, as indicated by the low contents of titin in raw fish after 2 days of storage.
Nebulin
Nebulin (600–900 kDa) is the fourth filament in the muscle, extending from the Z-disc to the tip of the filament and contributing to fixing the filament anchored at the Z-disc (Pappas et al., 2008). As depicted in Fig. 1(B), there was no obvious degradation of nebulin in uncooked fish blocks within 3 days post-mortem, but it almost disappeared at day 4. In comparison with uncooked muscles, nebulin was apparently degraded after being cooked and almost completely disappeared after 3 days post-mortem, suggesting the contribution of heating to the degradation of nebulin.
Desmin
Desmin, an intermediate filament, links to the adjacent myofibrils at their Z-disc levels and attaches the cell membrane to myofibrils (Robson & Huiatt, 1983). As shown in Fig. 1(B), the degradation of desmin was not apparent in uncooked or cooked samples. However, in both uncooked and cooked muscles (Table S1), their relative optical density (ROD) values reflected a slight degradation of desmin with prolonged storage time. The main reason might be that the calpain-induced degradation of desmin occurred on a limited basis, which apparently increased the activation of cathepsins at later stages of post-mortem storage (Caballero et al., 2009; Lomiwes et al., 2014).
TPM
TPM belongs to a regulatory protein of myofibrils, tightly entangling with the surface of the actin filament, which is heat-stable and water-soluble (Faisal et al., 2019). As illustrated in Fig. 1(B), TPM consisted of two subunits (TPM1 and TPM2), and both subunits showed an increasing trend within 3 days post-mortem whether in uncooked or cooked muscles, showing agreement with the study of Faisal et al. (2019) and Li et al., (2018). However, the reason for this trend remains unclear. With the further prolonged storage, the subunits of TPM began to degrade, which may due to the combined effects of cathepsins B, D and L (Ge et al., 2018). During this period, TPM was only slightly degraded after being cooked due to its heat stability.
The degradation pattern of structural proteins during post-mortem storage
According to the sarcomere changes observed in the TEM image (Fig. 1), the I-band first increased and then decreased at the 5th day of storage, and the Z-disc was gradually disrupted with prolonged storage time, implying that the degradation of structural proteins was correlated with the integrity of myofibrils (Lange et al., 2019). The NH2-terminal of titin is linked to the Z-disc, and the COOH-terminal is connected to the M-band region, spanning the half sarcomere with spring-like properties in the I-band (Herzog, 2018). Nebulin extends along the entire length of the thin filament, probably participating in regulating actin–myosin interactions in ageing muscle (Huff Lonergan et al., 2010). Desmin serves as an integral part of the intermediate filaments, stretching around the Z-disc and acting as a scaffold (Paulin & Li, 2004). Additionally, TPM also plays an indispensable role as a component of filaments that binds to actin (Kee et al., 2015).
From the Western blotting results of uncooked muscles, titin was apparently degraded at day 3, nebulin almost disappeared at day 4 and desmin was degraded with the prolonged storage time, while TPM began to degrade at day 4. The degradation of structural proteins is intimately related to calpains and cathepsins during post-mortem storage (Ge et al., 2018). In recent decades, the possible mechanism of endogenous enzymes on the protein degradation of freshwater fish has been extensively investigated. Calpains may be responsible for the degradation during the early post-mortem stage, while cathepsins may act in proteolysis during the later stage (Delbarre-Ladrat et al., 2006; Ahmed et al., 2015). Mitsuhashi et al. (2002) reported that approximately 80% of titin in jack mackerel was reduced after storage for 24 h, indicating that titin was sensitively degraded during the early stage of storage (Kasamatsu et al., 2004). Desmin was partially degraded due to the calpains occurring at special sites, and it may be subjected to further degradation during the later stage due to the presence of cathepsins (Lomiwes et al., 2014). The partial decrease of nebulin was attributed to the specificity of calpains for the degradation of N2-lines, which was a cytoskeletal network consisting of titin and nebulin that connected the thick and thin filaments to the Z-disc (Listrat et al., 2016). The cleavage of the rest of nebulin may be initiated by other proteases (Ahmed et al., 2015). During the later stage of storage, TPM was degraded due to the activation of cathepsins (Delbarre-Ladrat et al., 2006).
Based on the above analysis, the possible variation in the mechanism of structural protein degradation and raw fish microstructure during storage could be summarised in Fig. 2. During post-mortem storage, titin was initially degraded, accompanied with an increase in the I-band length. One possible explanation was that titin located in the I-band region, scaffolding for the myosin filaments, whose degradation would disrupt the microstructure of myofibril resulting in the change of the sarcomere length and meat tenderness. Meanwhile, the increase in I-band length of sarcomere could be caused by the weakening of the binding force between the filaments (Huff Lonergan et al., 2010). Therefore, the I-band length was influenced by titin degradation. Subsequently, desmin began to degrade, thus leading to a gradual breakage of the myofibrils linked by desmin. The followed degradation of nebulin accelerated the destruction of N2-lines along with a slightly fuzzy Z-disc. Finally, TPM began to degrade, and the remained desmin was also further degraded.
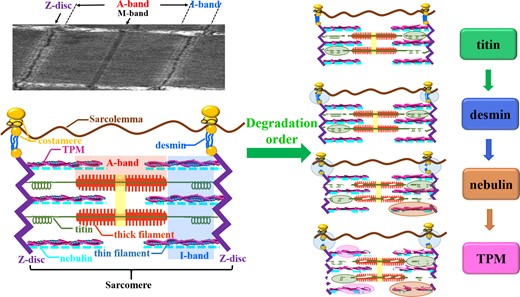
Degradation mechanism of structural proteins during post-mortem storage.
Denatured proteins and centre temperature of fish blocks during cooking
DSC is widely applied to detect the protein denaturation temperature, providing information on structural changes in proteins indirectly. As shown in Fig. 3(A) a, raw fish muscle showed three endothermic peaks, corresponding to myosin (50 °C), sarcoplasmic protein (58 °C) and actin (72 °C), respectively. After cooking for 60 s, all three endothermic peaks disappeared, implying the formation of the entire thermal denaturation of proteins. Additionally, the centre temperature alteration of fish blocks during thermal processing is presented in Fig. 3(A)b. The centre temperature of fish blocks was approximately 70 °C when cooked for 90 s, resulting in denaturation of these three proteins. The centre temperature reached approximately 100 °C when cooked for 300 s, which could guarantee the microbiological safety and quality of fish muscle.
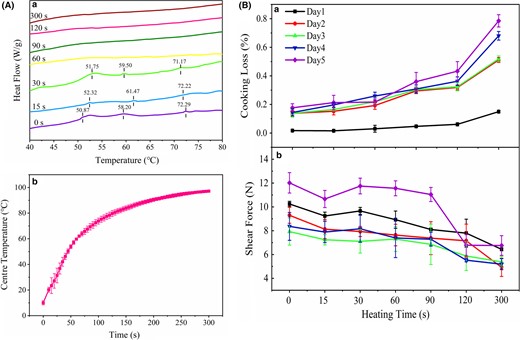
(A) The DSC of fish blocks at different cooking time illustrated in (a) and the variations of the centre temperature of fish blocks during cooking illustrated in (b). (B) Changes in cooking loss (a) and shear force (b) during cooking with different storage time (1–5 days).
Based on the results of the DSC and centre temperature of fish blocks, the heating process of fish blocks was determined as follows: the fish blocks (stored for 1–5 days at 4 °C) were taken from the refrigerator randomly and steamed for a certain time (0, 15, 30, 60, 90, 120 and 300 s, respectively). After being cooled with liquid nitrogen, the fish blocks were stored at 4 °C for further tests, including TPA, cooking loss, shear force and stress relaxation.
Quality indicators of mandarin fish
TPA
Figure 4 shows the TPA characteristics of fish muscle during the heating process after 1–5 days of storage. As depicted in Fig. 4a-c, the hardness, adhesiveness and chewiness of fish muscle exhibited similar decreasing trends as the heating time was extended, and it performed better with a shorter storage time due to the relatively intact sarcomere structure. For the fish muscle stored for 1 day, relatively high values of these indicators were demonstrated when cooked for 30 s. The reason may be that the surface of fish muscle became soft as the cooking time increased, yet its internal temperature was still actually low. In addition, the cohesiveness and springiness of fish muscle are illustrated in Fig. 4d-e, showing an upward trend with the increase of the heating time. However, there was no obvious difference observed in the fish muscle with different storage times.
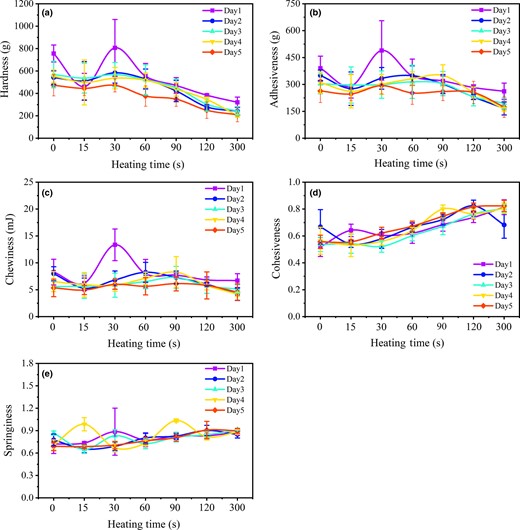
TPA indicators, including hardness (a), adhesiveness (b), chewiness (c), cohesiveness (d) and springiness (e) of mandarin fish during cooking with different storage time (1–5 days).
Cooking loss and shear force
As depicted in Fig. 3(B)a, the cooking loss of fish muscle gradually increased with prolonged storage time during cooking, and the fish muscle stored for 5 days showed the highest cooking loss, principally because the degree of shrinkage of myofibrils was positively correlated with the storage time (Straadt et al., 2007). Moreover, the heating process would endow a relatively loose protein structure due to the degradation of cytoskeletal proteins, thus leading to a decreased moisture retention capacity (Ovissipour et al., 2017).
Shear force is an important indicator for evaluating the tenderness of fish products, and the lower the shear force of fish muscle, the better the tenderness. As depicted in Fig. 3(B)b, the shear force of fish muscle generally declined during cooking, indicating the positive effect of cooking on improvement in the tenderness. For uncooked muscles, the shear force decreased within 3 days of storage, suggesting that the fish muscle became tenderer with prolonged storage time, mainly due to that its sarcomere length decreased with 1–3 days storage and the degradation of large structural proteins (titin and nebulin). As the storage time was extended further, the shear force increased, corresponding to an increase in sarcomere length. However, the shear force of fish muscle decreased as the cooking time increased, which was related to the protein unfolding and aggregation. The shear force of fish muscle stored for 5 days declined suddenly when cooked over 90 s, implying poor protein network formation due to the structural proteins degradation.
Stress relaxation
The stress-relaxation experiment was carried out to study the rheological properties of mandarin fish during cooking, by which the structural composition and viscoelastic state of samples were reflected. Based on the stress curves of fish muscle (Fig. 5), the generalised Maxwell model was used to describe the stress-relaxation property of fish muscle, which contained a spring connected in parallel with several Maxwell models, and can be expressed by eq. 1 (Herrero & Careche, 2005).
where, σ(t) represents the stress at time t during stress relaxation; t is the stress-relaxation test time; ε0 is the compressive deformation, ε0 = 30%; E0 is the equilibrium elastic modulus; Ei is the decay modulus of elasticity; τi is the relaxation time; and ηi is the damping coefficient of damper and ηi = Ei × τi.

The left panel depicts the stress-relaxation curve of uncooked sample at day 1, where a represents compression phase, b represents stress-relaxation phase and c represents expansion phase, the inner image represents the stress-relaxation phase (selection of function). And the right panel shows the generalised Maxwell model.
The original test of the stress-relaxation curve was performed with a three-element model (one spring in parallel with one Maxwell model), a five-element model (one spring in parallel with two Maxwell models) or a seven-element model (one spring in parallel with three Maxwell models), respectively, and the corresponding data were subjected to nonlinear regression fitting (Myhan et al., 2015). The coefficient (R2) was in the range of 0.951–0.988 for the three-element model, 0.995–0.999 for the five-element model and 0.998–0.999 for the seven-element model. The five-element and seven-element models fitted relatively better than three-element model, indicating the stress-relaxation phenomenon of mandarin fish followed these two models well. In order to simplify the data, the five-element model was selected to describe the stress-relaxation phenomenon of mandarin fish, and one of the experimental fitted curves is shown in Fig. 5.
The relaxation time τ is an essential parameter in the stress-relaxation model, which is associated with the protein–protein interactions and protein degradation. The longer the relaxation time, the greater the intermolecular adhesion of the myofibrillar proteins, indicating that they require a longer time to slide past each other. There are two relaxation times in the Maxwell five-element model, reflecting the collective variation of the relaxation characteristics (see Table S2). τ1 showed a decreasing trend initially, then increased and finally declined, and τ2 decreased initially and then increased.
In order to clearly reflect the viscoelasticity of fish muscle during the heating process, the obtained stress-relaxation parameters were repeatedly compared and analysed. Ultimately, τ (τ1/τ2) was selected to describe the variation law. As can be seen from Fig. 6(a), the τ value of uncooked muscles significantly increased within 2 days storage, which was probably ascribed to that the interactions between the myofibrillar proteins. According to the report of Sun et al. (2018a), the main change in the interactions between the myofibrillar proteins was the increase of hydrophobic interactions during post-mortem storage, resulting in the aggregation of myofibrillar proteins. Therefore, the τ value increased. When stored over 2 days, the structural proteins scaffolding for the myofibril filaments were degraded, which would cause the decrease of τ value, but the τ value was almost unchanged, principally due to the increase of the interactions between myofibril proteins balanced this change.
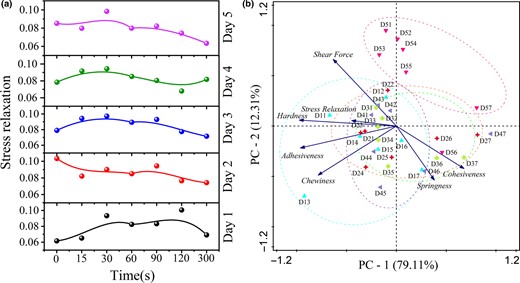
(a) Changes in stress-relaxation time during cooking with different storage time (1–5 days). (b) PCA of the TPA, shear force and stress relaxation of fish muscle. Blue icons: fish muscle stored for 1 day (cooking for 0, 15, 30, 60, 90, 120, 300 s, which corresponding to D11–D17). Red icons, green icons, purple icons and pink icons respectively represented the fish muscle stored for 2–5 days.
During the heating process, a peak appeared in 30 s at 1–5 days, which may be related to the test parameters. The average thickness of the fish blocks was 10 mm, and they were pressed down 3 mm by the probe. Hence, the τ value may mainly represent the protein–protein interactions of the surface of the fish blocks. The peak value indicated that the protein–protein interactions were enhanced during cooking, probably due to the formation of disulphide bonds in the surface of the fish blocks (Jia et al., 2019).
Correlation between quality indicators and protein degradation
PCA was performed on the quality indicators (TPA, shear force and stress relaxation) to obtain an overview of the results (Fig. 6b). The cumulative contribution rate of the two principal components reached 74.1%, and the eigen values were all greater than 1. Thus, the quality characteristics of mandarin fish muscle could be explained well by the first and second principal components. The first principal component (PC-1) mainly reflected the information of the chewiness, adhesiveness, hardness, stress and shear force, which could be used to describe the palatability of fish muscle. The second principal component (PC-2) mainly manifested the cohesiveness and springiness information, which could be used to describe the internal structural characteristics of fish muscle.
The correlation analysis results between the degradation of structural proteins and the variations of quality characteristics are shown in Table S3. For uncooked muscles, titin and nebulin showed a positive correlation with the chewiness and adhesiveness (R> 0.75), denoting that the degradation of titin and nebulin primarily impacted PC-1. Likewise, desmin was correlated with the hardness (P < 0.01) and adhesiveness (P < 0.05), and it also influenced PC-1. TPM was significantly correlated with the springiness (P < 0.01), whose degradation mostly affected PC-2.
For cooked muscles, titin was negatively correlated with the cohesiveness (P < 0.01) and springiness (R> 0.80), suggesting that the degradation of titin contributed to the improvement of the cohesiveness and springiness of fish muscle during the early stage of storage (1–2 days). This indicated that the degradation of titin primarily affected PC-2. However, nebulin was positively correlated with the hardness (P < 0.01), adhesiveness (P < 0.05) and chewiness (P < 0.05), implying that its degradation mainly affected PC-1. Hence, the palatability and internal structural characteristics of cooked fish muscle were mainly influenced by the degradation of titin and nebulin.
Based on the results of Western blotting, the structural proteins of the uncooked muscles, including titin, nebulin and desmin, were degraded with a prolonged storage time, while TPM began to degrade during the later stage of storage (4–5 days). Thus, it can be concluded that the palatability of uncooked fish muscle was mainly affected by the degradation of titin, nebulin and desmin during the early stage of storage (1–3 days), and the internal structural characteristics of uncooked muscles were influenced by TPM degradation during the later stage of storage (4–5 days). However, for the cooked muscles, the band of titin was undetectable after 2 days post-mortem, and nebulin was degraded completely after 3 days post-mortem. Therefore, the palatability and internal structural characteristics of cooked fish muscle were mainly influenced by the degradation of titin and nebulin during the early stage of storage (1–3 days).
Conclusion
During the post-mortem storage of mandarin fish, titin was degraded initially, accompanied with an increase of the I-band length. Subsequently, the degradation of desmin induced a gradual breakage of the myofibrils. The degradation of nebulin that followed accelerated the destruction of N2-lines, which occurred with a slightly fuzzy Z-disc. Finally, TPM began to degrade, and the remained desmin was also further degraded. Furthermore, the quality of fish muscle was highly related to the structural proteins. The degradation of titin, nebulin and desmin in uncooked muscles primarily impacted the palatability during the early stage of storage (1–3 days), and the degradation of TPM in uncooked fish muscle influenced the internal structural characteristics during the later stage of storage (4–5 days). However, the palatability and internal structural characteristics of cooked fish muscle were mainly influenced by the degradation of titin and nebulin during the early stage of storage (1–3 days). Hence, these structural proteins could be used as biomarkers to monitor the quality of fish muscle during post-mortem storage and cooking.
Acknowledgments
This work was supported by the National Key R&D Program of China (No. 2016YFD0400203), National Natural Science Foundation of China (No. 31671881 & No. 31901683), Fundamental Research Funds for the Central Universities (XDJK2019B028), Chongqing Research Program of Basic Research and Frontier Technology (No. cstc2018jcyjA0939).
Conflict of interest
The authors have no conflict of interest to disclose.
Data availability statement
Research data are not shared.
Ethical guidelines
Ethics approval was not required for this research.