-
PDF
- Split View
-
Views
-
Cite
Cite
Marco Brambilla, Francesca Martani, Paola Branduardi, The recruitment of the Saccharomyces cerevisiae poly(A)-binding protein into stress granules: new insights into the contribution of the different protein domains, FEMS Yeast Research, Volume 17, Issue 6, September 2017, fox059, https://doi.org/10.1093/femsyr/fox059
- Share Icon Share
Abstract
The Saccharomyces cerevisiae poly(A)-binding protein Pab1 is a modular protein composed of four RNA recognition motifs (RRM), a proline-rich domain (P) and a C-terminus. Thanks to this modularity, Pab1 is involved in different interactions that regulate many aspects of mRNA metabolism, including the assembly of stress granules. In this work, we analyzed the contribution of each domain for the recruitment of the protein within stress granules by comparing the intracellular distribution of synthetic Pab1-GFP variants, lacking one or more domains, with the localization of the endogenous mCherry-tagged Pab1. Glucose starvation and heat shock were used to trigger the formation of stress granules. We found that Pab1 association into these aggregates relies mainly on RRMs, whose number is important for an efficient recruitment of the protein. Interestingly, although the P and C domains do not directly participate in Pab1 association to stress granules, their presence strengthens or decreases, respectively, the distribution of synthetic Pab1 lacking at least one RRM into these aggregates. In addition to describing the contribution of domains in determining Pab1 association within stress granules, the outcomes of this study suggest the modularity of Pab1 as an attractive platform for synthetic biology approaches aimed at rewiring mRNA metabolism.
INTRODUCTION
Poly(A)-binding proteins (generically termed PABPs) are ubiquitous and conserved among Eukarya, where they are involved in almost all mRNAs’ post-transcriptional events (Eliseeva, Lyabin and Ovchinnikov 2013; Smith, Blee and Gray 2014). Multiple PABPs can be found in higher eukaryotes: in humans and in Arabidopsis thaliana, there are up to five and eight PABPs, respectively, while single-celled eukaryotes have just one (Goss and Kleiman 2013). Apart from some specialized PABPs (Eliseeva, Lyabin and Ovchinnikov 2013; Smith, Blee and Gray 2014), their structure is typically highly conserved. These modular proteins are composed of four RNA recognition motifs (RRMs) for poly(A) tail binding that are linked to a carboxy-terminal domain (C domain) through a flexible linker rich in proline (for this reason named P domain), methionine, glycine, glutamine and asparagine (Yao et al.2007; Richardson et al.2012). The structure of RRMs (∼90–100 residues) is typically highly conserved from yeast to higher eukaryotes: these globular domains consist of four antiparallel β-sheets flanked by two α-helices, with two highly conserved sequences (RNP-1 and RNP-2) in the two central β-sheets that are involved in poly(A) tail binding (Eliseeva, Lyabin and Ovchinnikov 2013; Smith, Blee and Gray 2014). Differently from RRMs, the P domain is intrinsically disordered and it is significantly divergent in length across different species (Riback et al.2017). In particular, the alignment of the P domain among closely related species revealed that many sites are perfectly conserved, while medium- and long-chain aliphatic residues often substitute from each other (Riback et al.2017). Finally, the C-terminal domain is globular with ∼75 residues that fold into four or five α-helices depending on the species (Smith, Blee and Gray 2014).
The PABP of Saccharomyces cerevisiae (named Pab1) has been extensively studied over the last decades to describe its role in controlling mRNA metabolism. It has been demonstrated that Pab1 binding to the poly(A) tail protects transcripts from degradation by inhibiting the deadenylase activity of the Ccr4/Pop2/Not complex (Yao et al.2007; Zhang et al.2013). On the contrary, Pab1 stimulates the activity of the minor deadenylation complex Pan2/Pan3 by recruiting it, through its C-terminal domain, to the poly(A) tail of mRNAs (Simón and Séraphin 2007; Wahle and Winkler 2013). The dissociation of Pab1 from the poly(A) tail, which is responsible for Ccr4 deadenylation, is induced by protein self-association, a process occurring through the formation of circular species and/or oligomers (Yao et al.2007). The circularization of Pab1 is likely accomplished by the interaction of the RRM1 with the RRM4 and the P domain, whereas the structure of Pab1 oligomers is less clear (Yao et al.2007). Furthermore, Pab1 is implicated in the control of translation initiation and termination. In fact, by binding eIF4G through the RRM1 and RRM2 domains, it allows the formation of a closed-loop structure of the mRNA that stimulates translation initiation (Richardson et al.2012; Melamed et al.2015). On the contrary, Pab1 negatively regulates translation termination by interacting, through its P and C domains, with the releasing factor eRF3 (Sup35); such interaction allows a basal level of translational readthrough (Roque et al.2015). Finally, Pab1 is involved in mRNA export from nucleus to cytoplasm through the Mex67/mRNA-dependent pathway. To exert this function, Pab1 enters into the nucleus through the interaction between its nuclear localization signal, localized in the RRM4 domain, and the importin Kap108/Sxm1 (Brune et al.2005; Richardson et al.2012). A second route through which Pab1 can be exported to the cytoplasm occurs through the Xpo1/Crm1-dependent pathway (Brune et al.2005; Dunn et al.2005).
Pab1 has also an important role in promoting the assembly of stress granules, of which it is a component but is not indispensable (Swisher and Parker 2010). Stress granules are cytoplasmic aggregates of untranslated mRNPs that form when translation initiation is impaired, such as when cells encounter stressful conditions (Buchan and Parker 2009; Protter and Parker 2016). They contain, among others, factors involved in translation initiation, such as the 40S ribosomal subunit and eIF4G; therefore, they are thought to be sites for mRNA translation re-initiation (Buchan and Parker 2009). However, depending on the stress, their composition can be slightly variable (Buchan and Parker 2009; Grousl et al.2009; Buchan, Yoon and Parker 2011; Giménez-Barcons and Díez 2011; Decker and Parker 2012). When not translated, mRNAs can be cleared by vacuolar autophagy together with the entire stress granule in which they are stored, or can be exchanged with processing bodies (P-bodies) where they are principally degraded (Buchan and Parker 2009; Buchan et al.2013; Walters and Parker 2015; Protter and Parker 2016). However, mRNAs in P-bodies can also return to stress granules and/or to translation (Buchan and Parker 2009; Buchan et al.2013; Walters and Parker 2015; Protter and Parker 2016). It has been proposed that Pab1 role in stress granules formation might be to promote the transition of polyadenylated mRNAs from P-bodies to stress granules. In fact, PAB1 deletion in the bypass suppressor spb2Δ strain reduced the number of stress granules and increased the number of P-bodies. However, being Pab1 an essential protein, the need for suppressors limits the possibility to accurately define its role in stress granules formation (Swisher and Parker 2010).
As described above, the domains of Pab1 are responsible for its multiple functions because of their specific interactions with different proteins involved in the regulation of mRNA metabolism. However, to the best of our knowledge little is known about their function in Pab1 recruitment within stress granules. In this work, we aimed to understand the contribution of each Pab1 domain in protein recruitment within stress granules. For this purpose, we created S. cerevisiae strains containing both the endogenous Pab1 tagged with mCherry, as a marker of stress granules, and different GFP-tagged versions of the protein, lacking one or more domains. The intracellular distribution and the propensity of Pab1 variants to localize within stress granules, evoked by glucose deprivation or heat shock, were then determined by fluorescent microscopy observations.
MATERIAL AND METHODS
Strains and plasmids construction
The Saccharomyces cerevisiae genetic background used in this study was CEN.PK113–11C (MATa; MAL2–8c; SUC2; ura3–52; his3Δ1). The S. cerevisiae strains created and used in this study are listed in Table 1.
Strains . | Relevant genotype . | Source . |
---|---|---|
CEN.PK113–11C | MATa; MAL2–8c; SUC2; ura3–52; his3Δ1 (reference strain) | Peter Kötter, Frankfurt, Germany |
PAB1-mCherry | CEN.PK113–11C; PAB1::PAB1-mCherry-HIS3 | This study |
R1-C | PAB1-mCherry [pYX012(-ATG)RRM1-C-GFP] | This study |
R2-C | PAB1-mCherry [pYX012(-ATG)RRM2-C-GFP] | This study |
R3-C | PAB1-mCherry [pYX012(-ATG)RRM3-C-GFP] | This study |
R4-C | PAB1-mCherry [pYX012(-ATG)RRM4-C-GFP] | This study |
P-C | PAB1-mCherry [pYX012(-ATG)P-C-GFP] | This study |
C | PAB1-mCherry [pYX012(-ATG)C-GFP] | This study |
R1-P | PAB1-mCherry [pYX012(-ATG)RRM1-P-GFP] | This study |
R2-P | PAB1-mCherry [pYX012(-ATG)RRM2-P-GFP] | This study |
R3-P | PAB1-mCherry [pYX012(-ATG)RRM3-P-GFP] | This study |
R4-P | PAB1-mCherry [pYX012(-ATG)RRM4-P-GFP] | This study |
P | PAB1-mCherry [pYX012(-ATG)P-GFP] | This study |
R1-R4 | PAB1-mCherry [pYX012(-ATG)RRM1-RRM4-GFP] | This study |
R2-R4 | PAB1-mCherry [pYX012(-ATG)RRM2-RRM4-GFP] | This study |
R3-R4 | PAB1-mCherry [pYX012(-ATG)RRM3-RRM4-GFP] | This study |
R4 | PAB1-mCherry [pYX012(-ATG)RRM4-GFP] | This study |
Strains . | Relevant genotype . | Source . |
---|---|---|
CEN.PK113–11C | MATa; MAL2–8c; SUC2; ura3–52; his3Δ1 (reference strain) | Peter Kötter, Frankfurt, Germany |
PAB1-mCherry | CEN.PK113–11C; PAB1::PAB1-mCherry-HIS3 | This study |
R1-C | PAB1-mCherry [pYX012(-ATG)RRM1-C-GFP] | This study |
R2-C | PAB1-mCherry [pYX012(-ATG)RRM2-C-GFP] | This study |
R3-C | PAB1-mCherry [pYX012(-ATG)RRM3-C-GFP] | This study |
R4-C | PAB1-mCherry [pYX012(-ATG)RRM4-C-GFP] | This study |
P-C | PAB1-mCherry [pYX012(-ATG)P-C-GFP] | This study |
C | PAB1-mCherry [pYX012(-ATG)C-GFP] | This study |
R1-P | PAB1-mCherry [pYX012(-ATG)RRM1-P-GFP] | This study |
R2-P | PAB1-mCherry [pYX012(-ATG)RRM2-P-GFP] | This study |
R3-P | PAB1-mCherry [pYX012(-ATG)RRM3-P-GFP] | This study |
R4-P | PAB1-mCherry [pYX012(-ATG)RRM4-P-GFP] | This study |
P | PAB1-mCherry [pYX012(-ATG)P-GFP] | This study |
R1-R4 | PAB1-mCherry [pYX012(-ATG)RRM1-RRM4-GFP] | This study |
R2-R4 | PAB1-mCherry [pYX012(-ATG)RRM2-RRM4-GFP] | This study |
R3-R4 | PAB1-mCherry [pYX012(-ATG)RRM3-RRM4-GFP] | This study |
R4 | PAB1-mCherry [pYX012(-ATG)RRM4-GFP] | This study |
Strains . | Relevant genotype . | Source . |
---|---|---|
CEN.PK113–11C | MATa; MAL2–8c; SUC2; ura3–52; his3Δ1 (reference strain) | Peter Kötter, Frankfurt, Germany |
PAB1-mCherry | CEN.PK113–11C; PAB1::PAB1-mCherry-HIS3 | This study |
R1-C | PAB1-mCherry [pYX012(-ATG)RRM1-C-GFP] | This study |
R2-C | PAB1-mCherry [pYX012(-ATG)RRM2-C-GFP] | This study |
R3-C | PAB1-mCherry [pYX012(-ATG)RRM3-C-GFP] | This study |
R4-C | PAB1-mCherry [pYX012(-ATG)RRM4-C-GFP] | This study |
P-C | PAB1-mCherry [pYX012(-ATG)P-C-GFP] | This study |
C | PAB1-mCherry [pYX012(-ATG)C-GFP] | This study |
R1-P | PAB1-mCherry [pYX012(-ATG)RRM1-P-GFP] | This study |
R2-P | PAB1-mCherry [pYX012(-ATG)RRM2-P-GFP] | This study |
R3-P | PAB1-mCherry [pYX012(-ATG)RRM3-P-GFP] | This study |
R4-P | PAB1-mCherry [pYX012(-ATG)RRM4-P-GFP] | This study |
P | PAB1-mCherry [pYX012(-ATG)P-GFP] | This study |
R1-R4 | PAB1-mCherry [pYX012(-ATG)RRM1-RRM4-GFP] | This study |
R2-R4 | PAB1-mCherry [pYX012(-ATG)RRM2-RRM4-GFP] | This study |
R3-R4 | PAB1-mCherry [pYX012(-ATG)RRM3-RRM4-GFP] | This study |
R4 | PAB1-mCherry [pYX012(-ATG)RRM4-GFP] | This study |
Strains . | Relevant genotype . | Source . |
---|---|---|
CEN.PK113–11C | MATa; MAL2–8c; SUC2; ura3–52; his3Δ1 (reference strain) | Peter Kötter, Frankfurt, Germany |
PAB1-mCherry | CEN.PK113–11C; PAB1::PAB1-mCherry-HIS3 | This study |
R1-C | PAB1-mCherry [pYX012(-ATG)RRM1-C-GFP] | This study |
R2-C | PAB1-mCherry [pYX012(-ATG)RRM2-C-GFP] | This study |
R3-C | PAB1-mCherry [pYX012(-ATG)RRM3-C-GFP] | This study |
R4-C | PAB1-mCherry [pYX012(-ATG)RRM4-C-GFP] | This study |
P-C | PAB1-mCherry [pYX012(-ATG)P-C-GFP] | This study |
C | PAB1-mCherry [pYX012(-ATG)C-GFP] | This study |
R1-P | PAB1-mCherry [pYX012(-ATG)RRM1-P-GFP] | This study |
R2-P | PAB1-mCherry [pYX012(-ATG)RRM2-P-GFP] | This study |
R3-P | PAB1-mCherry [pYX012(-ATG)RRM3-P-GFP] | This study |
R4-P | PAB1-mCherry [pYX012(-ATG)RRM4-P-GFP] | This study |
P | PAB1-mCherry [pYX012(-ATG)P-GFP] | This study |
R1-R4 | PAB1-mCherry [pYX012(-ATG)RRM1-RRM4-GFP] | This study |
R2-R4 | PAB1-mCherry [pYX012(-ATG)RRM2-RRM4-GFP] | This study |
R3-R4 | PAB1-mCherry [pYX012(-ATG)RRM3-RRM4-GFP] | This study |
R4 | PAB1-mCherry [pYX012(-ATG)RRM4-GFP] | This study |
To fuse the genomic PAB1 gene with mCherry, the reference strain was transformed with a fragment containing homologous flanking for PAB1, a 21-bp linker, the mCherry sequence, the 3΄UTR of PGK1 and the HIS3 auxotrophic. This fragment was PCR amplified with primers Pab1-mCh-FW and Pab1-mCh-RV from pYX022-mCherry vector that was previously constructed as follows: a 934-bp fragment that comprised the linker, the mCherry sequence and the 3΄UTR of PGK1 was PCR amplified with primers mCh-FW and mCh-RV from the vector YCplac33 EDC3-mCherry (pRP1657), kindly provided by Prof. Parker (Buchan, Muhlrad and Parker 2008). The fragment was subsequently XmaI and XhoI cloned into pYX022 plasmid (R&S system, Wiesbaden, Germany) to obtain the desired pYX022-mCherry vector.
The DNA sequences encoding for Pab1-GFP truncated versions were PCR amplified from YCplac33 PAB1-GFP (Martani et al.2015) and cloned under the control of the TPI promoter into the pYX012(-ATG) plasmid, which was created by digesting with EcoRI and BamHI, blunt-ending and re-ligating the pYX012 (R&S system, Wiesbaden, Germany). More in detail, the sequences encoding for all the synthetic variants carrying the C domain fused with GFP were PCR amplified using GFP-TER-RV as reverse primer and RRM1-TER-FW, RRM2-TER-FW, RRM3-TER-FW, RRM4-TER-FW, PC-TER-FW and C-TER-FW-NEW as forward primers. The corresponding fragments were ApaI/SalI cloned into pYX012(-ATG) to obtain vectors pYX012(-ATG) RRM1-C-GFP, pYX012(-ATG) RRM2-C-GFP, pYX012(-ATG) RRM3-C-GFP, pYX012(-ATG) RRM4-C-GFP, pYX012(-ATG) P-C-GFP and pYX012(-ATG) C-GFP.
Fragments lacking the sequence encoding for the C domain were PCR amplified using P-BamHI-RV as reverse primer and RRM1-TER-FW, RRM2-TER-FW, RRM3-TER-FW, RRM4-TER-FW and PC-TER-FW as forward primers. Fragments lacking the sequence encoding for the P and the C domains were PCR amplified using RRM4-BamHI-RV as reverse primer and RRM1-TER-FW, RRM2-TER-FW, RRM3-TER-FW and RRM4-TER-FW as forward primers. The corresponding fragments were cloned into pYX012(-ATG) RRM1-C-GFP plasmid (previously ApaI/BamHI digested to remove the RRM1-C sequence and to maintain the GFP) to obtain vectors pYX012(-ATG) RRM1-P-GFP, pYX012(-ATG) RRM2-P-GFP, pYX012(-ATG) RRM3-P-GFP, pYX012(-ATG) RRM4-P-GFP, pYX012(-ATG) P-GFP, pYX012(-ATG) RRM1-RRM4-GFP, pYX012(-ATG) RRM2-RRM4-GFP, pYX012(-ATG) RRM3-RRM4-GFP and pYX012(-ATG) RRM4-GFP.
The recombinant vectors were StuI linearized into the URA3 marker and used to transform the PAB1-mCherry strain. Transformation was performed with the LiAc/PEG/ss-DNA protocol (Gietz and Woods 2002). The primers used in this study are listed in Table 2.
Primer . | Sequence . |
---|---|
mCh-FW | CTCGAGCCCGGGTTAATTAACATG |
mCh-RV | TATGAACTCGAGCATAAAGGCATTAAAAGAGGAGCG |
Pab1-mCh-FW | GTCTTTCAAAAAGGAGCAAGAACAACAAACTGAGCAAGCTCGTCGACCCGGGTTAATTAAC |
Pab1-mCh-RV | TAAGTTTGTTGAGTAGGGAAGTAGGTGATTACATAGAGCACGTTTTAAGAGCTTGGTGAGC |
GFP-TER-RV | GGAAGCGTCGACTTATTTGTATAGTTCATCCATGCC |
RRM1-TER-FW | GTAAAAGGGCCCATGGCTGATATTACTGATAAG |
RRM2-TER-FW | GTAAAAGGGCCCATGGGTAACATCTTTATCAAGAACT |
RRM3-TER-FW | GTAAAAGGGCCCATGTACACTAACCTTTATGTGAAAA |
RRM4-TER-FW | GTAAAAGGGCCCATGAATTTGTTTGTTAAGAACTTAGATG |
PC-TER-FW | GTAAAAGGGCCCATGTTGGCTCAACAAATCCAAGC |
C-TER-FW | GTAAAAGGGCCCATGCAAAAGCAAAGACAAGCTTTG |
RRM4-BamHI-RV | GACTTTGGATCCGTTGAGAACGTCTTACGTC |
P-BamHI-RV | GACTGGGGATCCGTTGATAAAATTGGTTGTTATCG |
Primer . | Sequence . |
---|---|
mCh-FW | CTCGAGCCCGGGTTAATTAACATG |
mCh-RV | TATGAACTCGAGCATAAAGGCATTAAAAGAGGAGCG |
Pab1-mCh-FW | GTCTTTCAAAAAGGAGCAAGAACAACAAACTGAGCAAGCTCGTCGACCCGGGTTAATTAAC |
Pab1-mCh-RV | TAAGTTTGTTGAGTAGGGAAGTAGGTGATTACATAGAGCACGTTTTAAGAGCTTGGTGAGC |
GFP-TER-RV | GGAAGCGTCGACTTATTTGTATAGTTCATCCATGCC |
RRM1-TER-FW | GTAAAAGGGCCCATGGCTGATATTACTGATAAG |
RRM2-TER-FW | GTAAAAGGGCCCATGGGTAACATCTTTATCAAGAACT |
RRM3-TER-FW | GTAAAAGGGCCCATGTACACTAACCTTTATGTGAAAA |
RRM4-TER-FW | GTAAAAGGGCCCATGAATTTGTTTGTTAAGAACTTAGATG |
PC-TER-FW | GTAAAAGGGCCCATGTTGGCTCAACAAATCCAAGC |
C-TER-FW | GTAAAAGGGCCCATGCAAAAGCAAAGACAAGCTTTG |
RRM4-BamHI-RV | GACTTTGGATCCGTTGAGAACGTCTTACGTC |
P-BamHI-RV | GACTGGGGATCCGTTGATAAAATTGGTTGTTATCG |
CCCGGG: XmaI restriction site; CTCGAG: XhoI restriction site; GTCGAC: SalI restriction site; GGGCCC: ApaI restriction site; GGATCC: BamHI restriction site.
Primer . | Sequence . |
---|---|
mCh-FW | CTCGAGCCCGGGTTAATTAACATG |
mCh-RV | TATGAACTCGAGCATAAAGGCATTAAAAGAGGAGCG |
Pab1-mCh-FW | GTCTTTCAAAAAGGAGCAAGAACAACAAACTGAGCAAGCTCGTCGACCCGGGTTAATTAAC |
Pab1-mCh-RV | TAAGTTTGTTGAGTAGGGAAGTAGGTGATTACATAGAGCACGTTTTAAGAGCTTGGTGAGC |
GFP-TER-RV | GGAAGCGTCGACTTATTTGTATAGTTCATCCATGCC |
RRM1-TER-FW | GTAAAAGGGCCCATGGCTGATATTACTGATAAG |
RRM2-TER-FW | GTAAAAGGGCCCATGGGTAACATCTTTATCAAGAACT |
RRM3-TER-FW | GTAAAAGGGCCCATGTACACTAACCTTTATGTGAAAA |
RRM4-TER-FW | GTAAAAGGGCCCATGAATTTGTTTGTTAAGAACTTAGATG |
PC-TER-FW | GTAAAAGGGCCCATGTTGGCTCAACAAATCCAAGC |
C-TER-FW | GTAAAAGGGCCCATGCAAAAGCAAAGACAAGCTTTG |
RRM4-BamHI-RV | GACTTTGGATCCGTTGAGAACGTCTTACGTC |
P-BamHI-RV | GACTGGGGATCCGTTGATAAAATTGGTTGTTATCG |
Primer . | Sequence . |
---|---|
mCh-FW | CTCGAGCCCGGGTTAATTAACATG |
mCh-RV | TATGAACTCGAGCATAAAGGCATTAAAAGAGGAGCG |
Pab1-mCh-FW | GTCTTTCAAAAAGGAGCAAGAACAACAAACTGAGCAAGCTCGTCGACCCGGGTTAATTAAC |
Pab1-mCh-RV | TAAGTTTGTTGAGTAGGGAAGTAGGTGATTACATAGAGCACGTTTTAAGAGCTTGGTGAGC |
GFP-TER-RV | GGAAGCGTCGACTTATTTGTATAGTTCATCCATGCC |
RRM1-TER-FW | GTAAAAGGGCCCATGGCTGATATTACTGATAAG |
RRM2-TER-FW | GTAAAAGGGCCCATGGGTAACATCTTTATCAAGAACT |
RRM3-TER-FW | GTAAAAGGGCCCATGTACACTAACCTTTATGTGAAAA |
RRM4-TER-FW | GTAAAAGGGCCCATGAATTTGTTTGTTAAGAACTTAGATG |
PC-TER-FW | GTAAAAGGGCCCATGTTGGCTCAACAAATCCAAGC |
C-TER-FW | GTAAAAGGGCCCATGCAAAAGCAAAGACAAGCTTTG |
RRM4-BamHI-RV | GACTTTGGATCCGTTGAGAACGTCTTACGTC |
P-BamHI-RV | GACTGGGGATCCGTTGATAAAATTGGTTGTTATCG |
CCCGGG: XmaI restriction site; CTCGAG: XhoI restriction site; GTCGAC: SalI restriction site; GGGCCC: ApaI restriction site; GGATCC: BamHI restriction site.
Media and induction of stress granules
Strains were grown in shake flasks in Yeast Nitrogen Base (YNB, BD, Franklin Lakes, New Jersey, USA) minimal medium, with 2% glucose, until the exponential phase of growth (OD660nm = 0.3–0.6) prior to stress treatment. Heat-shocked cells were prepared as previously described (Grousl et al.2009): briefly, cell suspension was pelleted at 3000 rpm for 5 min, resuspended in pre-heated YNB minimal medium and then incubated at 46°C for 50 min at 160 rpm. Glucose-deprived cells were prepared as previously described (Buchan, Nissan and Parker 2010): briefly, 2 mL of cells were centrifuged for 30 s at 13 000 rpm, washed twice with YNB medium without glucose and incubated with the same medium at 30°C for 15 min at 160 rpm.
Protein extraction and western blot analysis
Cells were grown until the exponential phase (OD660nm 0.3–0.6) prior to protein extraction: cells were centrifuged at 3000 rpm for 5 min and resuspended in extraction buffer composed of 0.1 M potassium phosphate buffer (pH 7.5), 1 mM ethylenediaminetetraacetic acid, 1 mM dithiothreitol, 1 mM protease inhibitor cocktail and 1 mM phenylmethane sulfonyl fluoride. Cells were subjected to three cycles of mechanical disruption with the FastPrep-24 (MP Biomedical, Santa Ana, California, USA). Cellular lysates were centrifuged at 14 000 rpm for 20 min at 4°C. Supernatants were collected and protein concentration was estimated using bovine serum albumin as reference (Bradford 1976). Proteins were resuspended in Laemmli buffer, boiled 5 min and resolved in a SDS-PAGE. Western blot was performed using the primary antibodies anti-GFP antibody JL-8 (Clontech, Mountain View, California, USA) and anti-actin antibody C4 (Abcam, Cambridge, UK). Pierce Rabbit anti-mouse IgG (Fc) conjugated with alkaline phosphatase (ThermoScientific, Waltham, Massachusetts, USA) was used as secondary antibody. The membranes were developed adding CDP-Star Chemioluminescent Substrate (Sigma, Saint Louis, Missouri, USA) and then exposed to Pierce CL-Xposure film to reveal signals. Bands were quantified using ImageJ 1.47v software.
Fluorescence microscopy analyses
Cells were observed with Nikon Eclipse 90i microscope (Nikon, Minato, Tokyo, Japan) and images were acquired with Digital Sight DS-U3 Nikon camera using NIS-Elements software (version 4.3). To visualize nuclei, cells in exponential phase of growth were stained with DAPI as described in Forsburg and Rhind (2006) modified as follows: 200 μL of ethanol 70% was added to 1 mL of cells and incubated at room temperature for 5 min. Then cells were centrifuged at 6000 rpm for 2 min and resuspended in 100 μL of PBS with DAPI at a final concentration of 1 μg/mL. Quantitation of cells displaying both nuclear and cytosolic localization of the corresponding GFP-tagged version was blindly determined for each cell by merging images of the green and the blue channels. Then, the percentage ratio between the number of cells with dual localization and the total number of cells was calculated. Quantification of stress granules and protein aggregates was performed in a blind manner for three independent experiments. For each experiment, green and red fluorescent dots were counted in a variable number of cells between 60 and 100. For each cell, the ratio between the number of green and red dots was calculated and the overlap between green and red fluorescent dots was blindly determined by merging images of the green and red channels and looking for the resulting yellow dots; then, the ratio between the overlapped and the total number of dots was calculated. Finally, the mean and the standard deviation were calculated for all the parameters. Student's t-test was used for evaluating significant differences between strains.
RESULTS
Contribution of RRM domains in the intracellular localization of Pab1
To examine the contribution of protein domains in the intracellular distribution of Pab1 and its association within stress granules, we analyzed the in vivo localization of Pab1 mutants in which the domains, starting from the RRM1, were sequentially removed (Fig. 1A). For this purpose, these protein versions were tagged with GFP and their localization was juxtaposed and compared to that of the endogenous Pab1 tagged with mCherry, used as a marker of stress granules (Buchan, Nissan and Parker 2010; Swisher and Parker 2010; Wheeler et al.2016). To ensure high expression levels, the constitutive TPI promoter was chosen for the overexpression of each variant.
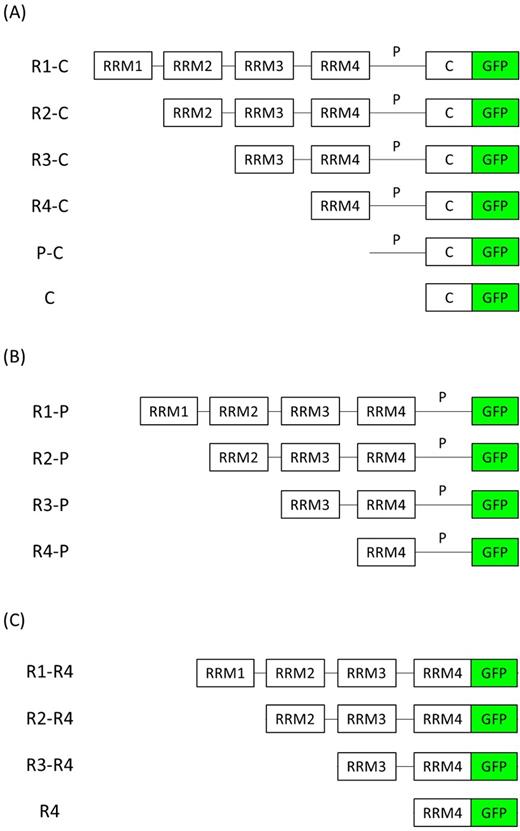
List of GFP-tagged Pab1 variants. Schematic representation of Pab1 variants (A) R1-C, R2-C, R3-C, R4-C, P-C and C, (B) R1-P, R2-P, R3-P and R4-P, (C) R1-R4, R2-R4, R3-R4 and R4. RRM: RNA recognition motifs, P: P-domain, C: C-domain, GFP: green fluorescent protein. The DNA constructs encoding for each Pab1 variant were constitutively overexpressed with the TPI promoter.
First, exponentially growing cells were analyzed to detect whether the absence of one or more domains could influence Pab1 localization under unstressed conditions. As shown in Fig. 2A, the full-length R1-C version, corresponding to the wild-type protein, overlapped completely with the endogenous Pab1 and localized predominantly in the cytoplasm as diffused protein, while almost no signal could be detected in the nucleus (see Table S1, Supporting Information). On the contrary, cells carrying the N-truncated versions also displayed green fluorescence in the nucleus; remarkably, this phenomenon was more pronounced for the R3-C variant (Fig. 2A; Table S1).
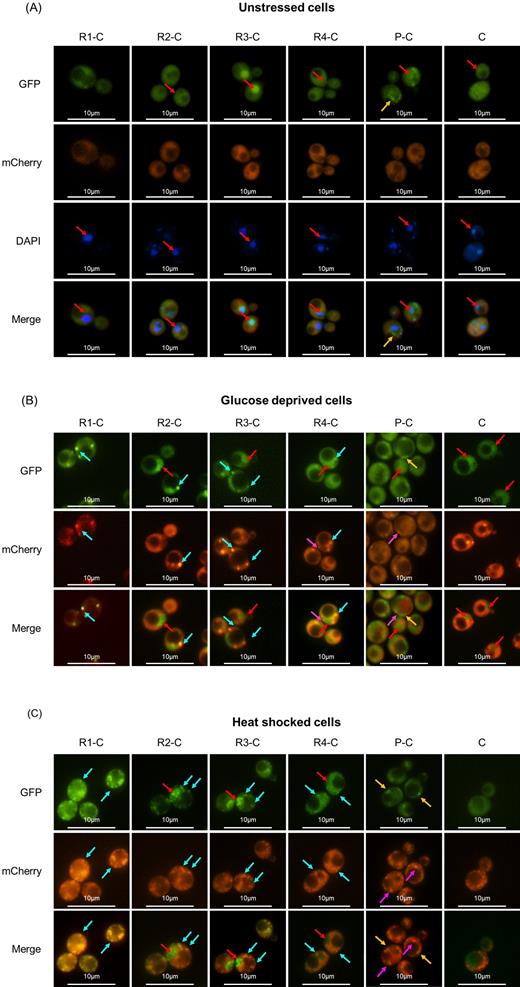
Effect of the removal of RRM on the localization of Pab1 in the absence and presence of stress. Intracellular localization of Pab1-mCherry and GFP-tagged Pab1 variants shown in Fig. 1A. Cells were grown until the exponential phase of growth (OD660nm = 0.3–0.6) and then (A) stained with DAPI to a final concentration of 1 μg/mL or (B) glucose starved for 15 min or (C) heat shocked for 50 min. Light blue arrows indicate co-localized green and red foci; fuchsia arrows indicate Pab1-mCherry stress granules without a co-localizing counterpart in the Pab1-GFP variant; yellow arrows indicate aggregates of the corresponding Pab1-GFP variant; red arrows indicate nuclei.
As previously reported, stress granules resulting from different type of stress display a different assembly and composition (Buchan, Yoon and Parker 2011). Therefore, we evoked stress granules by glucose starvation or heat shock to reinforce general observations and underline possible peculiarities. By scoring protein aggregates related to the wild-type Pab1 (red fluorescent dots) and the mutant versions (green fluorescent dots), we evaluated (i) the percentage of cells with green fluorescent dots; (ii) the mutant/wild-type dots ratio, as an index of the propensity of synthetic versions to aggregate similarly as the endogenous Pab1; (iii) the overlap between green and red fluorescent dots to evaluate the localization of Pab1 variants within stress granules.
Under stressful conditions, cells overexpressing the full-length protein displayed both red and green fluorescent foci that completely overlaid, indicating that the R1-C protein resides in stress granules (Fig. 2B and C; Fig. 3A; Table S2, Supporting Information). Similarly, the cytoplasmic fraction of all the protein variants carrying at least one RRM almost fully co-localized within stress granules (Fig. 2B and C; Fig. 3A; Table S2). However, in glucose-starved cells, the propensity to aggregate into stress granules generally decreased by lowering the number of RRM domains: in fact, R2-C, R3-C and R4-C variants displayed fewer protein aggregates compared to the endogenous stress granules, as observed by the mutant/wild-type dots ratio of 0.90, 0.67 and 0.68, respectively (Fig. 2B and 3B; Table S3, Supporting Information). A similar but less pronounced effect was observed in heat-shocked cells (Fig. 2C and 3B; Table S3). Although we cannot exclude a direct correlation between mutant/wild-type dots ratio and mutant protein levels, in stressful conditions the R4-C showed the highest expression level (Fig. S1 and Table S4, Supporting Information), but a low mutant/wild-type dots ratio. Therefore, it is likely that, at least for these variants, the different expression levels do not influence the propensity of the synthetic versions to aggregate into stress granules.
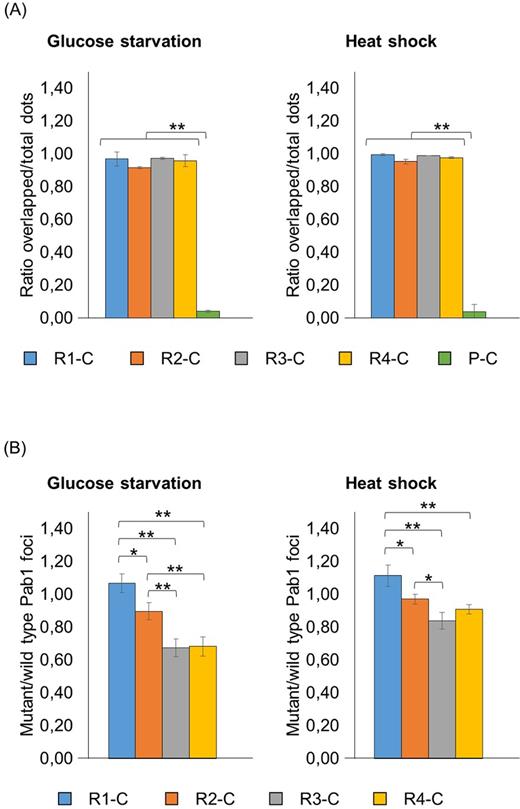
Analysis of the recruitment into stress granules of Pab1 variants carrying the C-terminal domain. Strains carrying the GFP-tagged Pab1 variants shown in Fig. 1A were grown until the exponential phase of growth (OD660nm = 0.3–0.6) and then subjected to glucose starvation for 15 min or heat shock for 50 min. Data in panel (A) show the ratio between the overlapped green-red fluorescent dots and the total number of dots. Data in panel (B) show the ratio between the number of mutant (green) and the number of wild type (red) foci. The variant C was not included in the histograms because no aggregates were detected in the corresponding strain, while the variant P-C was not included in panel (B) because the corresponding aggregates did not co-localize with endogenous stress granules. Values indicate the mean and the standard deviation of three independent experiments. Significance was calculated with two-tailed student t-test (*P ≤ 0.05; **P ≤ 0.01; see Table S6).
Both in the absence and in the presence of stress, in more than 90% of the cells the P-C variant displayed protein aggregates that did not co-localize with stress granules (Fig. 2 and 3A; Table S2). This behavior was likely related to the P domain because no aggregates were detected when the C domain was present alone, independently from a possible imposed stressful condition (Fig. 2).
These data suggest that the presence of at least one RRM is indispensable to allow a specific recruitment of Pab1 within stress granules, and that the presence of all four RRM domains seems to be required to direct its efficient inclusion into these aggregates. Furthermore, in the absence of the four RRMs, the C terminal domain seems not directly involved in protein localization into stress granules; however, its presence might influence the association of Pab1 within stress granules.
Cellular localization of Pab1 variants lacking the C-terminal domain
To evaluate the contribution of the C domain in Pab1 localization and recruitment within stress granules, we analyzed the intracellular localization of the previously described versions lacking the C-terminus (Fig. 1B). The P domain alone was not detected by western blot analysis indicating that it may not be translated or it may undergo degradation (data not shown). In the absence of stress, the localization of all these variants was as the one observed in the presence of the C domain (see Fig. S2A, Supporting Information).
In glucose-starved or heat-shocked cells, the green fluorescence associated to the R1-P, R2-P, R3-P and R4-P variants almost completely overlapped with the red fluorescent aggregates of the wild-type Pab1 (Fig. 4A; Fig. S2B and C; Table S2). Thus, the protein could localize into stress granules even in the absence of the C domain. However, the mutant/wild-type dots ratio of these variants was slightly higher than the one measured in cells containing the R2-C, R3-C and R4-C versions (Fig. 4B; Table S3). These results suggest that the C domain may have a negative effect on the Pab1 recruitment within stress granules in the absence of one or more RRM domains, even though we cannot exclude that the observation could be affected by different expression levels of the variants under stressful conditions (see Fig. S1 and Table S4).
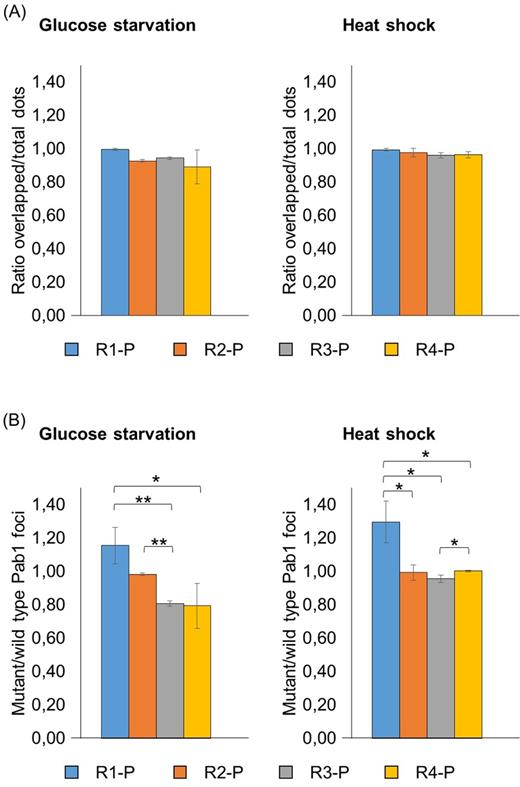
Analysis of the recruitment into stress granules of Pab1 variants lacking the C-terminal domain. Strains carrying the GFP-tagged Pab1 variants shown in Fig. 1B were grown until the exponential phase of growth (OD660nm = 0.3–0.6) and then subjected to glucose starvation for 15 min or heat shock for 50 min. Data in panel (A) show the ratio between the overlapped green-red fluorescent dots and the total number of dots. Data in panel (B) show the ratio between the number of mutant (green) and the number of wild type (red) foci. Values indicate the mean and the standard deviation of three independent experiments. Significance was calculated with two-tailed student t-test (*P ≤ 0.05; **P ≤ 0.01; see Table S6).
Cellular localization of Pab1 variants lacking the P and C domains
The P domain has a prominent role in Pab1 self-aggregation, a process responsible for favoring mRNA deadenylation by Ccr4 (Yao et al.2007; Richardson et al.2012) and that may affect the recruitment of the protein into stress granules. Therefore, the P domain was removed together with the C domain leading to protein versions composed of only one or more RRM domains (R1-R4, R2-R4, R3-R4 and R4, Fig. 1C) that were studied for their ability to localize into stress granules.
In the absence of stress, the R1–R4 variants localized predominantly in the cytoplasm, while the removal of the RRM1 domain led to a Pab1 distribution in both the nucleus and the cytoplasm (Fig. S3A and Table S1, Supporting Information), as previously observed. Interestingly, the nuclear localization of the R3-R4 variant was not as severe as that observed in R3-C and R3-P (Fig. S2A and S3A).
When cells were glucose starved or heat shocked, proteins co-localization within stress granules were not significantly affected by the removal of the P and the C domains for all the four variants (Fig. 5A; Fig. S3B and C; Table S2). Remarkably, the R4 strain showed a very low ratio of mutant/wild-type dots (0.04 in glucose-deprived and 0.12 in heat-shocked cells) (Fig. 5B; Table S3). Moreover, a poor number of cells displaying R4-associated aggregates were observed compared to the others (13.2% in glucose-deprived and 44.7% in heat-shocked cells, Table S5, Supporting Information), indicating that the RRM4 domain alone is not particularly prone to be delivered into stress granules. The propensity to be recruited into stress granules improved with the further addition of one and two RRMs: indeed, when cells were glucose starved, the ratio of mutant/wild-type dots for the R3-R4 and R2-R4 variants increased up to 0.48 and to 0.59, respectively (Fig. 5B; Table S3). Interestingly, when cells were subjected to heat shock, this parameter resulted 0.30 for R3-R4 and 0.95 for R2-R4 variants (Fig. 5B; Table S3), thus indicating that in this condition the presence of a third RRM significantly enhanced recruitment within stress granules. Finally, the R1-R4 version fully associated to stress granules independently from the triggering stressful conditions, since cells displayed almost the same number of green and red fluorescent foci (Fig. 5B; Table S3).
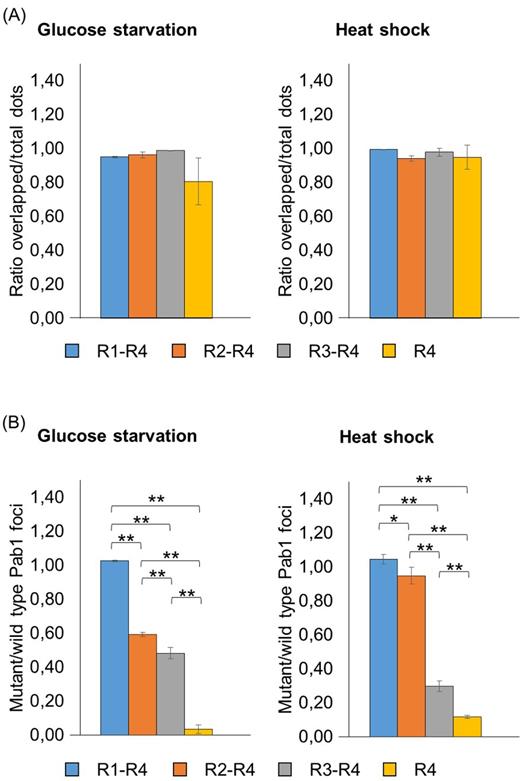
Analysis of the recruitment into stress granules of Pab1 variants lacking the P and the C domains. Strains carrying the GFP-tagged Pab1 variants shown in Fig. 1C were grown until the exponential phase of growth (OD660nm = 0.3–0.6) and then subjected to glucose starvation for 15 min or heat shock for 50 min. Data in panel (A) show the ratio between the overlapped green-red fluorescent dots and the total number of dots. Data in panel (B) show the ratio between the number of mutant (green) and the number of wild type (red) foci. Values indicate the mean and the standard deviation of three independent experiments. Significance was calculated with two-tailed student t-test (*P ≤ 0.05; **P ≤ 0.01; see Table S6).
Therefore, a synthetic version lacking the P and the C domain necessarily requires all the four RRMs to be efficiently recruited within stress granules. Furthermore, this result points out that the P domain is not strictly required for the inclusion of Pab1 into stress granules. However, the ratio of mutant/wild-type dots for R2-P, R3-P and R4-P was consistently higher compared to the corresponding variants lacking the P domain (Figs 4B and 5B; Table S3), indicating that its presence strengthens the recruitment of the N-truncated variants into stress granules.
DISCUSSION
The Saccharomyces cerevisiae poly(A)-binding protein Pab1 is one of the major actors in mRNA metabolism. By binding poly(A) tails, this protein can protect mRNAs from degradation (Simón and Séraphin 2007) and creates a scaffold for recruiting partners involved in mRNA polyadenylation (Zhao, Hyman and Moore 1999), nuclear export (Brune et al.2005; Dunn et al.2005), deadenylation (Simón and Séraphin 2007; Yao et al.2007; Parker 2012; Richardson et al.2012; Wahle and Winkler 2013), translation initiation (Wyers et al.2000) and termination (Richardson et al.2012; Roque et al.2015).
Despite several studies having been devoted to associate specific functions of this protein to its single domains, to the best of our knowledge their role in determining Pab1 association within stress granules is still unclear. Thus, in this work we sought to elucidate this point by observing the localization of several Pab1 GFP-tagged versions, lacking one or more domains, in stressful conditions known to evoke stress granules: glucose deprivation and heat shock. The endogenous Pab1 protein tagged with mCherry was used as a marker of these mRNPs aggregates (Buchan, Nissan and Parker 2010; Swisher and Parker 2010; Wheeler et al.2016).
We observed that the number of RRM domains influences Pab1 distribution between the nucleus and the cytoplasm. The RRM1 domain is implicated in the cytosolic localization of Pab1, probably because it contains the amino-terminal nuclear export sequence (NES) that has a predominant role in exporting Pab1 from the nucleus by Xpo1/Crm1 (Brune et al.2005; Dunn et al.2005).
The RRM1 domain contains the amino-terminal nuclear export sequence, which has a role in the export of Pab1 from the nucleus mediated by Xpo1/Crm1 (Brune et al.2005; Dunn et al.2005). Therefore, variants carrying the RRM1 resulted diffused in the cytoplasm, while the dual cytosolic/nuclear distribution of the variants lacking this domain might be related to a compensation between the two minor pathways responsible for Pab1 import by Kap108/Sxm1 and export by Mex67-mRNA (Brune et al.2005), whose effects could be accentuated in the absence of nuclear export sequence. Among all, the R3-C and R3-P variants showed the most intense nuclear localization, which was quite surprising since the nuclear localization signal resides in the RRM4; we should have therefore expected to find a marked nuclear localization of all the versions carrying this domain but lacking the RRM1. In addition, we observed a less intense localization of R3-R4 and R4-P variants in the nucleus. Hence, these results suggest that the presence of both the RRM3 and the P domain favors the nuclear localization or impedes the export to the cytoplasm.
We found out that the removal of one, two or three RRM domains did not affect Pab1 co-localization within stress granules, but it reduced the propensity of the protein to be recruited within them; this effect was particularly evident in the absence of two and three RRM domains. Therefore, the efficient Pab1 association to stress granules correlates with the number of RNA recognition motifs, possibly because the presence of more RRMs enables higher protein–protein interactions that boost mRNPs formation and, subsequently, the assembly of higher ultrastructures (Wheeler et al.2016). However, we cannot exclude that the presence of a single RRM1, RRM2 or RRM3, or diverse combinations of them, might behave differently because of the specific interactions in which they are involved. In fact, several post-translational modifications such as seven methyl-glutamate, five acetyl-lysine and one phosphoserine (Low et al.2014) are present along Pab1 sequence and may impact on many molecular aspects, among which the delivery of the protein into stress granules (Protter and Parker 2016).
Moreover, we discovered that the sole presence of RNA recognition motifs seems to be sufficient to allow Pab1 association within stress granules upon stress, since the variant carrying all the four RRMs fully co-localized with native stress granules even in the absence of P and C domains. Accordingly, it has been recently demonstrated that in mild stress conditions Pab1 molecules undergo phase separation even in the absence of the low-complexity P domain (Riback et al.2017). Furthermore, in the absence of all RRM domains, the P-C and C domains alone were not recruited into stress granules, thus supporting the role of RRMs in determining the specific localization of Pab1 within these aggregates.
Although the recruitment of Pab1 within stress granules can occur independently from the P domain, in the absence of at least one RRM its presence favors Pab1 association to stress granules. Therefore, we suggest that this domain may strengthen the aggregation and, consequently, the delivery of Pab1 into stress granules, thanks to its intrinsically disordered nature (Riback et al.2017) that may promote non-specific hydrophobic interactions. Indeed, variants without RRM domains formed irregularly shaped aggregates even in the absence of stress.
Interestingly, Pab1 variants lacking at least one RRM displayed a slight better recruitment within stress granules in the absence, rather than in the presence, of the C-terminal domain, even though we cannot exclude that small difference in expression levels might have influenced this result. Thus, the C domain might have a negative effect on Pab1 localization within stress granules. In particular, we hypothesize that the absence of the C domain might increase the P domain exposure, thus enhancing its intrinsically disordered nature that, in turn, will increase redundant interactions. A second possible explanation might reside in a reduced binding of Pab1 with eRF3 (involved in translation termination), Pbp1 and the PAN complex (both involved in deadenylation), whose activities are dependent on their interaction with the C domain (Richardson et al.2012; Roque et al.2015). If confirmed, this last hypothesis would open new questions about the role of these proteins in stress granule formation.
Finally, Pab1 localization within stress granules evoked by glucose starvation or heat shock was similarly affected by the removal of modules, but in some cases, the extent of the effect was different depending on the stress. This might be related to a diverse composition of the resulting granules (Buchan, Muhlrad and Parker 2008; Grousl et al.2009; Buchan, Yoon and Parker 2011; Grousl et al.2013) and/or to different mechanisms of assembly. In fact, while glucose starvation is suggested to form stress granules in conjunction with pre-existing P-bodies, heat shock is described to promote assembly independently from them (Buchan, Yoon and Parker 2011). Remarkably, it has been recently found that the lysine acetyltransferase NuA4 complex, which is known to interact with Pab1, is required for regulating stress granules formation in glucose-deprived cells but not in heat-shocked ones (Rollins et al.2017).
It is important to underline that Pab1 can undergo a process of self-association, which is dependent on the presence of the RRM1 and the P domain (Yao et al.2007). Although this phenomenon has been demonstrated to influence Ccr4/Pop2/Not deadenylation (Yao et al.2007), little is known about its role in Pab1 recruitment into stress granules. Therefore, we cannot exclude that self-association might affect the delivery into stress granules of Pab1 variants carrying or lacking the RRM1 or the P domain. In addition, in our experimental setting, the contemporary expression of the endogenous and the synthetic PAB1 versions may result in cross-oligomerization among the corresponding proteins, thus possibly affecting their recruitment into stress granules. This aspect could be better elucidated by using different pab1Δ suppressor strains, such as pab1Δ spb2Δ and pab1Δ pat1–2 (Swisher and Parker 2010), coupled with other markers of stress granules, such as Pbp1.
In conclusion, in this work we demonstrated that Pab1 domains differently contribute in the recruitment of Pab1 into stress granules. These novel findings suggest that the modularity of Pab1 may be an interesting candidate for module-based engineering, in which domains replacement or insertion can be rationally designed for the development of synthetic proteins. Surely, further studies including the modeling of Pab1 modules and their functions can be of help for understanding the dynamic equilibrium of the wild-type protein as well as for tailoring novel constructs.
SUPPLEMENTARY DATA
Supplementary data are available at FEMSYR online.
Acknowledgements
The authors acknowledge Alfredo Mento and Ilaria Vitangeli for technical contribution and data analysis, respectively. The authors acknowledge Prof. Roy Parker for providing YCplac33 EDC3-mCherry (pRP1657) plasmid.
FUNDING
This work was supported by FA (Fondo di Ateneo) of the University of Milano Bicocca and by the PhD fellowship of the University of Milano-Bicocca to MB.
Conflict of interest. None declared.