-
PDF
- Split View
-
Views
-
Cite
Cite
Andrzej Urbanik, Justyna Kozub, Paulina Karcz, Monika Ostrogórska, Changes in the brain directly following alcohol consumption—a study of healthy male individuals, with the use of proton magnetic resonance spectroscopy (1HMRS) and diffusion (DWI), Alcohol and Alcoholism, Volume 56, Issue 4, July 2021, Pages 415–424, https://doi.org/10.1093/alcalc/agaa119
- Share Icon Share
Abstract
To use proton magnetic resonance spectroscopy (1HMRS) and diffusion weighted imaging (DWI) to identify ethanol in the brain directly after consumption, and examine changes in brain metabolite levels and brain microstructure relative to the duration of time following exposure to alcohol.
The study involved 44 male volunteers (18–55 years). All brain changes were assessed in the frontal lobes, occipital lobes, basal ganglia and cerebellum, however the detailed analyses focused on the frontal lobes. All participants were examined four times, i.e. before and 0.5-hour, 1 hour and 2 hours after consumption of 150 mL pure vodka (60 g of ethanol).
The highest ethanol levels were identified between 0.5 and 1 hour following alcohol intake. There were significant increases in the concentrations of lipids and lactates approximately one hour after alcohol consumption, and the concentration levels were found to normalise during the following two hours. Some statistically insignificant trends of changes were found for tCr, tCho, mI, GABA, Glc, Glx and tNAA. For the DWI and ADC (Apparent Diffusion Coefficient of water) values, the findings showed statistically insignificant decrease and increase, followed by a tendency towards normalisation. Similar associations in changes of metabolite concentrations and DWI and ADC values were found in the other locations investigated in the study.
A single dose of alcohol as used in this experiment produces increases in lipids and lactates in brain tissues that appear reversible.
Introduction
The brain is adequately protected against numerous toxins, owing to the blood–brain barrier, i.e. a mechanism preventing direct contact between circulating blood and nervous tissues in the brain (Haorah et al., 2005, Rubio-Araiz et al., 2017). However, ethanol (being an essential component of alcoholic beverages) can pass through this barrier. In view of the widespread consumption of alcohol, and the effects it produces in the brain, numerous studies are conducted in order to examine the mechanisms of its activity (Lee et al., 2013, Anghel, 2008, Schweinsburg et al., 2000, Modi et al., 2011, Biller et al., 2009, Meyerhoff and Durazzo, 2008, Enrico and Diana, 2017, Hermens and Lagopoulos, 2018).
Most studies which employ imaging methods focus on assessment of brain morphology. Proton magnetic resonance spectroscopy technique (1HMRS) enables in vivo examination of the metabolic composition of the brain. The technique can also be used to detect the presence of ethanol and to determine its concentration. This is possible because 1HMRS signal of ethanol(ethyl alcohol) has been found to consist of peaks located at 4.8–4.9, 3.6–3.7 and 1.2 ppm (Borowski et al., 2000, Larsen et al., 2006) (Fig. 1).
In view of the fact that alcohol changes the fluidity of cell membranes, possible changes in brain tissue microstructure also seem to be particularly interesting (Yeh et al., 2009). This may be examined indirectly, by measurement of water diffusion in the brain with the use of non-invasive diffusion MR technique (diffusion weighted imaging—DWI) (Kong et al., 2012, Buhler and Mann, 2011, Fabiano et al., 2005, Baker et al., 2013, Elofson et al., 2013, Rosenbloom et al., 2003) such as altered cell membranes.
Research has mainly focused on effects of long-term alcohol abuse, to design better and more effective treatment of alcohol problems (Buhler and Mann, 2011, Estilaei et al., 2001, Baker et al., 2013). The purpose of the current study, which applied 1HMRS and DWI, was to identify ethanol in the brain directly after a single drink as well as changes in brain metabolite levels and brain microstructure relative to the duration of time following exposure to alcohol.
Material and methods
The study was approved by the local Bioethics Commission. It involved 44 male volunteers, aged 18–55 years (mean age 26.9 ± 9.9 years). Exclusion of female subjects was because of the ethical reasons as women may not be aware of being pregnant and should not consume alcohol for scientific procedures. The subjects reported no alcohol related problems at the time of the study or in the past, and only occasional light consumption of alcohol (e.g. once a week at most). None of the subjects declared any diseases and particularly any previous or actual disorder of or injury to the central nervous system. The subjects were examined after abstaining from drinking alcohol for a week, and after a night of fasting. During the experiment, they did not eat anything, nor did they drink anything except for a dose of alcohol specified in the study protocol. Body weight of the subjects was in the range of 65–88 kg (mean 76.6 kg).
The examinations were performed with a 1.5 T magnetic resonance system (SIGNA EXCITE, GE Medical System, Milwaukee, WI). The MR system was calibrated before the 1HMRS procedures. The main procedure was preceded with 1:37 minutes long T2-weighted imaging, designed to rule out any changes to the central nervous system. Parameters of the spectroscopy examination: PRESS sequence, TE—35 ms, TR—1500 ms, FA—90°, NEX—8. The duration of the single 1HMRS sequence was 2:12 minutes. Parameters of the DWI examination: TR = 8200 ms, FA = 90°, NEX = 2, b = 1500, slice thickness = 5 mm. The duration of the single DWI sequence was 2:05 minutes. Signal-to-noise ratio (SNR) and full width at half maximum (FWHM<5 Hz) were controlled during the whole study in order to obtain data of good quality.
The time procedure was as follows (fig. 2):
session 1—T2-weighted imaging, 1HMRS and DWI examinations following night fasting,
consumption of 150 mL pure vodka (60 g of ethanol) outside the scanner,
session 2—T2-weighted imaging, 1HMRS and DWI examinations started 0.5 hour after intake of alcohol,
breath ethanol concentration measurement outside the scanner,
session 3—T2-weighted imaging, 1HMRS and DWI examinations started 1 hour after intake of alcohol,
breath ethanol concentration measurement outside the scanner,
session 4—T2-weighted imaging, 1HMRS and DWI examinations started 2 hours after intake of alcohol,
breath ethanol concentration measurement outside the scanner.
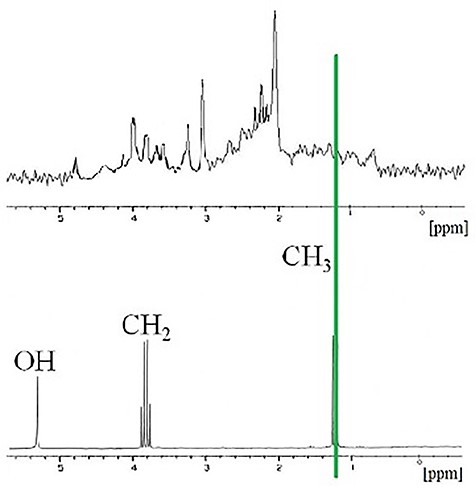
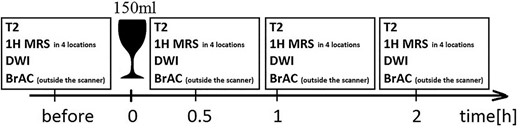
Schematic diagram of the study procedure: T2, 1HMRS and DWI were performed before, 30 minutes, 60 minutes and 120 minutes after drinking of 150 mL of alcohol; concentration of ethanol in exhaled breath (BrAC) was also measured.
Four sessions were performed at specified time intervals. In each session T2-weighted imaging was performed at first. Then 1HMRS data was obtained from four locations (dimensions of 2x2x2 cm) within the brain (Fig. 3): in the frontal lobes (centrally), basal ganglia (on the right side), occipital lobe (centrally) and cerebellum. In all exam sessions the sequence of the brain location studied was the same starting with the frontal lobes. Then DWI data was collected. Thus, the time lag for the exam to begin in each specific brain location was the same in all the sessions. Additionally, the consecutive sessions were preceded with measurement of breath ethanol concentration (BrAC) using legalised breathalyser.
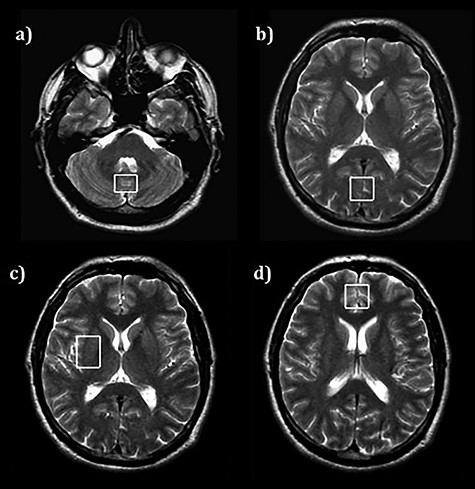
The MR signal was acquired from a) cerebellum, b) occipital lobes (centrally), c) basal ganglia (in right hemisphere), d) frontal lobes (centrally).
To define the temporal sequences for the consecutive sessions of 1HMRS examination, two volunteers were examined before the main examination as a pilot study. In this case 1HMRS scans were performed in succession before and immediately after the subjects consumed ethanol, and then every 5 minutes for the following three hours. The peak at 1.2 ppm occurred as early as 5 minutes after the intake of alcohol. The highest peak was observed in one subject after 0.5 hour and in the other subject after one hour. In both cases it visibly decreased two hours after drinking. Both the dose of ethanol consumed and the temporal sequences applied in the protocol were ultimately defined jointly with experts from the Institute of Forensic Research in Kraków.
Raw data from the 1HMRS examinations were analysed using dedicated software—SAGE 7.0 (GE Medical System, Milwaukee, WI). A spectrum was modeled with a Gaussian function with a line broadening parameter LB = 7 Hz. Based on the reconstructed 1H MR spectra, concentrations of N-acetylaspartate (tNAA, 2 ppm), Creatine (tCr, 3 ppm), Choline (tCho, 3.2 ppm), Myo-inositol (mI, 3.5 ppm), γ-aminobutyric acid (GABA, 2.2–2.4 ppm), Glucose (Glc, 3.8 ppm), Glutamine and Glutamate (Glx, 2.2–2.4 ppm), Lipids (Lip, 0.9–1.3 ppm), Lactate (Lac, 1.33 ppm) and Ethanol (EtOH, 1.2 ppm) were calculated in the studied brain locations for each session. The average concentrations from all participants for each time session were calculated and analysed in order to assess the changes in ethanol and to evaluate metabolite concentrations during the consecutive sessions. All concentrations are given in arbitrary units (a.u.).
For the results of the DWI study, raw data was analyzed in FuncTool (GE Medical System, Milwaukee, WI). The ADC parameter (Apparent Diffusion Coefficient of water) was calculated for four ROIs (regions of interest) corresponding to the brain localizations from which 1HMRS data was collected.
Regarding the presence of the time lag for the start of the examination in each specific brain location, it was impossible to compare directly results between brain regions, so the statistical analysis was performed only for the data collected from the frontal lobe. This location was selected due to the fact that many studies have pointed out that frontal lobes are most vulnerable to the effects of alcohol (Waddell et al., 2008, Schweinsburg et al., 2001, Meyerhoff et al., 2004, Parks et al., 2002, Fabiano et al., 2005). The other brain regions were only evaluated to show the trends of any metabolic or ADC changes in each localization. The changes in brain metabolites and ADC values after alcohol intake as well as the changes in ethanol concentrations in the frontal lobes and in breath before and after exposure to alcohol was examined for statistical significance using One-Way ANOVA with post-hoc Bonferroni correction. Additional correlations between ethanol concentrations in the brain and in breath were examined with reference to the participants’ self-reported tolerance to alcohol. The statistical significance for each analysis was defined as p < 0.05.
Results
Sixteen 1H MR spectra were obtained for each participant of the experiment in all the sessions of the experiment.
Ethanol changes
Following exposure to alcohol, a peak corresponding to ethanol (EtOH) could be seen in all the spectra at 1.2 ppm; it was not observed in the baseline measurement. The peak changed its height (reflecting a change in the concentration) over time (Fig. 4). Changes in ethanol levels were examined in the HMR spectra, during the experiment. During session 2, following consumption of alcohol, the level of ethanol increased significantly (p < 0.001), compared to the baseline. During the time from 0.5 to 1 hour (between session 2 and 3) the concentration decreased slightly (no statistical significance). During the time from 1 to 2 hours (between session 3 and 4), ethanol levels continued to decrease and the result was statistically significant (p < 0.05).
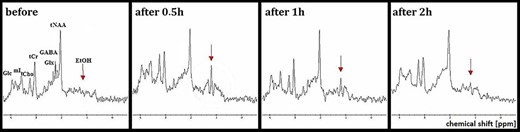
Changes in 1HMRS in the frontal lobes after drinking alcohol shown at the example spectra of a single subject; the arrow indicates the peak corresponding to ethanol.
Individual differences were found in the time needed to achieve maximum ethanol levels. However, the maximum height of ethanol peak (and concentration) in the specific subjects occurred between 0.5 and 1 hour after drinking (between session 2 and 3). The time when ethanol peak was at the highest was found to correlate with the participants’ self-reported tolerance to alcohol (18 subjects). Accordingly, the men who were found with the maximum peak height at 1.2 ppm in most locations after 0.5 hours (session 2) reported poorer tolerance to ethanol. On the other hand, those subjects who presented with the highest peak at 1.2 ppm after one hour (session 3) claimed to have high tolerance to alcohol (26 subjects). The subjective reports were fully compatible with the times of occurrence of the highest ethanol peak. After two hours (session 4) a visible decrease was found in all the subjects in the peak at 1.2 ppm (and consequently in the ethanol concentration).
Analyses of changes in ethanol levels during the experiment were also performed in the other locations of the brain, as defined earlier. The results are shown in Fig. 5. It can be observed that the curves corresponding to these changes reflect similar patterns as those related to the frontal lobe. As an exception the cerebellum curve shows that the maximum value was reached later (after one hour, compared to the remaining locations). This may be linked with the fact that the cerebellum was the final location to be examined.
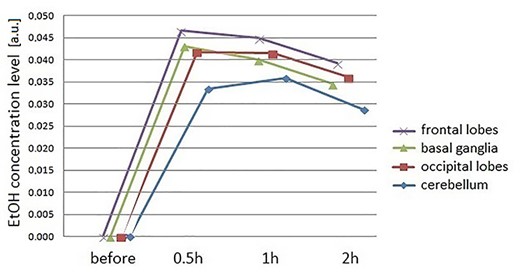
Comparison of changes in ethanol concentrations in various brain regions, in frontal lobes (x), basal ganglia (▲), occipital lobes (□) and cerebellum (◊).
The changes in ethanol levels identified with 1HMRS in the brain were compared to the results of breath tests. The results are shown in Figure 6. The findings showed a similar profile of changes in ethanol level in exhaled breath and in the concentration in the frontal lobes. Like in the case of 1HMRS analysis, breath ethanol level significantly increased, reaching the maximum value after 0.5 h (session 2), later it decreased, yet after 2 hours (session 4) it still did not go down to the baseline. Additionally, a statistically significant difference (p < 0.05) was observed between the penultimate session (after 1 hour) and the ultimate session (after 2 hours), with greater decrease in ethanol concentration in exhaled breath than in the brain.

The dynamics of changes in ethanol concentrations, average value for frontal lobes examined (dark columns) and breath ethanol concentrations (bright columns); error bars denote standard deviations.
Metabolic changes
All changes in the concentrations of the metabolites in the frontal lobe region, basal ganglia, occipital lobes and cerebellum, before and after alcohol consumption, are shown in Supplementaryy Materials.
Following alcohol consumption, within 0.5 hour, there was an increase in the concentrations of the following metabolites in the frontal lobes: tCho, tCr, mI, GABA, Lip and Lac. The findings showed only trends, without statistical significance, in the changes of these concentrations. Only in the case of lactates the p value was approaching statistical significance. During the same time a decrease in the concentrations was found for the following metabolites: tNAA, Glx and Glc; the differences in the concentrations were statistically insignificant.
Statistical significance was identified also in the increase of Lip and Lac levels between the first session (before alcohol consumption) and the third one (1 h after alcohol consumption) and in the increase of Lac level between the first session (before alcohol consumption) and the fourth one (2 h after alcohol consumption). No statistically significant Lip changes were found in other brain locations examined. Notably, a statistically significant increase in Lac concentration in basal ganglia and occipital lobes was found between the first session (before alcohol consumption) and all further sessions. This clear-cut result was obtained despite the “time lags” resulting from the differences in the timing of the measurements in the specific locations. All metabolic changes that were statistically significant are presented in Figure 7.
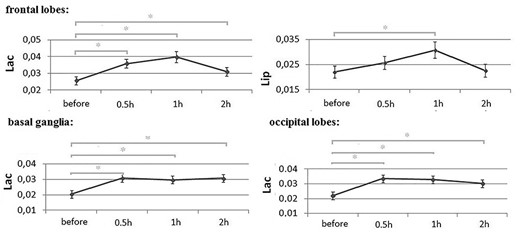
Statistically significant changes in concentrations (given in arbitrary units) of the metabolites in the frontal lobes, basal ganglia and occipital lobes, before and after alcohol consumption; error bars denote standard errors of the mean; abbreviations used in the text; *—statistically significant change (p < 0.05).
The same trends of changes in metabolite levels, within 2 hours, in all examined brain locations were found in the case of:
tCr—a moderate statistically insignificant increase,
tCho—a statistically insignificant increase followed by a decrease to a level below the baseline,
mI—a statistically insignificant increase followed by a decrease or plateau at a level higher than the baseline,
GABA—a statistically insignificant increase followed by a decrease to the baseline concentration.
In the case of the remaining metabolites the findings showed differences in trends of metabolite level changes in the specific locations examined:
tNAA—in the frontal lobes—a statistically insignificant decrease within the first 0.5 hour, followed by a statistically insignificant increase to a value exceeding the baseline, and a decrease to a level below the initial concentration; a similar profile from the location in the occipital lobes,—in basal ganglia and in cerebellum—a statistically insignificant increase within first 0.5 hour, followed by a statistically insignificant decrease but not exceeding the baseline.
Glc
in the frontal lobes a slight statistically insignificant decrease within 0.5 hour, followed by a statistically insignificant increase to a level exceeding the baseline
a statistically insignificant increase in the remaining locations.
Glx
in the frontal lobes, a statistically insignificant decrease within 0.5 hour, followed by a return to the baseline,
a statistically insignificant increase in the remaining locations.
ADC changes
The changes in ADC and DWI identified in the frontal lobes, before and after alcohol consumption, are shown in Fig. 8. Immediately, within the period 0–0.5 hour there was an increase in ADC value, followed by a decrease. The changes were not significant statistically (p > 0.05). Immediately, within the period 0–0.5 hour there was a decrease in DWI value, followed by an increase. No statistical significance was identified. In the case of the remaining brain locations the changes in ADC and DWI values were statistically insignificant, as well.
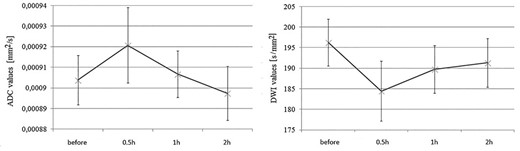
Comparison of changes in ADC and DWI values in frontal lobes; error bars denote standard errors of the mean.
Discussion
The present findings are consistent with earlier studies reporting that it is possible to use 1HMRS to perform in vivo measurements of ethanol contents in various locations of the brain (Mendelson et al., 1990a). The comparisons of the results from this study with results from the previous studies contain not only human studies but also animal studies. It is possible and reasonable because some important parallels between the two species was found in previous MR spectroscopy studies in alcoholism in both humans and animals (Frischknecht et al., 2017, Streit et al., 2018, Hermann et al., 2012).
During analysis of the current findings it is necessary to take into account the fact that a part of the ethanol cannot be seen in 1HMRS signal. This is associated with the so-called membrane-bound alcohol which is characterised by short T2 relaxation time, resulting in weak MR signal. Irrespective of that, alcohol may occur in intra- and extra-cellular fluids as the so-called “free” ethanol (Anghel, 2008, Fein and Meyerhoff, 2000). Due to the varied T2 relaxation times of these two forms of ethanol, 1HMRS allows to directly measure only the “free” ethanol. This means that “bound” ethanol cannot be seen in 1HMRS and because of this the method makes it possible to detect only a part of it present in the brain (Anghel, 2008, Kaufman and Levin, 2001, Fein and Meyerhoff, 2000).
EtOH
Review of the related literature shows that few 1HMRS studies have focused on immediate effects of alcohol consumption, and some of these involved animals. Liu et al. (Liu et al., 2014) examined a group of 25 adult rats given ethanol via gastric administration and a control group of rats given drinking water. Spectra were obtained through measurements after 1, 3, 5, 12 and 24 hours. Maximum EtOh levels were found after one hour, later there was a decrease.
First studies regarding possibility of measuring ethanol in human brain were performed by Mendelson et al (Mendelson et al., 1990a, Mendelson et al., 1990b). Six healthy adult men (age: 23–26 years; weight: 77.5 ± 3.7 kg) were studied before and after they drank a mixed drink of orange juice and vodka (0.7 g/kg). The measurement was repeated every 5 minutes starting in 20th minute after ethanol administration till 120th minute. The most important result of this examination was that no alcohol resonance is present prior to drinking, but 35 minutes after drinking a clearly distinguishable ethanol peak is observed (Mendelson et al., 1990b).
Biller et al. (Biller et al., 2009) examined 15 subjects aged 20–38 years (mean 23.9 ± 4.6 years) including 7 women and 8 men. The subjects drank 300 mL of a beverage containing 0.65 g of ethanol per each kilogram of body weight in the females, and 0.75 g of ethanol per each kilogram of body weights. The measurements were performed seven times within 93.71 minutes. Another measurement was carried out after 12 hours. Blood samples were also collected from the subjects. The peak corresponding to EtOH in the brain, was visible in 1H MR spectrum (1.19 ppm) after 5.79–8.64 minutes from exposure to alcohol (the time was different in the specific subjects). It reached a maximum value after 91.14 ± 11.69 minutes (in the fronto-mesial region) and 74.43 ± 5.58 minutes (in the cerebellum), and there were no differences in ethanol levels between the locations examined. A similar experiment was carried out by Mendelson et al. (Mendelson et al., 1990a). The six volunteers were given a drink containing orange juice and vodka (0.7 g ethanol per one kilogram of body weight). Spectroscopy data were collected from selected regions every 5 minutes for 2 hours after the volunteers were exposed to alcohol. In this study blood samples were also analysed. The highest EtOH peak appeared approximately after 50 minutes (15 minutes later than the maximum blood alcohol contents). A group of 20 subjects (18 males and 2 females; mean age of 32 ± 6 years) was examined by G. Fein and D.J. Meyerhoff (Fein and Meyerhoff, 2000). The participants consumed 0.85 g of ethanol (with orange juice) per one kilogram of body weight. The study also investigated blood and breath alcohol concentrations. 1HMRS exams were performed after 60 minutes from exposure to alcohol. The concentrations of EtOH in the brain were found to correlate with both blood and breath alcohol levels. Importantly, additional tests were carried out using a magnetization transfer technique allowing a conclusion that 1HMRS enables identification of EtOH fraction only.
In the study prepared by Kubo et al. (Kubo et al., 2013), four subjects (3 men, 1 woman; mean age: 26.5 years) fasted 4 h and then drank 750 mL of alcoholic liquor (7%) with a light meal. The continuous measurement (before and after 10, 25, 40, 55 minutes) showed time-dependent changes in alcohol in the brain (Kubo et al., 2013).
While making comparisons of various findings it should be emphasised that the present study involved a significantly larger group of subjects than the other reports referred to. Most importantly, the earlier and the present findings show that maximum ethanol levels occur within 30–60 minutes (50–90 minutes in the studies referred to) and then they visibly decrease.
Furthermore, in accordance with the present findings breath alcohol concentrations measured with a breathalyser, present similar dynamics of changes compared to ethanol concentrations in the brain measured with 1HMRS, which is consistent with the results reported by the above studies. It was also confirmed, in line with other research, that the level of ethanol in exhaled breath decreases much faster than in the brain. This fact is important in the context of sobriety testing with the use of breathalysers, because it has been demonstrated that high levels of ethanol in the brain are maintained longer, compared to results of tests related to exhaled breath. The present study showed that the difference is statistically significant.
tNAA
N-acetylaspartate (tNAA) is a marker of neuronal viability and density (Kok et al., 2001, Limperopoulos and Clouchoux, 2009, Chrzan et al., 2013, Zahr and Pfefferbaum, 2017); it also reflects oligodendrocyte proliferation and differentiation (Girard and Chaumoitre, 2012). The current findings do not show statistically significant changes in tNAA concentrations in the frontal lobes following alcohol consumption. The observations showed only a statistically insignificant decrease within the first 0.5 hour, followed by an increase exceeding the baseline value, and then a decrease.
Adalsteinsson et al. (Adalsteinsson et al., 2006), who examined 10 adult rats (age: 150–250 days), did not observe changes in tNAA levels within 1–1.5 hours following intraperitoneal or intragastric alcohol injection. No such changes were also reported by Liu et al. (Liu et al., 2014) who examined 60 adult rats (age: 8–10 weeks) divided into two groups: one group was given ethanol via oral administration (15 mL ethanol per 1 kg of body weight), and the other group was given drinking water. Likewise, no statistically significant changes in tNAA concentrations were identified in people following alcohol consumption, by Biller et al. (Biller et al., 2009), Monnig et al. (Monnig et al., 2019) or Gomez et al. (Gomez et al. 2012).
According to Meyerhoff et al. (Meyerhoff et al., 2004), who examined 46 chronic heavy drinkers (age range: 27–56 years; mean age: 41.3 ± 9.4 years; 100 alcoholic drinks containing 13.6 g of pure alcohol per month over 3 years before the study) and 52 light drinkers (age range: 21–56 years; mean age: 41.0 ± 8.5 years; less than 45 drinks per month), tNAA in the frontal white matter (WM) was lower in heavy than light drinkers; that was associated with lower executive and working memory functions. Moreover, frontal WM tNAA was lower in female than in male heavy drinkers.
tCr
Creatine (tCr) is a marker of energy transformations and intracellular metabolism (Cichocka and Urbanik, 2017). In the current study, tCr concentrations, following alcohol consumption by the volunteers, were found to increase moderately throughout the experimental procedure (i.e. 2 hours), the increase however, was not statistically significant. Similarly, earlier studies in rats, following intraperitoneal or intragastric alcohol injection (Adalsteinsson et al., 2006), or oral alcohol administration (Liu et al., 2014), failed to show statistically significant changes in Cr concentrations. Xu et al. (Xu et al., 2018) conducted a study involving mice and found lower tCr levels in the thalamus in the ethanol-fed specimens compared to the control group. Biller et al. (Biller et al., 2009) observed significant decrease in supratentorial tCr and no changes in infratentorial tCr concentrations in 15 individuals examined following alcohol exposure.
tCho
Biller et al. (Biller et al., 2009) observed a decrease in supratentorial and infratentorial tCho levels in 15 humans examined following exposure to alcohol. On the other hand, Monnig et al. (Monnig et al., 2019) examined 13 individuals before and after they consumed 0.6 g of alcohol per kilogram of body weight; their findings showed a statistically significant increase in thalamic tCho/tCr concentrations, approximately 5 hours after the exposure.
The study by Modi et al., involving individuals addicted to alcohol, showed increase in tCho/tCr concentrations in the occipital lobes (Modi et al., 2011).
According to de Souza et al. (de Souza et al., 2018), 23 AUD patients showed lower tCho/tCr ratio when compared to 23 non-AUD controls in the left prefrontal cortex. No difference was found in the right prefrontal cortex.
mI
Myo-inositol (mI) occurs in glial cells (mainly in astrocytes) and, consequently, is their marker (Story et al., 2011, Mailath-Pokorny et al., 2012, Story et al., 2013, de Graaf, 2007, Zahr and Pfefferbaum, 2017). In the current study, mI levels in the participating volunteers were found to increase insignificantly within the first hour following alcohol consumption, and then there was a decrease. Earlier studies in animals and humans also failed to show a lack of changes in mI concentrations; these include the studies by Liu et al. (Liu et al., 2014) and Biller et al. (statistically insignificant decrease in the concentrations) (Biller et al., 2009).
GABA
GABA, i.e. γ-aminobutyric acid, is an inhibitory neurotransmitter (Harris et al., 2017, de Graaf, 2007, Ende, 2015)—glutamate antagonist (Chrzan et al., 2013). It is also involved in the regulation of synaptic integration and neural plasticity (Ende, 2015, Cichocka and Urbanik, 2017). Changes in GABA levels may lead to mood disorders (Luscher and Fuchs, 2015). In the current study, GABA concentrations increased within the first hour following exposure to alcohol, and then they started to decrease. These changes were not significant statistically. Previously, behaviour of this metabolite following exposure to alcohol was not widely investigated. It was only observed by Gomez et al. (Gomez et al., 2012) that there was a statistically significant decrease in GABA levels in the occipital lobe in 11 volunteers, which was reflected by examinations conducted before and 90 minutes after intravenous ethanol infusion.
Glutamine and Glutamate
Glutamine (Glu) and glutamate (Gln) are dominant excitatory neurotransmitters (Brighina et al., 2009, Harris et al., 2017, Govindaraju et al., 2000). The current findings showed statistically insignificant decrease in Glx levels in the frontal lobes within 0.5 hours from exposure to alcohol, followed by a return to the baseline (the profile of the changes opposite to that observed in the case of EtOH). The remaining locations were found with statistically insignificant changes in Glx levels.
Liu et al. (Liu et al., 2014) also observed negative correlations between EtOH levels and Glu concentrations in rats exposed to alcohol. Furthermore, Xu et al. (Xu et al., 2018) reported higher concentrations of Gln in the hippocampus and thalamus as well as Glu in the hippocampus in alcohol-fed mice, compared to the control group.
Biller et al. (Biller et al., 2009) observed increased Glu levels in 15 human subjects examined following exposure to alcohol. On the other hand, Leurquin-Sterk G et al. (Leurquin-Sterk et al., 2018) examined 11 volunteers aged 22.9–62.6 years (mean: 40.1 ± 12.2 years) and observed that alcohol consumption resulted in decreased Glx levels in the anterior cingulate cortex (ACC). Monnig et al. (Monnig et al., 2019) examined 13 subjects, before and after they drank 0.6 g alcohol per kilogram of body weight, and found increased Glx/tCr concentrations in the thalamus approximately after 5 hours from exposure to alcohol. Another study involving healthy volunteers (32 men, mean age 25.1 yrs.; range 18–36 yrs.) was performed by Betka et al. (Betka et al., 2019). The negative correlation between Glx concentration with AUDIT (Alcohol Use Disorders Identification Test) score was observed.
Lipids
A peak corresponding to lipids (Lip) is not visible in 1H MR spectrum of a healthy brainIn pathological conditions it reflects either damage to cell membrane or necrosis (Cichocka and Urbanik, 2017). It was established that concentrations of lipids in tissues correlate with the intensity of necrosis (Cichocka and Urbanik, 2017). Lipids are visible in 1H MR spectra during ischemic stroke (Seeger et al., 2003, Gasparovic et al., 2001). In the current study lipid concentrations in the volunteers exposed to alcohol tended to increase during the first hour, and then the levels started to decrease. These changes were statistically significant, and their profile was positively correlated to the changes in EtOH levels. No analyses of changes in Lip levels, in relation to alcohol consumption, were found in the available literature.
Lactate
A peak corresponding to lactates (Lac) is not visible in 1H MR spectrum of a healthy brain. A visible Lac peak provides evidence for the presence of anaerobic metabolism, and oxygen deficiency in the brain due to pathological changes, for instance stroke (Zhu and Barker, 2011, Cichocka and Urbanik, 2017). Lactate levels following consumption of alcohol, by the volunteers in the present study, were increasing within the first hour and then started to decrease. These changes were statistically significant, and their profile was positively correlated to the changes in EtOH levels. No analyses of changes in Lac levels, in relation to alcohol consumption, were found in the available literature.
DWI
Diffusion of water is obstructed mainly by myelin sheaths and cell membranes, hence it is affected by axon density (Yeh et al., 2009). Therefore the value of ADC is determined in DWI examinations in order to identify overall diffusivity in the person’s brain (Kong et al., 2012). In view of the above, based on this parameter it is possible to assess whether exposure to alcohol leads to abnormalities in brain microstructure, not visible in conventional MRI (Kong et al., 2012). The structures most commonly listed among those which are most vulnerable to the effects of alcohol include the frontal lobe (Waddell et al., 2008, Schweinsburg et al., 2001, Meyerhoff et al., 2004, Parks et al., 2002, Fabiano et al., 2005). Given this, the current study focused on analyses of the microstructure of this brain region.
Biller et al. (Biller et al., 2009) did not find evidence of changes in neuronal integrity or water contents in the brain immediately following alcohol consumption. Similarly, Duning et al. (Duning et al., 2008) did not observe any significant changes in the ADC values, relative to the time from ethanol consumption in any of the ROI examined. In line with those reports, the current findings do not show significant changes in the ADC between the consecutive measurements. Conversely, Kong et al. (2012) observed significantly reduced ADC values in the frontal lobe after 1 hour, and in the middle part of the cerebellum after 2 hours from exposure to alcohol in all the healthy subjects, irrespective of the amount of alcohol consumed. The authors hypothesised that this may be associated with the appearance of cytotoxic oedema. They also reported no statistically significant changes in the other brain regions examined, which is consistent with our findings. After the minimum values were reached, ADC gradually increased. The examination performed by the authors after 3 hours showed increased ADC in the frontal lobe and thalamus which may suggest gradual waning of the oedema (Kong et al., 2012). The changes in ADC values, shown in the current study, are consistent with the results reported by Liu et al. (2014) and related to the first three hours following exposure to alcohol. Likewise, Luo et al. (2017) found that ADC value did not differ significantly in alcohol-fed rats and the controls.
Study limitations
The current study has certain limitations. To strengthen any future analysis, it would be beneficial to extend the scope of research to include female volunteers, which was impossible in this study for ethical reasons. It would also be desirable to evaluate all parameters in further time points in order to check if and when exactly they go back to same levels as before alcohol intake. Finally, an interesting issue, for which the present study may be the basis, is studying the effects of alcohol consumed in small doses but regularly.
Conclusion
The current study shows it is possible to assess the dynamics of alcohol present in the brain, as well as metabolic changes and changes in the diffusion parameters directly following alcohol consumption. The detailed analysis in strictly defined time sequences focused on the frontal lobes. Changes were also examined in the occipital lobes, basal ganglia and in the cerebellar vermis, however the “time lag” made the assessment difficult. The most evident consequences included the statistically significant increases in lipid and lactate levels, identified after approximately one hour from consumption of 60 mL of pure alcohol, which was followed by normalisation of the concentration during two hours. As for DWI and ADC values, the findings showed statistically insignificant decrease and increase, respectively, followed by a tendency towards normalisation. Similar relations were found in the remaining locations examined in the study. The dynamics of changes in brain ethanol concentrations corresponds to the changes in breath ethanol levels, yet the decrease in ethanol concentration in exhaled breath occurs faster than in the brain. This information is of key importance in the context of sobriety testing based on breathalysers, because ethanol in the brain is retained longer compared to results of tests related to exhaled breath. In general, based on the current study it can be assumed that one-time amount of alcohol used in this experiment produces adverse effects in the brain tissues that seem to be reversible.
Declaration of interest
The authors declared no potential conflicts of interest with respect to the research, authorship, and/or publication of this article.