-
PDF
- Split View
-
Views
-
Cite
Cite
Ting Yao, Yi Zhang, Mengjiao Lv, Guoqing Zang, Soon Seng Ng, Xiaohua Chen, Advances in 3D cell culture for liver preclinical studies, Acta Biochimica et Biophysica Sinica, Volume 53, Issue 6, June 2021, Pages 643–651, https://doi.org/10.1093/abbs/gmab046
- Share Icon Share
Abstract
The 3D cell culture model is an indispensable tool in the study of liver biology in the field of health and disease and the development of clinically relevant products for liver therapies. The 3D culture model captures critical factors of the microenvironmental niche required by hepatocytes for exhibiting optimal phenotypes, thus enabling the pursuit of a range of preclinical studies that are not entirely feasible in conventional 2D cell models. In this review, we highlight the major attributes associated with and the components needed for the development of a functional 3D liver culture model for a range of applications.
Introduction
A 3D cell culture is defined as an artificial environment that allows cells to grow and interact in all three spatial dimensions, mimicking the in vivo architecture of the liver that comprises an intricate structural pattern, extracellular matrix (ECM), and non-parenchymal cells (NPCs) [1]. The 2D cell culture model provides suboptimal preclinical results because of the lack of proper cell–cell and cell–matrix interactions, thereby losing some critical cellular phenotype. For instance, the expression levels of many liver-specific genes are decreasing, and the functions of primary human hepatocytes (PHHs), such as albumin secretion, viral infectivity, and cytochrome P450 (CYP) enzyme activity, are rapidly deteriorating in 2D cell culture upon seeding [2]. The 3D culture was first described in 1912; this opened up avenues to address the limitations of 2D models [3]. Along with the development of biomaterials and biotechnology, 3D systems with a broad range of attributes and features have been proposed to address the limitations associated with 2D models (Tables 1 and 2).
3D culture system . | Key point . |
---|---|
Spheroid |
|
Microporous hydrogel |
|
Macroporous hydrogel |
|
Bioreactors |
|
3D bioprinting | Includes Inkjet bioprinting, EBB, and LAB
|
3D culture system . | Key point . |
---|---|
Spheroid |
|
Microporous hydrogel |
|
Macroporous hydrogel |
|
Bioreactors |
|
3D bioprinting | Includes Inkjet bioprinting, EBB, and LAB
|
3D culture system . | Key point . |
---|---|
Spheroid |
|
Microporous hydrogel |
|
Macroporous hydrogel |
|
Bioreactors |
|
3D bioprinting | Includes Inkjet bioprinting, EBB, and LAB
|
3D culture system . | Key point . |
---|---|
Spheroid |
|
Microporous hydrogel |
|
Macroporous hydrogel |
|
Bioreactors |
|
3D bioprinting | Includes Inkjet bioprinting, EBB, and LAB
|
Primary material . | Advantage/disadvantage . | Relative 3D scaffold . |
---|---|---|
Polyethylene glycol (PEG) | 1. Covalently cross-linkable with ECM proteins. 2. Tunable mechanical stiffness. 3. Highly modular building blocks. 4. Highly transparent and scalable structure. 5. Highly consistent monomer size and structure. | 1. PEG hydrogel scaffold 2. Hybrid hydrogel |
Natural material | 1. Closest to microenvironment. 2. Holistic biochemical signals. 3. Inconsistent monomer size and structure. 4. High batch-to-batch variation. | 1. Decellularized liver scaffold 2. Matrigel |
Chitosan | 1. Biocompatible. 2. Biodegradable. 3. Highly modular. 4. Easy to process from natural materials. 5. Resembles glycosaminoglycans. | 1. Chitosan-gelatin porous structures 2. Chitosan nanofiber |
Alginate | 1. Biocompatibility. 2. Low immunogenicity. 3. High clinical precedent. 4. High batch-to-batch variation. | 1. GL–Alg–Ca hydrogel 2. Chitosan alginate scaffold |
Primary material . | Advantage/disadvantage . | Relative 3D scaffold . |
---|---|---|
Polyethylene glycol (PEG) | 1. Covalently cross-linkable with ECM proteins. 2. Tunable mechanical stiffness. 3. Highly modular building blocks. 4. Highly transparent and scalable structure. 5. Highly consistent monomer size and structure. | 1. PEG hydrogel scaffold 2. Hybrid hydrogel |
Natural material | 1. Closest to microenvironment. 2. Holistic biochemical signals. 3. Inconsistent monomer size and structure. 4. High batch-to-batch variation. | 1. Decellularized liver scaffold 2. Matrigel |
Chitosan | 1. Biocompatible. 2. Biodegradable. 3. Highly modular. 4. Easy to process from natural materials. 5. Resembles glycosaminoglycans. | 1. Chitosan-gelatin porous structures 2. Chitosan nanofiber |
Alginate | 1. Biocompatibility. 2. Low immunogenicity. 3. High clinical precedent. 4. High batch-to-batch variation. | 1. GL–Alg–Ca hydrogel 2. Chitosan alginate scaffold |
Primary material . | Advantage/disadvantage . | Relative 3D scaffold . |
---|---|---|
Polyethylene glycol (PEG) | 1. Covalently cross-linkable with ECM proteins. 2. Tunable mechanical stiffness. 3. Highly modular building blocks. 4. Highly transparent and scalable structure. 5. Highly consistent monomer size and structure. | 1. PEG hydrogel scaffold 2. Hybrid hydrogel |
Natural material | 1. Closest to microenvironment. 2. Holistic biochemical signals. 3. Inconsistent monomer size and structure. 4. High batch-to-batch variation. | 1. Decellularized liver scaffold 2. Matrigel |
Chitosan | 1. Biocompatible. 2. Biodegradable. 3. Highly modular. 4. Easy to process from natural materials. 5. Resembles glycosaminoglycans. | 1. Chitosan-gelatin porous structures 2. Chitosan nanofiber |
Alginate | 1. Biocompatibility. 2. Low immunogenicity. 3. High clinical precedent. 4. High batch-to-batch variation. | 1. GL–Alg–Ca hydrogel 2. Chitosan alginate scaffold |
Primary material . | Advantage/disadvantage . | Relative 3D scaffold . |
---|---|---|
Polyethylene glycol (PEG) | 1. Covalently cross-linkable with ECM proteins. 2. Tunable mechanical stiffness. 3. Highly modular building blocks. 4. Highly transparent and scalable structure. 5. Highly consistent monomer size and structure. | 1. PEG hydrogel scaffold 2. Hybrid hydrogel |
Natural material | 1. Closest to microenvironment. 2. Holistic biochemical signals. 3. Inconsistent monomer size and structure. 4. High batch-to-batch variation. | 1. Decellularized liver scaffold 2. Matrigel |
Chitosan | 1. Biocompatible. 2. Biodegradable. 3. Highly modular. 4. Easy to process from natural materials. 5. Resembles glycosaminoglycans. | 1. Chitosan-gelatin porous structures 2. Chitosan nanofiber |
Alginate | 1. Biocompatibility. 2. Low immunogenicity. 3. High clinical precedent. 4. High batch-to-batch variation. | 1. GL–Alg–Ca hydrogel 2. Chitosan alginate scaffold |
The liver plays an important role in a variety of critical bodily functions, such as detoxification of the systemic and portal blood, and secretion of multiple proteins and bile components [4]. Drugs administered orally are first processed by the liver and broken down into multiple metabolites before entering the systemic circulation. This first-pass effect executed by the liver affects the pharmacokinetics of drugs in the body and, in turn, dictates the eventual therapeutic efficacy. Termination of the clinical trials of most drugs along the discovery pipeline or their withdrawal from the market is caused by unintended adverse effects that were not predicted in preclinical toxicological studies [5]. For instance, acetaminophen (APAP) causes acute liver injury due to the incomplete expression of critical transporters on apical membrane and cytochrome CYP3A4 metabolic enzymes. In other words, the 2D hepatocyte culture is less susceptible to APAP-induced toxicity [6]. In addition to the use of 3D culture models in regenerative medicine and drug discovery, they could serve as imperative models for various liver diseases to better understand hepatocellular carcinoma (HCC) [7].
In this review, we introduce the attributes and niche factors associated with the 3D liver culture system and then focus on some relevant advanced 3D systems and their respective applications.
Attributes of the 3D Liver Cell Culture
The principal aim of developing a novel 3D liver culture model is to acquire liver phenotypes and functionalities in vitro. To achieve this, attributes such as microenvironmental architecture, phenotypical sustainability, and authentic functionality of the liver must be evaluated in the development of a new 3D culture model.
Microenvironmental Architecture
Various 3D models have been proposed to recapitulate the microenvironments of liver tissues in vitro to achieve authentic cell–cell interactions and cell polarity, which are essential for hepatic phenotypes [8–10]. The nature of the interaction of hepatocytes with their microenvironment dictates their overall shape and cytoarchitecture. Moreover, these are related to the expression of transcription factors and gene programs. The sandwich model, where a monolayer of hepatocytes is sandwiched by two layers of ECM proteins such as collagen I or Matrigel [2], is a quasi-3D configuration that prolongs hepatic functions by up to 2 weeks in culture, thus enabling short-term studies such as those on bile salt transportation and the mechanism underlying cholestatic toxicity [11]. The 3D hydrogel cell-encapsulated model is another model heavily employed to facilitate extensive cell–cell and cell–matrix interactions in 3D [12]. Unlike the sandwich model, the cell encapsulation model allows a higher degree of mobility for cells to navigate. However, the downside is that cells generally suffer from necrosis due to the suboptimal mass transportation properties in 3D hydrogels [13] (Fig. 1). To circumvent this limitation, a series of macroporous 3D scaffolding models such as salt-leaching scaffolds, inverse colloidal crystal scaffolds, and nanofiber scaffolds have been developed to deliver highly porous scaffolds that allow cells to move freely in the 3D microenvironment without compromising their physical integrity.
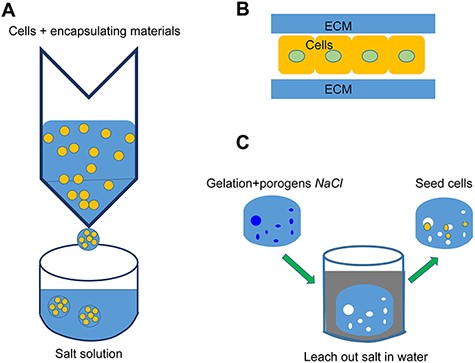
Conventional 3D models (A) Cell encapsulation model: Cells and alginate solution mixture are dispersed into a salt bath using a syringe for rapid polymerization to form microbeads. (B) Sandwich model: A monolayer of hepatocytes is sandwiched by two layers of an ECM protein such as collagen I or by Matrigel. (C) Salt-leaching hydrogel: Sacrificial salt porogens are initially mixed with the hydrogel for polymerization, then leached out to form a macroporous hydrogel.
Phenotypes and Functions
The models to date fail to recapitulate some critical phenotypes of hepatocytes in vitro, hence limiting their preclinical functions, particularly in the assessment of metabolism-driven cytotoxicity. The most potent or toxic form of a compound may not be the primary compound, but rather one of its metabolites [14]. Freshly isolated PHHs are considered the gold standard model for in vitro studies. Unfortunately, the viability and phenotypes of PHHs rapidly deteriorate due to dedifferentiation upon plating [15]. To circumvent this limited study window presented by PHHs, hepatoma cell lines (such as HepG2, Hep3B, and Huh7) due to their superior amplification ability, relatively stable phenotypes, and advanced 3D culture systems have been proposed [16–18]. However, hepatoma cell lines are also known to demonstrate significant variation in their performance and suboptimal phenotypes, particularly in detoxification and nitrogen and carbohydrate metabolism [19]. Therefore, it is imperative to develop cell culture models for predicting drug toxicity.
Critical Components of 3D Liver Cell Culture
Interaction between the cellular and ECM
Hepatocytes rely on intricate interactions with their surroundings to sustain proper hepatic phenotypes and functions. The disruption of parenchymal cell arrangement, NPC interaction, and ECM composition and configuration is known to be the major factor driving the deterioration of liver function in 2D models [20].
Homotypic cell interaction.
Extensive cell–cell interaction has been known to have a direct impact on gene expression profiles and hence on function. Studies have revealed that spheroids, where hepatocytes establish tight homotypic interactions in clusters, are more effective than 2D models in capturing liver phenotypes and functions [2,6,13]. Another critical feature of the spheroid model is the diffusivity gradient associated with the spheroid and it could be applied to model the metabolic zonation of the liver acinus, thus making this model a more relevant platform to study metabolism, synthesis, and regeneration [21].
ECM interaction.
The ECM comprises a mosaic of lipids, proteins, and carbohydrates, constituting a complex, heterogeneous, and dynamic microenvironment that is critical for maintaining the hepatic phenotype and hosting NPCs [2,22]. Various soluble factors like growth factors are certainly potent, and physical factors specifically the flexibility of the microenvironment contribute to differentiation [23]. Integrins are the crucial molecules for cells engaging their surroundings to adhere to ligands abundantly displayed on the ECM. Recent biological studies have validated the importance of integrins to the cell spreading, cell survival [24], fundamental metabolism of the hepatocytes [25], hepatocyte-specific genes, and proteins expression for hepatocyte-like cells (HLCs) differentiated from stem cells [26]. Collagen is the major component of the ECM and has been widely utilized in cell culture because of its excellent characteristics, including biocompatibility, mechanical strength, degradability, and limited immunogenicity [27]. Matrigel, an acid-urea extract from Engelbreth–Holm–Swarm tumor tissues, is widely used in hepatocyte culture for its superiority in preserving hepatic activity [28]. However, due to its xenobiotic nature and ill-defined composition, the viability of Matrigel as a reliable ECM source for cell culture is currently being debated. To enhance consistency, researchers have utilized two recombinant full-length human laminins (pure laminin 521 (L521) and a blend of laminins 521 and 111) as substrates for human embryonic stem cell (hESC)-derived differentiation [29]. However, some studies have shown that limited matrix formation is favorable for preserving the functions of adult hepatocytes and enhancing hepatic stem cell differentiation on the densely packed ECM surface [30].
NPC interaction.
Even though NPCs account for only 20% of the liver mass, they play a critical role in the construction and maintenance of ECM as well as the mediation of cellular function, including transport and metabolism [31]. Major liver NPCs include liver sinusoidal endothelial cells (LSECs), hepatic stellate cells (HSCs), and Kupffer cells (KCs).
LSECs serve as a channel for supplying nutrients and oxygen into the liver, maintaining overall hepatic homeostasis and clearance, metabolizing drugs and xenobiotics, and influencing some idiosyncratic chemical-induced hepatotoxicities [32]. The co-culture of primary hepatocytes with LSECs results in the maintenance of hepatocytes with stable phenotypes for a longer time and with better proliferation [33].
Mature HSCs produce both network and fibrillar collagens (large amounts of type I collagen and lower levels of type III, IV, and V collagen), large amounts of elastin, and both heparan sulfate proteoglycans and chondroitin. HSCs also produce important cytokines and growth factors for intercellular communication in normal and injured liver [34]. HSCs could maintain hepatocyte structure and function such as albumin secretion, urea synthesis, expression of hepatocyte-specific genes and function of cytochrome P450 (CYP450) in co-culture with hepatocyte. These effects were generated either by direct cell contacts or via factors derived from HSC-conditioned medium, preferentially through cell-bound signal and transfer of lipids [35,36].
KCs play an active role in immune surveillance, liver pathogenesis, and modulation of extrahepatic systemic inflammation via antigen presentation and suppression of the activation and proliferation of T cells [37]. Notably, the presence of KCs in hepatocyte culture has demonstrated that inflammation is one of the factors that may increase the sensitivity of hepatic cells to APAP-induced toxicity [38].
Bioengineered scaffolds
The liver is made of a repeated fundamental unit called the hepatic lobule. Lobules are arranged in a hexagonal pattern in a polygon-shaped block. Portal triads consisting of the hepatic artery, bile duct, and portal vein are at the corners of the lobule, while the central vein is located at its center (Fig. 2A). Plates of hepatocytes radiate from the central vein to the lobule perimeter. The lining of hepatocytes serves as the microcosm of the major hepatic microenvironments, containing the essential cellular and physiological features that define the unique architecture of the liver tissue [2,15] (Fig. 2B).
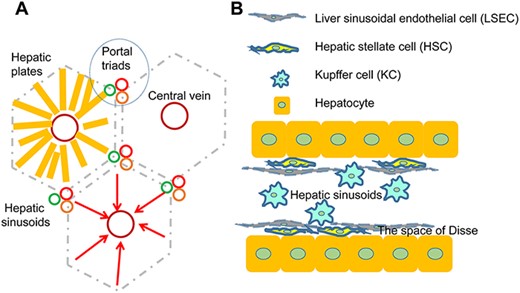
The structure of the hepatic lobules (A) The structure of the hepatic lobules and interlobules. (B) The location of the variety of liver cells.
To capture the ideal in vitro hepatic phenotypes, decades of effort have been dedicated to recreating the liver microenvironment using various scaffold-based technologies. In liver tissue engineering, the ideal scaffold should serve as a temporary platform to host hepatocytes and provide necessary biophysical and biochemical cues for cells to attach, expand, and ultimately become functional for application [39]. Decellularized matrix scaffolds, channel-liked scaffolds, printing technology, and other methods (some models shown in Fig. 3) produce a porous structure with an excellent surface area-to-volume ratio, facilitating infiltration and communication.
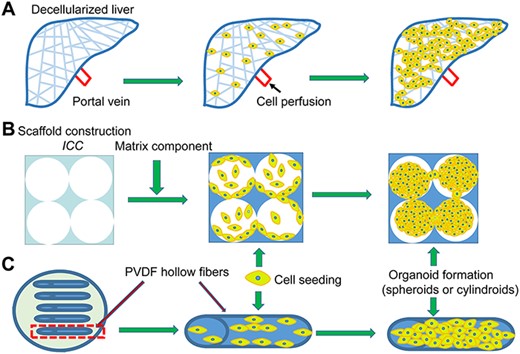
Advanced 3D model (A) Decellularized liver: The liver organ is decellularized and reseeded using target cells for repopulation over the native matrix. (B) ICC: Hydrogel with highly uniform pore size and porosity mimicking the 3D hexagonal structure of the liver lobule. (C) Channel-liked scaffolds: Cell suspensions are injected into the hollow fibers to form cylindroids.
Scaffold-free.
Scaffold-free spheroids form as hanging drops in multi-well plates with ultra-low attachment surfaces or stirred tank bioreactors, and on a large scale, by self-aggregation in the absence of added substrates that promote cell attachment [40]. For drug toxicity tests, the major drawbacks of scaffold culture systems include lack of scalability, binding of drugs to scaffolds, difficulties in handling, and batch-to-batch differences in ECM substrates, which affect reproducibility [15]. Other than scaffolds, the binding of drugs and/or drug metabolites to serum proteins may confound the interpretations of viability data. Thus, some researchers have developed and extensively characterized an easily scalable 3D PHH spheroid system under serum-free, chemically defined conditions, suitable for long-term (maintained for 5 weeks) functional toxicological studies of liver diseases [41].
Decellularized matrix scaffold.
The advantage of using a decellularized liver scaffold is the preservation of liver-specific ECM, architecture, and bioactive molecules, thus providing the necessary signals for hepatocyte engraftment, survival, and function [42]. De Kock et al. [43] used the decellularized rat livers to evaluate anticancer drug efficacy that opened the possibility of using the rat liver scaffold as a viable 3D model for drug screening. Kamal et al. [44] followed and succeeded in generating decellularized rat liver scaffolds that preserve the vascular structure and ECM integrity via the effective removal of cellular and nuclear materials. The decellularized scaffold is then repopulated by functional donor cells through portal vein perfusion [42,43]. Decellularized livers, derived from natural organs, are the ideal structural components of the cell microenvironment, which is composed of a sophisticated assembly of collagens, proteoglycans, laminins, elastin, and growth factors. These components represent the basic substances needed for cell growth attachment, growth, and proliferation [44].
Hollow fiber scaffolds.
Hollow fiber scaffolds capture the channel-like structure of hepatocytes. These scaffolds provide structural benefits to cell culture, such as enabling maximal surface for cell attachment and allowing mass transportation and exchange between the attached cells and the media constantly [45]. There are two major types of hollow fiber scaffolds differentiated by the location of the cell that forms cylindroids: one has a rough inner surface favoring hepatocyte adherence, such as polyvinylidene fluoride hollow fiber [39], and the other provides the external surface of the hollow fiber for hepatocyte seeding while the inner channel serves as a pseudovascular structure [46]. These culture systems can maintain cell strong growth ability, synthetic and secreted ability as well as metabolism enzymes at least 2 weeks [39,47].
Microporous scaffolds.
Microporous scaffolds are achieved by polymerization of cells and hydrogel mixture [45,48]. The cells embedded in the polymer network can freely interact with the surrounding materials through interconnected submicron-sized pores. The main goal of microporous scaffold engineering is to create a semipermeable membrane that is capable of keeping the cells embedded securely while allowing oxygen and nutrients to enter freely and allowing proteins and wastes produced by the cells to leak out [49]. To achieve this intricate balance in mass transportation, a variety of biomaterial matrices (e.g. alginate and polyesters) and strategies have been proposed to fine-tune the properties of microporous scaffolds [50]. Fine-tuning of the initial polyethylene glycol adipate/hyaluronic acid (PEGdA/HA) concentration and maintenance of stiffness close to the normal liver parenchymal part would provide hepatocytes with prolonged functionally favorable microenvironments. In turn, rapid degradation of the HA-enriched zone in semi-interpenetrating networks (semi-IPNs) by enzymatic activity and ECM formation by supporting cells can lead to stiffness changes. As the flexibility of 3D liver model close to liver, the cells presented the mature phenotype and function, including the hepatic gene expression, albumin secretion, cytochrome P450 activity, and drug metabolism [30].
Macroporous scaffolds.
Comparing to the micropores, macropores have better permeability and more space/interface for exchange nutrients and oxygen, cell adhesion, proliferation, and ECM deposition [48].
Chitosan gelatin (CG) is a conventional material used to form macroporous scaffolds through a freeze-drying technique. By adjusting the concentration of these components, the pore size, stiffness, degradation rates, and mechanical integrity can be easily controlled to enable cell engraftment and proliferation [51,52]. Heparan sulfate or hyaluronic acid is generally incorporated in the fabrication of hydrogels to make the surface more conducive to cell adhesion, as well as for good degradation characteristics that allow fibroblasts to synthesize the matrix to rebuild the scaffolds [53].
Recently, a more sophisticated macroporous scaffold called the inverted colloidal crystal (ICC) scaffold has been developed to better engineer pore size and porosity. The 3D hexagonal porous structure of ICC resembles the anatomy of the liver lobule [54]. The internal geometry of the ICC enables the spontaneous formation of heterotypic liver spheroids within each cavity and interconnected cell growth across adjacent cavities [55,56]. This engineered human liver tissue provided proof-of-concept determination of human-specific drug metabolism, retained the ability to support infection with human hepatitis virus for subsequent antiviral drug testing, and facilitated the detection of human-specific drug hepatotoxicity associated with late-onset liver failure [56].
3D bio-printing.
3D bioprinting has its fascinating advantages over all the conventional tissue engineering approaches. With the help of a computer-aided design (CAD) model, a layer of material is successively added by a robotic arm to create scaffolds with delicate structures such as vasculature-like networks in a convenient, precise, and highly automated manner [57]. There are several 3D printing methods, such as inkjet bioprinting, extrusion-based bioprinting (EBB), and light-assisted bioprinting (LAB), along with digital light processing-based bioprinting and laser-based two-photon polymerization bioprinting. Inkjet bioprinting is a classical method based on heat, piezoelectric, or electromagnetic force. The resolution is relatively low, but it is a relatively quick process, cheaper to assemble and the applications in multiple surgical fields have been explored, such as orthopedics, maxillofacial surgery, cranial surgery, and spinal surgery [58]. EBB is rather slow, and extrusion stress may damage cellular viability [59]. LAB relies on bioink and has an advantage in the micro- and nano-architecture. Cells through the highly precise laser vaporization approach, which is common for scaffolds that require a high level of resolution [60].
Bioink is a critical component for 3D bioprinting. It must be low in viscosity to avoid clotting in the system but not too low that it cannot hold the cells in suspension homogenously. Bioink biocompatibility is also vital because the cells are generally added to bioink and exposed to polymerization [57]. Materials such as alginate, Matrigel, and gelatin methacrylamide have demonstrated their potentials as bioinks for liver cell culture [61]. It is hard to judge which method is best, but the most likely future trend is high-resolution, high-speed organization of cells, low cost and matching various bioinks.
A 3D printing product combined with a perfusion system or bioreactor can form a perfusion culture system or a bioreactor system. It can be used to predict toxicity [61] and demonstrate differentiation in induced pluripotent stem cells (iPSCs) [62].
Vasculature formation
In the absence of flow in the culture system, cells residing at the core of a densely populated 3D structure have the propensity to suffer from necrosis due to insufficient exchange with oxygenated media [63]. To circumvent this limitation associated with static conditions, perfused vascular networks have been proposed to facilitate the transportation of oxygen, nutrients, and waste products in and out of the system [64]. In addition, shear stress associated with flow condition is more in physiological in vivo-like conditions. It has been hypothesized that fluid mechanical stress activates regeneration-related genes in liver progenitor cells [65]. Therefore, much effort has been devoted to the incorporation of vasculature into the 3D liver culture model to introduce the necessary flow and biochemical signals to hepatocytes. Here, we highlight two approaches developed for vasculature formation: artificial blood vessel engineering and cell-driven vasculature formation in the process of organogenesis.
Blood vessel engineering.
Bioprinting is a revolutionary technology to assemble scaffolds for growing tissues. Functional vascularized tissue structure, an artificial physical barrier layer to separate the cell culture area from the microfluidic channel, can be printed directly enabling fluid flow for transport of nutrition, gaseous exchange, and removal of waste [64]. The flow generated by a pneumatic pump along the microfluidic channel diffuses into the hepatocyte cord area via osmosis, mimicking the physiological dynamics observed in the body. A porous polyester membrane was developed that allows seeding of hepatocytes on one side and occupation of the opposite site by NPCs, such as sinusoidal endothelial cells, KCs, and stellate cells [66]. Perfusion bioreactor devices provide a suitable and dynamic environment with continuous flow optimizing the mass transport and exchange and the delivery of oxygen and nutrients. These allow non-invasive monitoring of culture parameters and media sampling [67]. Diverse perfusion bioreactor devices to the culture of hepatocyte spheroids, such as spinning perfusion bioreactor, radial flow bioreactor, multichamber bioreactor have been applied in the drug discovery [68]. Organ-on a-chip models recapitulate the structural, microenvironmental, and physiological functions of human real organs in a chip with the help of microfabrication and microfluidic technology [69]. Fluid flow in organ-on-a-chip models reflects physiological flows, such as blood flow, and tissue functions, such as peristalsis, breathing, and heartbeat [70]. Continuing homogenous media flow experienced by cells of hepatic spheroids can be achieved by the 3D microfluidic system [71]. This setup has successfully sustained the phenotypes of PHH for up to 40 days and could be infected with hepatitis B virus (HBV), becoming an attractive in vitro model for HBV research.
Cell-driven vasculature formation in organoids.
The cell-driven vasculature formation in the process of in vitro organoid generation is an alternative approach to the engineering mentioned above for the generation of functional blood vessels in the 3D model. Leveraging on the advantages of iPSC technology, hepatic dermal cells, progenitors of hepatocytes, were co-cultured with endothelial cells and mesenchymal stem cells (MSCs) on Matrigel-coated surfaces [72]. The MSCs served as the driving force for condensation and folding of the 2D sheet into the 3D liver bud, mimicking the liver bud initiation observed during in vivo fetal liver development. In the process of liver bud formation, human umbilical vein endothelial cells (HUVECs) develop into premature vasculature networks in the condensed organoid. This scaffold-free self-condensation process has been shown to be very robust and compatible with a range of organs in vascularization [72,73]. Upon transplantation, this pre-vasculature liver bud demonstrated superior integration and became functional by connecting with the host blood vessel within 48 hours. The authors also demonstrated the ability to scale up the production of liver buds to serve as a potential high-throughput platform for drug discovery [74].
Cell sourcing.
The phenotypes and functions of hepatocytes in vitro are highly regulated by the seamless synchronization between the cells and culture model. The choice of culture model in association with the specific hepatic cell source is critical for the success of individual hepatic in vitro studies.
PHHs are regarded as the gold standard cell type for in vitro metabolic studies because of their physiologically relevant expression of cofactors, enzymes, and membrane drug transporters [15]. PHHs are also highly sought after in cell therapy because they are not artificially altered by cell culture, which might lead to unwanted genetic mutations that could be potentially cancerous upon implantation. However, inter-individual differences and cell alterations due to the isolation procedure result in inevitable variations in experimental outcomes [17,75,76]. In addition, donor scarcity and challenging logistics in PHH processing render this cell type less viable for large-scale studies and applications. The shelf life of PHHs is another limiting factor because the cells lose metabolic activity within several hours upon seeding onto the 2D culture [44], although the shelf life has been found to be extended to 10 days in a 3D multi-compartment bioreactor [77].
Immortalized cell lines are popular alternatives to PHHs owing to their availability, functional consistency, and in vitro expansion. The high expression of phase II genes makes the HepG2 cell line useful for studying drug metabolism [78]. However, HepG2 cells express very low levels of phase I drug-metabolizing enzymes [74]. The incomplete expression of surface junction proteins restricts hepatitis C virus entry into HepG2, which limits its use in hepatitis exploration [79]. Similar to HepG2, Huh7 cells express Phase I and II xenobiotic drug metabolism genes as well as some critical hepatocyte-specific transcripts [18]. HepaRG is a terminally differentiated hepatic cell line derived from human HCC cell lines. Unlike HepG2 and Huh 7 cell lines, HepaRG cells possess a stable phenotype and functional capacity of Phase I and II xenobiotic-metabolizing enzymes and transporters. HepaRG cells can proliferate, subsequently differentiating into hepatocytes and biliary cells under the appropriate culture conditions [4]. Regardless of its superiority over other cancer cell lines, HepaRG still suffers from subpar sensitivity in predicting drug metabolism and safety [17].
Although the overall development of human liver organs and the underlying stem cell populations that regulate regeneration and homoeostasis have remained elusive, the conventional consensus is that human fetal liver cells comprise two main populations: human hepatic stem cells (hHpSCs) and hepatoblasts [79]. The evidence that hHpSCs yield mature liver tissue after transplantation supports the notion that they are potentially an excellent cell source for establishing long-term culture systems with advanced hepatic functions [80]. However, one big problem is that this cell source is difficult to come by, and the ethical concerns are too much of a hurdle to overcome in obtaining approval from regulatory boards.
The pluripotent stem cell (PSC)-derived hepatocyte approach has garnered significant progress in regenerative medicine over the past decade. iPSCs can be reprogrammed from somatic cells by introducing transcription factors Oct3/4 and Sox2. hESCs derived from the inner cell mass of blastocysts are another stem cell candidate in liver studies. iPSCs and hESCs can proliferate indefinitely without loss of potency and retain the capacity to differentiate into various cells [81]. Nevertheless, hESCs have raised ethical concerns [17], while iPSCs have raised considerable excitement in the field of regenerative medicine because these cells do not rely on the donor or cause life-long immunosuppression. Although various protocols for stem cell differentiation into hepatocytes have been established to date, the generated HLCs are characterized as fetal liver cells with immature phenotypes and reduced hepatic functionality compared to the fully functional PHHs [82]. In addition, due to the lack of standardized criteria and profiling assays, it is difficult to compare the success of different approaches and identify promising modifications that may enhance hepatocyte maturation [78]. All the existing protocols rely on the understanding of embryogenesis in guiding the supplementation of growth factors in the tissue culture dish [83]. Recent studies validated PSC-derived hepatic organoids exhibit self-renewal (expandable and further able to differentiate) while maintaining more mature phenotypes compared with those of 2D differentiated hepatocytes and tissue-derived hepatic organoids [84].
In summary, iPSCs are the most potential cell type for clinical application because of easily being produced, which attract some companies to commercialize iPSC-derived hepatocytes.
Conclusion and Perspective
The liver is the main metabolic organ of the human body, and liver failure will lead to death. Accurate prediction of drug toxicity can help reduce the occurrence of liver injury and maintain the phenotypes and functions of hepatocytes. Though 3D cell culturing models expand the drug database of absorption, distribution, metabolism, and excretion, it seems quite far from clinical application. However, organ-on-a-chip has begun to spawn from academic research to drug discovery industry [69,70]. Large scale national research grants regarding organ-on-a-chip enhanced the expectation for practical use of this technology [68]. One drug through the human body requiring multi-organ participation so that the final goal is to achieve a ‘human-on-a-chip’ that integrates numerous organs into a single chip [69,85]. The formation of liver organoids, such as bioartificial liver (BAL) system [86] and injectable hydrogels for cell therapy, can help treat hepatocyte necrosis diseases and enhance tissue regeneration because of the minimally invasive nature of their application surgical procedures, ease of handling, and complete filling of the defect area [87]. Organoids transplanted into animals have demonstrated a rescue effect in acute liver failure and restored critical liver function.
We have stated various advantages of the 3D cell culture and some components of the 3D cell culture system. Therefore, we have reason to believe that 3D hepatocyte culture is a feasible and promising method of drug metabolism and pharmacokinetics research.
Funding
This work was supported by the grant from the National Natural Science Foundation of China (No. 82070615).
Conflict of Interest
The authors declare that they have no conflict of interest.