-
PDF
- Split View
-
Views
-
Cite
Cite
Luyao Chen, Yaping Guo, Zixuan Wu, Shuwu Zhao, Zhaiyi Zhang, Fang Zheng, Likang Sun, Zheng Hao, Chen Xu, Tao Wang, Yanfei Peng, Epicatechin gallate prevents the de novo synthesis of fatty acid and the migration of prostate cancer cells, Acta Biochimica et Biophysica Sinica, Volume 53, Issue 12, December 2021, Pages 1662–1669, https://doi.org/10.1093/abbs/gmab144
- Share Icon Share
Abstract
Lipid metabolism disorder caused by the upregulation of lipogenic genes is a typical feature of prostate cancer. The synthesis of fatty acids is enhanced to accelerate the development of prostate cancer and is considered as a potential therapeutic target. Epicatechin gallate, an active compound of green tea, has been reported to modulate lipid metabolism. In this research, the potential role of epicatechin gallate in prostate cancer cells was evaluated. The results indicated that epicatechin gallate downregulates the expression of acetyl-CoA carboxylase, ATP citrate lyase, and fatty acid synthase in prostate cancer cells and prostate xenograft tissues, suggesting that epicatechin gallate can inhibit de novo fatty acid synthesis. Moreover, epicatechin gallate significantly restrains the migration rather than the viability of prostate cancer cells. PI3K/AKT/mTOR signaling pathway, which exhibits regulatory effect on lipogenesis, is also inhibited under epicatechin gallate treatment, while pretreatment with AKT activator SC79 or mTOR activator MHY1485 blocks the inhibitory effect of epicatechin gallate on the expression of lipogenic genes and the migration of prostate cancer cells. In conclusion, this study revealed that epicatechin gallate impairs the synthesis of fatty acids via inhibition PI3K/AKT/mTOR signaling pathway and then attenuates the migration of prostate cancer cells.
Introduction
Prostate cancer is recognized as the second most common malignant tumor with the fifth foremost etiology of cancer-related death among males worldwide [1]. Deregulation of lipid metabolism contributes to the pathologic progression and has been recognized as a typical feature of prostate cancer [2]. Lipogenesis is increased in prostate cancer due to the upregulated expression of lipogenic genes [3]. Restraining the lipogenesis may be a potential therapeutic strategy in prostate cancer treatment [4–6].
ATP citrate lyase (ACLY) serves as the key enzyme to catalyze the formation of cytosolic acetyl-CoA in lipogenesis. Acetyl-CoA carboxylase (ACC) catalyzes the carboxylation of acetyl-CoA to malonyl-CoA, which is the first and rate-limiting reaction in de novo fatty acid synthesis. Another enzyme, fatty acid synthase (FASN), is responsible for the synthesis of palmitate from acetyl-CoA and malonyl-CoA [7]. The transcription of these key lipogenic genes (ACC, ACLY, and FASN) is activated by the pivotal transcription factor—sterol regulatory element-binding proteins (SREBPs), which comprise three main proteins (SREBP-1a, SREBP-1c, and SREBP-2) encoded by two genes SREBP1 and SREBP2 [8]. The SREBPs are synthesized as inactive precursor proteins and can be proteolytic cleavage to free N-terminal domain of SREBPs, which then move to the nucleus and bind to specific sterol regulatory elements (SREs) in the promoter region of lipogenic genes to activate transcription. This process is termed the maturation of SREBPs [9]. In prostate cancer, oncogenic activation of PI3K/AKT/mTOR signaling is a frequent event and facilitates the progression of the disease [10]. Several reports have indicated that the PI3K/AKT/mTOR signaling pathway regulates the maturation of SREBPs and SREBP-mediated lipogenesis [9,11,12]. Epicatechin gallate (ECG) belongs to green tea polyphenols and exhibits an anti-tumor activity. In a previous report, ECG was found to inhibit the progression of prostatic tumorigenesis by diminishing inflammation [13]. Furthermore, the combination of ECG and other polyphenols (curcumin and resveratrol) display a stronger anti-tumor effect in cervical cancer cells [14]. Moreover, ECG can regulate lipid metabolism and was reported to reduce the de novo synthesis of fatty acids in colon cancer cells [15]. However, it is still ambiguous if ECG can inhibit the progression of prostate cancer via the modulation of lipid metabolism.
In this research, we evaluated the potential effect of ECG in prostate cancer and revealed that ECG decreases the synthesis of fatty acids and the migration of prostate cancer cells by inhibiting PI3K/AKT/mTOR signaling pathway.
Materials and Methods
Cells and reagents
Prostate cancer cell lines PC3 and 22RV1 were kindly provided by Life College of Nankai University (Tianjin, China) and cultured in RPMI 1640 medium (Sigma, St Louis, USA) supplemented with 10% fetal bovine serum (FBS; Lonsera Science SRL, Canelones, Uruguay). ECG was purchased from Standard Technology (Shanghai, China). SC79 and MHY1485 were purchased from Med Chem Express (New Jersey, USA).
Real-time reverse transcriptase-polymerase chain reaction
PC3 and 22RV1 cells were treated with vehicle dimethyl sulfoxide (DMSO) or different doses of ECG (1, 10, and 100 μg/l) for 24 h. Total RNA was extracted using Trizol reagent (Invitrogen, Carlsbad, USA). Reverse transcription was performed with a commercial reverse transcription kit (Promega, Madison, USA). Real-time polymerase chain reaction (PCR) was performed and primers are listed in Table 1. The relative gene expression was determined using the comparative computed tomography method and normalized to housekeeping gene hypoxanthine phosphoribosyl transferase 1 (HPRT). All the experiments were performed three times, and the results were expressed as the mean ± standard deviation (SD).
Primer . | Sequence (5ʹ → 3ʹ) . |
---|---|
Human HPRT Forward | TGACACTGGCAAAACAATGCA |
Human HPRT Reverse | GGTCCTTTTCACCAGCAAGCT |
Human ACC Forward | AACCGGAAATCTGAACGG |
Human ACC Reverse | TCTCGGAGGGCGAATACA |
Human ACLY Forward | ACGGATGGCGTCTATGAG |
Human ACLY Reverse | GCAGACGATGGGCTTAGTG |
Human FASN Forward | CGCGTGGCCGGCTACTCCTAC |
Human FASN Reverse | CGGCTGCCACACGCTCCTCT |
Primer . | Sequence (5ʹ → 3ʹ) . |
---|---|
Human HPRT Forward | TGACACTGGCAAAACAATGCA |
Human HPRT Reverse | GGTCCTTTTCACCAGCAAGCT |
Human ACC Forward | AACCGGAAATCTGAACGG |
Human ACC Reverse | TCTCGGAGGGCGAATACA |
Human ACLY Forward | ACGGATGGCGTCTATGAG |
Human ACLY Reverse | GCAGACGATGGGCTTAGTG |
Human FASN Forward | CGCGTGGCCGGCTACTCCTAC |
Human FASN Reverse | CGGCTGCCACACGCTCCTCT |
Primer . | Sequence (5ʹ → 3ʹ) . |
---|---|
Human HPRT Forward | TGACACTGGCAAAACAATGCA |
Human HPRT Reverse | GGTCCTTTTCACCAGCAAGCT |
Human ACC Forward | AACCGGAAATCTGAACGG |
Human ACC Reverse | TCTCGGAGGGCGAATACA |
Human ACLY Forward | ACGGATGGCGTCTATGAG |
Human ACLY Reverse | GCAGACGATGGGCTTAGTG |
Human FASN Forward | CGCGTGGCCGGCTACTCCTAC |
Human FASN Reverse | CGGCTGCCACACGCTCCTCT |
Primer . | Sequence (5ʹ → 3ʹ) . |
---|---|
Human HPRT Forward | TGACACTGGCAAAACAATGCA |
Human HPRT Reverse | GGTCCTTTTCACCAGCAAGCT |
Human ACC Forward | AACCGGAAATCTGAACGG |
Human ACC Reverse | TCTCGGAGGGCGAATACA |
Human ACLY Forward | ACGGATGGCGTCTATGAG |
Human ACLY Reverse | GCAGACGATGGGCTTAGTG |
Human FASN Forward | CGCGTGGCCGGCTACTCCTAC |
Human FASN Reverse | CGGCTGCCACACGCTCCTCT |
Western blot analysis
PC3 and 22RV1 cells were treated with vehicle or different doses of ECG (1, 10, and 100 μg/l) for 48 h. Total proteins were extracted with radio immunoprecipitation assay (RIPA) buffer (CWBIO, Beijing, China) and then western blot analysis was performed. The primary antibodies were anti-ACC antibody (Abcam, Cambridge, UK), anti-ACLY antibody (Abcam), anti-FASN antibody (Santa Cruz, Santa Cruz, USA), and anti-β-actin antibody (BIOSS, Beijing, China). Secondary antibodies (goat anti-rabbit or goat anti-mouse) conjugated with horseradish peroxidase (HRP) were purchased from Proteintech (Rosemont, USA).
Cells were treated with vehicle or 100 μg/l ECG for 18 h to detect PI3K/AKT/mTOR signaling pathway and the maturation of SREBPs. The anti-AKT antibody, anti-phosphorylated AKT (p-AKT) antibody, anti-mTOR antibody, anti-p-mTOR antibody, and anti-SREBP1 antibody were purchased from BIOSS. Anti-SREBP2 antibody was purchased from Abcam. All the results were obtained from three independent experiments.
Cell viability
PC3 and 22RV1 cells were seeded into 24-well plates (5 × 104 cells/well) and treated with vehicle or 100 μg/l ECG for 0, 24, 48, and 72 h. Then CCK8 kits (US Everbright INC, Suzhou, China) were used to evaluate the viability of cells according to the instructions of the manufacturer. The results were expressed as the mean ± SD of triplicates.
Wound healing assays
PC3 and 22RV1 cells were cultured in 6-well plates. Each well was scratched with disposable tips in the cell monolayer. Then, PC3 cells were treated with vehicle or 100 μg/l ECG for 24 h, and 22RV1 cells were treated with vehicle or 100 μg/l ECG for 48 h. Images were taken at 100× magnification under a phase-contrast microscope (Leica, Heidelberg, Germany). Image J software was used to analyze the relative migration distance. The results were expressed as the mean ± SD of triplicates.
Transwell assays
PC3 and 22RV1 cells were treated with vehicle or 100 μg/l ECG for 48 h. Then, cells were collected and counted, followed by transwell assays performed as previous report [16]. The cells were seeded in transwell inserts with 8 μm pore size (BD Biosciences, San Diego, USA) at 5 × 104 cells per well in RPMI 1640 medium supplemented with 10% FBS. After 24 h, cells on the upper surface of the filter were removed using a cotton swab. Cells that had migrated through the filter to the lower surface were fixed with 4% paraformaldehyde and stained with 0.5% crystal violet. Images were taken at 100× magnification under a phase-contrast microscope and cells were counted from five randomly selected fields per chamber.
Treatment with SC79 or MHY1485
PC3 and 22RV1 cells were pretreated with DMSO, 10 μM SC79, or 10 μM MHY1485 for 30 min. Then the cells were treated with vehicle or 100 μg/l ECG for another 48 h. Total protein was extracted and western blot was performed to detect the expressions of ACC, ACLY, and FASN. The cells were also collected and counted, then transwell assays were performed, and the photographs were taken at a magnification of 100×. All the results were obtained from three independent experiments.
PC3 xenograft model
The protocol for in vivo studies was approved by the Ethics Committee of Tianjin University of Traditional Chinese Medicine and conformed to the Guide for the Care and Use of Laboratory Animal published by the National Institutes of Health (eighth edition, updated 2011). Fourteen Balb/c nude mice (male, 6 weeks old) were purchased from Huafukang Biotechnology Company (Beijing, China). The mice were acclimated in specific pathogen-free units for 1 week, followed by subcutaneous injection with 5 × 106 PC3 cells on the right side of axillary. The mice were normally fed for another 2 weeks and divided into two groups: control group (seven mice) and ECG treatment group (seven mice). Daily gavage with phosphate buffer saline (PBS) was performed in control group and daily gavage with ECG (40 mg/kg weigh) was performed in ECG treatment group. After the administration of PBS or ECG, the body weights of both groups were monitored at different time points. After two more weeks, the mice were sacrificed and the xenografts were isolated for immunohistochemistry (IHC) staining and western blot analysis.
IHC staining
The xenografts isolated from mice were embedded and made into frozen sections. The sections were fixed and sealed with phosphate buffer supplemented with 10% FBS. The primary antibodies for IHC assays were anti-ACC and anti-ACLY antibodies (both from BIOSS) and anti-FASN antibody (Santa Cruz). Secondary antibodies (goat anti-rabbit or goat anti-mouse) conjugated with Biotin and streptomycin conjugated with HRP were purchased from BIOSS. DAB developer (Solarbio, Beijing, China) was utilized to detect the expression of corresponding proteins, and hematoxylin was used to stain the nucleus. The pictures were taken with a phase-contrast microscope (Leica) at 200× and 400× magnifications.
Statistical analysis
All statistical analysis was performed using SPSS software (version 23.0; IBM Corp., Armonk, USA). Data are presented as the mean ± SD. Analysis of t-test was used to analyze the data in two groups. Variance analysis was performed in multiple groups. P < 0.05 was considered to be statistically significant.
Results
ECG inhibited the expression of ACC, ACLY, and FASN
PC3 and 22RV1 were treated with different doses of ECG (0, 1, 10, and 100 μg/l) for 24 h, and the messenger RNA (mRNA) expression of ACC, ACLY, and FASN was detected by real-time reverse transcriptase-PCR. The results indicated that ECG treatment significantly decreased the mRNA expressions of ACC, ACLY, and FASN in the cells (Fig. 1A). Western blot analysis also indicated that ECG treatment decreased the protein expressions of ACC, ACLY, and FASN (Fig. 1B,C).
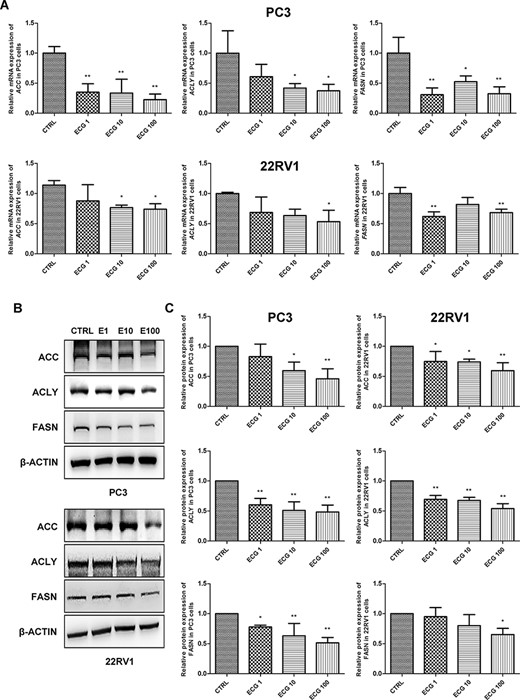
ECG inhibited the expression of lipogenic genes in prostate cancer cells (A) Real-time reverse transcriptase-PCR analysis of the mRNA expressions of ACC, ACLY and FASN in prostate cancer cells treated with different doses of ECG. (B) Western blot analysis was performed to determine the protein expressions of ACC, ACLY and FASN in prostate cancer cells treated with different doses of ECG. (C) Quantitative results of the western blots. *P < 0.05, **P < 0.01.
ECG inhibited the migration rather than the viability of prostate cancer cells
CCK8 assays were performed to detect cell viability, and the results indicated that 100 μg/l ECG did not affect the viability of PC3 and 22RV1 cells (Fig. 2A). Then, the migration of prostate cancer cells was investigated and wound healing assays were performed. Treatment with 100 μg/l ECG inhibited the migration of PC3 and 22RV1 cells (Fig. 2B). Transwell assays confirmed the restrained effects of ECG on the migration of prostate cancer cells (Fig. 2C).
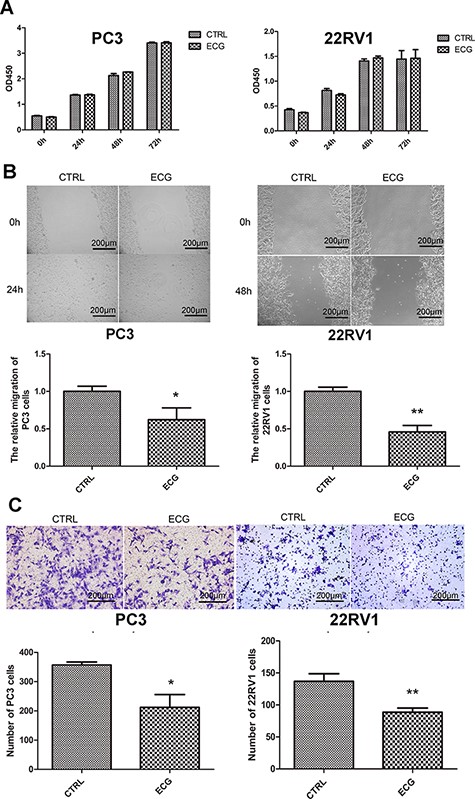
ECG inhibited the migration of prostate cancer cells (A) CCK8 assays showed that 100 μg/l ECG had no regulatory effect on the viability of PC3 and 22RV1 cells. (B) Wound healing assays indicated that 100 μg/l ECG decreased the migration of PC3 and 22RV1 cells. (C) Transwell assays confirmed the inhibitory effect of ECG on the migration of prostate cancer cells. All the photographs were taken at the magnification of 100 ×. *P < 0.05, **P < 0.01.
ECG decreased de novo fatty acid synthesis and migration of prostate cancer cells by inhibiting PI3K/AKT/mTOR signaling pathway
PI3K/AKT/mTOR signaling pathway modulates various biological processes, including migration and lipogenesis in cancer cells. We evaluated the regulatory effect of ECG on this signaling pathway in PC3 and 22RV1 cells. Western blot analysis results showed that treatment with ECG for 18 h significantly inhibited the phosphorylation of AKT and mTOR, suggesting the inhibitory effect of ECG on PI3K/AKT/mTOR signaling pathway (Fig. 3). Furthermore, we detected the maturation of the pivotal transcript factors SREBPs, which can be cleaved to maturation and subsequently enhance the transcription of lipogenic genes. The expression of precursor SREBP-1 (P-SREBP1) and SREBP-2 (P-SREBP2) was not affected by ECG treatment, while the maturation of SREBPs (M-SREBP1 and M-SREBP2) was inhibited (Fig. 3).
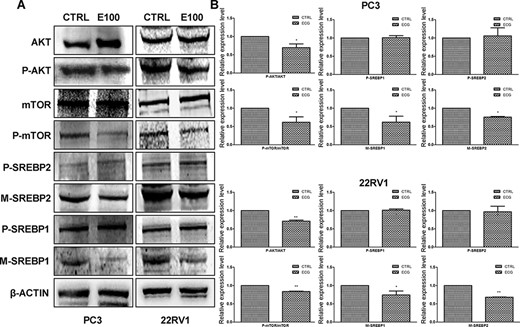
ECG inhibited PI3K/AKT/mTOR signaling pathway and the maturation of SREBPs (A) Western blot analysis was performed to evaluate the effects of ECG. (B) Quantitative results of the western blots. *P < 0.05, **P < 0.01.
The activators of AKT (SC79) and mTOR (MHY1485) were used to pretreat prostate cancer cells. As shown in the results, pretreatment with SC79 or MHY1485 blocked the inhibitory effect of ECG on the expressions of ACC, ACLY, and FASN (Fig. 4A,B). These results indicated that ECG decreased the expression of lipogenic genes via inhibiting the PI3K/AKT/mTOR signaling pathway. Furthermore, pretreatment with SC79 and MHY1485 also antagonized the inhibitory effects of ECG on the migration of prostate cancer cells (Fig. 4C,D).
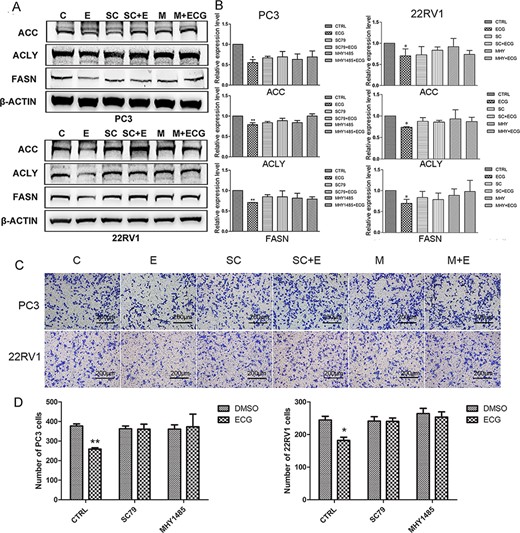
ECG inhibited the expression of lipogenic genes and the migration of prostate cancer cells via inhibition PI3K/AKT/mTOR signaling pathway (A) Pretreatment with SC79 or MHY1485 blocked the inhibitory effect of ECG on the expressions of ACC, ACLY and FASN. (B) Quantitative results of the western blots. (C) Pretreatment with SC79 or MHY1485 blocked the inhibitory effect of ECG on the migration of PC3 and 22RV1 cells. (D) Quantitative results of the transwell assays. All the photographs were taken at the magnification of 100×. C, CTRL; E, ECG; SC, SC79; M, MHY1485. *P < 0.05, **P < 0.01.
ECG inhibited the expressions of ACC, ACLY, and FASN in xenograft tissues
The xenograft model was established by subcutaneous injection of PC3 cells on the right side of the axillary. Two weeks after cell injection, the gavage with PBS or ECG (40 mg/kg mouse weight) was daily performed for another 2 weeks. During treatment, the body weights of mice decreased, but no significant difference was observed between the two groups (Fig. 5A). Then, the mice were sacrificed and the tumors were isolated. There was no significant change in tumor sizes between the two groups (Fig. 5B). These results indicated that ECG had no inhibitory effects on the proliferation of cancer cells. IHC assays were performed to evaluate the expressions of ACC, ACLY, and FASN in xenograft tissues, and the results indicated that ACC, ACLY, and FASN were downregulated in the tissues isolated from the ECG-treated group (Fig. 5C). Total protein was extracted from xenograft tissues, and western blot analysis was performed, and the results confirmed that ECG decreased the expressions of ACC, ACLY, and FASN in xenograft tissues (Fig. 5D,E).
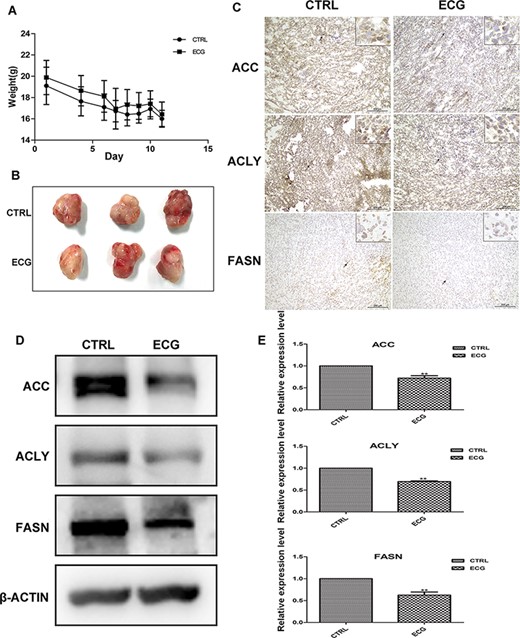
ECG down regulated the expression of lipogenic genes in PC3 xenograft tissue (A) The body weights of the two groups were monitored during the treatment. (B) The tumor tissues isolated from control group and ECG-treated group. (C) IHC assays of the expressions of ACC, ACLY and FASN in xenograft tissues. Black arrows indicate the positively stained cells. All the photographs were taken at the magnification of 200× and the boxes at the upper left corner are at 400× magnification. (D) Western blot analysis of the expressions of ACC, ACLY and FASN in xenograft tissues. (E) Quantitative results of the western blots. **P < 0.01.
Discussion
The disorder of lipid metabolism has been considered as a potential therapeutic target in prostate cancer treatment [17]. ACC, ACLY, and FASN are the critical lipogenic enzymes regulating the synthesis of fatty acids. Previous report indicated that RNA interference-mediated silencing of ACC inhibits the growth of prostate cancer cells and induces their apoptosis [18]. A similar report indicated that miR-22 attenuates the proliferation and invasion of prostate cancer cells by inhibiting ACLY in vitro and in vivo [19]. FASN has attracted the most attention since its upregulation was observed in both androgen-dependent and androgen-independent prostate cancer cells. Orlistat, a commonly used inhibitor of FASN, has been reported to increase the sensitivity of prostate cancer to chemotherapy and radiotherapy [20–22]. However, the inhibitors of lipogenic enzymes have not been widely used in the clinical treatment of prostate cancer. In this research, we found that ECG downregulated the expressions of ACC, ACLY, and FASN in prostate cancer cells both in vitro and in vivo, indicating the inhibitory effect of ECG on de novo fatty acid synthesis.
Green tea catechins have been widely studied and considered to be beneficial in the treatment of prostate cancer [23]. Among the catechins, epigallocatechin-3-gallate (EGCG) has been widely studied. EGCG was reported to inhibit the carcinogenesis of prostate cancer [24], while the effect of ECG has not been fully clarified. Previous research indicated that ECG induced the apoptosis of prostate cancer cell DU145 [25], but had no inhibitory effect on the growth of human prostate tumors in athymic mice, suggesting that ECG could not prevent the growth of prostate xenograft in vivo [26]. In our research, the viability of PC3 and 22RV1 cells was not regulated by ECG treatment. However, we discovered that treatment with ECG inhibited the migration of prostate cancer cells, suggesting that ECG may suppress the progression of prostate cancer by preventing metastasis.
SREBP1 and SREBP2 act as the pivotal transcription factors in lipogenesis and cholesterol synthesis and are considered as potential therapeutic targets in prostate cancer [3]. Precursor SREBPs are activated by cleavage, and the N-terminal peptide is translocated into the nucleus to activate the transcription of lipogenic genes, and this process is termed maturation of SREBPs [27,28]. Huang et al. [29] reported that the expression of SREBP1 is positively associated with the clinical Gleason grades in human prostate cancer and promotes prostate cancer progression via regulation signaling networks involving androgen receptor, lipogenesis, and reactive oxygen species in prostate cancer cells. Li et al. [30] reported that SREBP2 is elevated in advanced pathologic grade and metastatic prostate cancer and significantly associated with poor clinical outcomes, since SREPB2 promotes stem cell-like properties by upregulating c-myc in prostate cancer cells. These results suggested that inhibiting the expression or maturation of SREBPs may be effective in damping the progression of prostate cancer. In this research, ECG treatment was found to inhibit the maturation of SREBP1 and SREBP2, which may be the reason why ECG inhibits the synthesis of fatty acids.
The deregulation of PI3K/AKT/mTOR signaling pathway has attracted much attention and been considered as an important target for cancer therapy, since it is always associated with cancer progression. In prostate cancer, aberrant activation of the PI3K/AKT/mTOR signaling pathway correlates with the resistance of androgen ablative treatment, the progression to hormone refractory disease and poor outcome, which has been observed in 40% early diagnosed disease and in more than 70% advanced disease [31]. Increasing evidence has pointed out that PI3K/AKT/mTOR signaling pathway may serve as a pivotal regulator in the maturation of SREBPs. Du et al. [32] reported that LY294002, an inhibitor of PI3K, could reverse high glucose-induced increase of matured SREBP-1, ACC, and FASN in HK-2 cells. Yi et al. [12] demonstrated that CCI-779, an inhibitor of mTORC1, decreased the level of mature form of SREBP1 in mammary cancer cell lines. In melanoma cells, both LY294002 and rapamycin have the ability to reduce the mature forms of SREBP-1 and SREBP-2 [11]. All these pieces of evidence supported that the maturation of SREBPs and subsequent lipogenesis are the downstream of PI3K/AKT/mTOR signaling pathway. In this research, we found that PI3K/AKT/mTOR signaling pathway was significantly inhibited by ECG treatment, which could provide the reason of the attenuation of SREBP maturation and the expression of lipogenic genes by ECG.
In conclusion, we investigated the potential effect of ECG on prostate cancer cells and discovered the inhibitory role of ECG in the de novo synthesis of fatty acids and migration of prostate cancer cells, most probably through the inhibition of the PI3K/AKT/mTOR signaling pathway.
Funding
This work was supported by the grants from the National Natural Science Foundation of China (Nos. 81703828, 81873100 and 81673732), and the research and innovation project of graduate students in the Tianjin University of Traditional Chinese Medicine (No. YJSKC-20191023).
Conflict of Interest
The authors declare that they have no conflict of interest.