-
PDF
- Split View
-
Views
-
Cite
Cite
Hannah Scott, Alisha Guyett, Jack Manners, Nicole Stuart, Eva Kemps, Barbara Toson, Nicole Lovato, Andrew Vakulin, Leon Lack, Siobhan Banks, Jillian Dorrian, Robert Adams, Danny J Eckert, Peter Catcheside, Circadian-informed lighting improves vigilance, sleep, and subjective sleepiness during simulated night-shift work, Sleep, Volume 47, Issue 11, November 2024, zsae173, https://doi.org/10.1093/sleep/zsae173
- Share Icon Share
Abstract
Shiftwork is associated with cognitive impairment and reduced sleep time and quality, largely due to circadian misalignment. This study tested if circadian-informed lighting could improve cognitive performance and sleep during simulated night shifts versus dim control lighting.
Nineteen healthy participants (mean ± SD 29 ± 10 years, 12 males, 7 females) were recruited to a laboratory study consisting of two counterbalanced 8-day lighting conditions (order randomized) 1-month apart: (1) control lighting condition - dim, blue-depleted and (2) circadian-informed lighting condition - blue-enriched and blue-depleted where appropriate. Participants underwent an adaptation night (22:00–07:00 hours), then four nights of simulated night work (cognitive testing battery of nine tasks, 00:00–08:00 hours), and sleep during the day (10:00–19:00 hours). Psychomotor vigilance task (PVT) lapses, Karolinska Sleepiness Scale (KSS) scores, and polysomnography-derived sleep outcomes were compared between conditions and across days using mixed models.
Significant condition-by-day-by-time of task interaction effects were found for PVT lapses, median reaction time, and reaction speed, with ~50% fewer lapses by the end of simulated shift work with circadian-informed lighting versus control (mean ± SD 7.4 ± 5.0 vs. 15.6 ± 6.1). KSS was lower around the night shift midpoints on days 6 and 7 with circadian versus control lighting. Participants slept 52 minutes longer [95% CIs: 27.5, 76.5 minutes] by day 7 with circadian-informed versus control lighting, p < .001. Effects were inconsistent on other performance tasks.
Circadian-informed lighting improved sleep, sleepiness, and vigilance compared to control lighting. These findings support the potential for lighting interventions to improve sleep and vigilance in night shift workers chronically exposed to dim lighting.
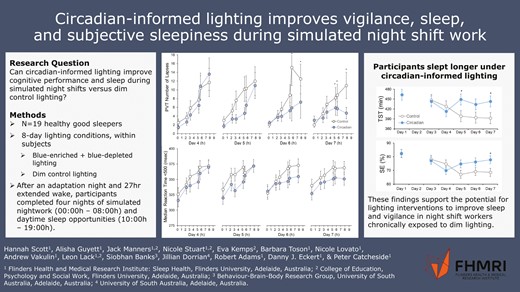
Shift work can lead to impaired cognitive performance and disrupted sleep due to the commonly experienced misalignment between the shift work schedule and circadian rhythms (“body clocks”). Light is one of the most potent methods to help retime circadian rhythms. This study found that a new overhead lighting strategy helped accelerate circadian realignment to night shift work conditions, which in turn boosted vigilance and sleep. These findings highlight the potential for lighting interventions to help enhance worker performance, bolster overall safety, and mitigate health risks, particularly in workplace settings with chronic dim lighting such as those found across healthcare, manufacturing, and defense industries.
Cognitive performance and sleep under night shift work conditions are typically impaired compared to day work conditions [1, 2]. A leading underlying cause of these impairments is circadian misalignment [3]: de-synchrony between the timing of the circadian system and the night shift work schedule. Cognitive performance is affected by circadian timing, with the poorest cognitive performance typically occurring around the circadian nadir in core body temperature (04:00–05:00 am in a normally entrained individual) [4]. Circadian rhythms also regulate sleep, which in turn, affects cognitive performance [5]. Accordingly, circadian misalignment will impair sleep and cognitive performance during the night shift, contributing to poorer work performance and increased risks of work errors and accidents, including fall-asleep events. Consequences can be catastrophic in high-risk shift-work settings such as hospitals [6, 7], transport [8, 9], and military operations [10, 11], where sustained wake and vigilance are critical. While pre-disposing resilience and training to adapt to sleep loss may partially mitigate some of the consequences of poor sleep [12], the biological imperative for sleep and the nature of circadian regulation of cardiometabolic and cellular functions dictate that chronic poor sleep and circadian misalignment will inevitably impair performance, safety, and health in any individual.
Given the profound influence of the circadian system on sleep and cognitive performance, interventions that support circadian adjustment to night-shift work may be amongst the most effective strategies to improve cognitive performance and sleep. Light is the strongest stimulus to the circadian timing system and blue-enriched light also has direct alerting effects [13]. Hence, lighting interventions designed to promote alertness and facilitate circadian adjustment are one of the most promising strategies to enhance cognitive performance during night-shift work [14]. Characteristics of light exposure, including spectral features, timing, and brightness all importantly influence circadian system responses to light [15–18]. Bright, blue-enriched light exposure before the core body temperature minimum promotes circadian delay, while administration after the core body temperature minimum promotes circadian advance, with maximal re-timing effects occurring 2–3 hours on either side of this transition point [19]. In contrast, dim, blue-depleted light produces minimal circadian effects and can therefore be used to provide lighting at times when a phase-shifting effect is undesirable. A recent test of a sleep opportunity timing-dependent, blue-enriched lighting strategy found large circadian phase delays on blood and saliva melatonin markers compared to a constant illuminance control (~100 lux, similar to office lighting), supporting that continuous overhead lighting can be an effective strategy for promoting rapid circadian phase delay [20]. Lighting interventions that manipulate lighting characteristics 24/7 therefore appear to be useful to directly promote alertness and facilitate circadian timing adjustments to the night shift work schedule, to potentially further improve sleep, subjective alertness, and cognitive performance.
Shift workers in enclosed environments are a high-value target population for a circadian-informed lighting intervention. The overhead lighting in some complex work environments is typically very dim (<10 lux) and sometimes blue-depleted at nighttime in certain areas to assist with dark adaptation. This is especially the case for submarines, where the dim, blue-depleted lighting helps preserve control room periscope operator night-vision and conserves power. Circadian adjustment is likely to be especially challenging in these work environments. In a recent 67-day long field study of a small group of submariners on a 6-hour on /6-hour off-duty watch schedule [21], salivary melatonin profiles showed no evidence of circadian re-adjustment to the constant new shift work schedule in crews of either day or night-shift rotations. This may be due to the complexities of circadian adjustment with a 6-hour on/6-hour off-watch schedule, or the dimly lit and blue-depleted lighting conditions, or both. These submariners slept on average ~5.5 hours during the night shift rotation and 6.4 hours during the day shift rotation across each 24-hour period, split into a main sleep period and a nap. Given the known cumulative effects of sleep loss on cognitive performance [12, 22], this level of chronic sleep restriction is expected to markedly impair vigilance. Consistent findings of chronic poor sleep in shift workers in the military [23–25], transport [26, 27], and mining [28, 29] suggest that poor sleep and circadian dysregulation are major chronic problems negatively impacting performance capability and safety.
The fundamental importance of environmental light exposure to the circadian system means that a lighting intervention should be an effective strategy for driving faster circadian adjustments toward better cognitive performance and sleep under night shift work conditions [14]. Recent advances in light emitting diode (LED) technology have provided much greater automatable control over lighting, enabling customization of 24/7 lighting spectra and intensity schedules beyond what is possible with traditional lights. We recently showed that a blue-enriched lighting strategy dependent upon predicted core body temperature minimum timing (i.e. reliant upon the phase response curve to light) rapidly accelerated circadian adjustment to night shift work, resulting in an approximately 3-fold faster circadian phase delay compared to control lighting [30]. The present study investigated whether this faster circadian adjustment with the circadian lighting intervention also improved simulated on-shift cognitive performance and off-shift sleep compared to lighting conditions similar to submariner and other dimly-lit shift work environments.
Methods
Study design
This study used a randomized controlled, within-participants, cross-over design to examine the effect of circadian-informed versus control lighting on cognitive performance and sleep following the transition to a simulated 4-night shift work schedule. Participants completed one lighting condition during each laboratory visit (in random allocation order), with a 4-week washout period between visits. Minimization (via MinimPy version 0.3 software) [31] using a biased coin approach (0.7 base probability, marginal balance, 1:1 allocation ratio) to help balance condition order between laboratory visit groups for potentially confounding characteristics, namely group size (≤3 or >3), the proportion of females (≤50% or >50%), and median age (≤25 or >25 years). The primary cognitive performance outcome was the number of psychomotor vigilance task (PVT) lapses (>500 milliseconds), recorded during six PVT tests over each night shift. The primary sleep outcome was polysomnography-derived total sleep time, assessed during the daytime sleep opportunities between each night shift. The study was approved by the Southern Adelaide Clinical Human Research Ethics Committee (protocol number: 86.20 HREC/20/SAC/91) and conducted at the Adelaide Institute for Sleep Health (Adelaide, Australia) from February 2020 to August 2022.
Participants
Participants were recruited via the Adelaide Institute for Sleep Health research volunteer registry, online and social media platforms, posters on University noticeboards, and word of mouth. After expression of interest, potential participants completed a screening questionnaire to assess eligibility, which included a general health screen, the Insomnia Severity Index [32], the Pittsburgh Sleep Quality Index (PSQI) [33], Morningness–Eveningness Questionnaire (MEQ) [34], and Epworth Sleepiness Scale [35].
The eligibility criteria were partly based on Australian Defense Force Navy entry requirements and designed to recruit healthy, good sleepers, to first test the effectiveness of the lighting interventions with minimal risk of confounding from potential chronic shift work effects. The specific criteria were: (1) ≥18 years old, (2) self-rated normal health and fitness (via general health screen), (3) body mass index between 18.5 and 32.9 kg/m², (4) reported habitual total sleep time of 7–9 hours on the PSQI, (5) reported typical bedtime between 22:00 and 00:30 and wake up time between 07:00 and 09:00 on the PSQI, (6) intermediate chronotype, with a MEQ score between 12 and 17, (7) no self-reported symptoms of a sleep disorder (ISI, PSQI, ESS), (8) no shift work or international travel (>2 hours time zone shift) in the past 6 months, (9) no language difficulties that may preclude informed consent, (10) no cognitive impairment, mental illness, or intellectual disability, (11) no history of psychiatric, neurological, heart, or lung disease/disorder, (12) no significant eye or cervical spine related disease, disorder or surgery, (13) nonsmoker and no excessive alcohol (<10 standard drinks/week) or caffeine consumption (<250 mg/day).
Laboratory conditions
Participants remained in the sleep laboratory throughout each 8-day study protocol, under tightly controlled conditions including temperature control (~21°Celsius, ~70°Fahrenheit). Participants spent most of their time in one of the six laboratory bedrooms, including during the simulated night shifts, except for short periods with the freedom to move from their bedroom to a nearby lounge room for meals. Participants were allocated to the same bedroom for both laboratory visits. Throughout the laboratory visit, caffeine, alcohol, and nicotine consumption was not permitted and mobile phone access was restricted to allocated rest periods and only with the phone screen on the lowest light setting. Adherence with study procedures was carefully monitored by staff via video feed to a central control room.
Lighting conditions
Further details regarding lighting conditions are reported elsewhere [30]. In brief, both lighting conditions were administered using wall-mounted LED lights (Philips Hue) to deliver automated changes to the lighting according to a pre-determined schedule, supplemented with the existing in-built overhead lighting in the sleep laboratory where needed. In-built overhead lights were not automatable, so remained under select study staff control to boost or reduce light intensity according to the pre-determined schedule. Other staff and participants remained blinded to lighting conditions: all lighting appeared white, and all automated changes in lighting were gradual (occurring over 5–15 minutes), such that transitions were not visually perceptible. The lighting levels in both conditions were monitored regularly (≥4 times every 24 hours) via a light spectrometer by selected study staff and adjusted on rare occasions (<7% of checks) if required.
The control condition was designed to simulate lighting in a submarine control room (~7 lux, ~2400 K), off-shift recreational (15–20 lux, ~3100 K), and sleep environments (<1 lux, or <10lux if greater light levels were needed, i.e. for bathroom breaks). The circadian-informed lighting condition was designed to maximally delay the circadian rhythm based on a recent model of light exposure and circadian system effects on PVT performance [36]. Bright (>300 lux), blue-enriched white lighting was administered at times predicted to produce strong circadian delay, and dim (<10 lux), blue-depleted white lighting was administered at other times. Given anticipated daily circadian timing changes, light exposure timing changes were also adjusted daily.
Study measures
Cognitive performance.
All cognitive performance tasks were administered via Inquisit 6 software (Millisecond) on dual 23-inch monitors (Dell) using high-performance computers (Dell OptiPlex 7080 MT XCTO) with mechanical keyboards (Alienware 310K Mechanical Gaming Keyboard). Table 1 lists the outcome variables for all cognitive performance tasks, and Supplementary Table S1 describes the instructions to participants for each task (Supplementary File). These cognitive performance tasks were chosen based on consultation with submariners: the primary target population of the research funding call. Submariners working in the control room of submariners are often required to maintain a high level of vigilance and memory to encode and retrieve information. In addition, they are required to switch tasks and make decisions/act quickly when potential threats arise. We chose to test the effect of the lighting conditions on vigilance, working memory, task switching, risky decision-making, and cognitive flexibility in this project given the importance of these cognitive domains to this work context. Nonetheless, these cognitive functions are also crucial across a variety of different shift work occupations, including in healthcare and transportation. The number of lapses on the psychomotor vigilance test (PVT) was the primary outcome of this study given the importance of vigilance across many of these work duties.
Task . | Cognitive domain . | Outcome variables . |
---|---|---|
Psychomotor Vigilance Test (PVT) | Sustained attention | - Number of lapses, defined as reactions ≥500 ms - Median reaction times <500 ms - Reaction speed (1/mean reaction time) - Slowest 10% reaction times. |
Continuous Performance Test [37] | Sustained and selective attention | - Mean reaction time - Proportion correct |
Digit Symbol Substitution Task (DSST) [38] | Working memory | - Number of correctly classified symbols - Number of incorrectly classified symbols |
Operation span [39] | Working memory | - Operation Span score (sum of sets recalled) - Total math errors |
Trail Making Task [40–42] | Task switching | - Number of errors (i.e. mouse tracings through the incorrect number or letter in the sequence) |
Stroop Task [43, 44] | Cognitive flexibility and inhibition | - Mean reaction response time - Proportion correctly classified |
Tower of London [45] | Planning | - Total number of moves - Mean solution time |
Balloon Analogue Risk Task [46] | Decision-making | - Mean balloon pumps - Total balloon pops |
Iowa Gambling Task [47] | Decision-making | - NET of beneficial and detrimental solutions. |
Task . | Cognitive domain . | Outcome variables . |
---|---|---|
Psychomotor Vigilance Test (PVT) | Sustained attention | - Number of lapses, defined as reactions ≥500 ms - Median reaction times <500 ms - Reaction speed (1/mean reaction time) - Slowest 10% reaction times. |
Continuous Performance Test [37] | Sustained and selective attention | - Mean reaction time - Proportion correct |
Digit Symbol Substitution Task (DSST) [38] | Working memory | - Number of correctly classified symbols - Number of incorrectly classified symbols |
Operation span [39] | Working memory | - Operation Span score (sum of sets recalled) - Total math errors |
Trail Making Task [40–42] | Task switching | - Number of errors (i.e. mouse tracings through the incorrect number or letter in the sequence) |
Stroop Task [43, 44] | Cognitive flexibility and inhibition | - Mean reaction response time - Proportion correctly classified |
Tower of London [45] | Planning | - Total number of moves - Mean solution time |
Balloon Analogue Risk Task [46] | Decision-making | - Mean balloon pumps - Total balloon pops |
Iowa Gambling Task [47] | Decision-making | - NET of beneficial and detrimental solutions. |
Task . | Cognitive domain . | Outcome variables . |
---|---|---|
Psychomotor Vigilance Test (PVT) | Sustained attention | - Number of lapses, defined as reactions ≥500 ms - Median reaction times <500 ms - Reaction speed (1/mean reaction time) - Slowest 10% reaction times. |
Continuous Performance Test [37] | Sustained and selective attention | - Mean reaction time - Proportion correct |
Digit Symbol Substitution Task (DSST) [38] | Working memory | - Number of correctly classified symbols - Number of incorrectly classified symbols |
Operation span [39] | Working memory | - Operation Span score (sum of sets recalled) - Total math errors |
Trail Making Task [40–42] | Task switching | - Number of errors (i.e. mouse tracings through the incorrect number or letter in the sequence) |
Stroop Task [43, 44] | Cognitive flexibility and inhibition | - Mean reaction response time - Proportion correctly classified |
Tower of London [45] | Planning | - Total number of moves - Mean solution time |
Balloon Analogue Risk Task [46] | Decision-making | - Mean balloon pumps - Total balloon pops |
Iowa Gambling Task [47] | Decision-making | - NET of beneficial and detrimental solutions. |
Task . | Cognitive domain . | Outcome variables . |
---|---|---|
Psychomotor Vigilance Test (PVT) | Sustained attention | - Number of lapses, defined as reactions ≥500 ms - Median reaction times <500 ms - Reaction speed (1/mean reaction time) - Slowest 10% reaction times. |
Continuous Performance Test [37] | Sustained and selective attention | - Mean reaction time - Proportion correct |
Digit Symbol Substitution Task (DSST) [38] | Working memory | - Number of correctly classified symbols - Number of incorrectly classified symbols |
Operation span [39] | Working memory | - Operation Span score (sum of sets recalled) - Total math errors |
Trail Making Task [40–42] | Task switching | - Number of errors (i.e. mouse tracings through the incorrect number or letter in the sequence) |
Stroop Task [43, 44] | Cognitive flexibility and inhibition | - Mean reaction response time - Proportion correctly classified |
Tower of London [45] | Planning | - Total number of moves - Mean solution time |
Balloon Analogue Risk Task [46] | Decision-making | - Mean balloon pumps - Total balloon pops |
Iowa Gambling Task [47] | Decision-making | - NET of beneficial and detrimental solutions. |
Subjective sleepiness.
Over the course of each simulated night shift, participants were asked to self-rate their current level of sleepiness, using Qualtrics software, on the Karolinska Sleepiness Scale (KSS) from 1 = “extremely alert” to 9 = “very sleepy, great effort to keep awake, fighting sleep” [48].
Sleep.
In-laboratory polysomnography was setup and recorded according to American Academy of Sleep Medicine (AASM) guidelines [49] using Compumedics Grael devices and Profusion software (version 4) during the laboratory visits. This included 13 channels of electroencephalography (EEG), right and left electrooculography (EOG), chin electromyography (EMG), two-lead electrocardiography (ECG), oximetry, and position recordings during all sleep opportunities. A trained and qualified sleep technician masked to lighting conditions scored the data according to AASM sleep staging criteria [49]. Total sleep time, wake after sleep onset, sleep onset latency, sleep efficiency, and sleep stage durations (rapid eye movement [REM] sleep and non-REM sleep stages 1, 2, and 3) are reported.
Actiwatch Spectrum devices (Philips Respironics) recorded movement in three-dimensional space via a tri-axial accelerometer worn on the wrist of the non-dominant hand for 14 days prior to each laboratory visit. Movement data were pre-processed in 30-second epochs. The Philips Actiware Sleep software (version 6.0.0, Philips Respironics, Bend, OR) scored each epoch into sleep or wake by using a wake threshold of 40 (medium setting), 10 immobile minutes for sleep interval detection, and 5 consecutive sleep epochs for sleep onset and offset detection. The sleep interval onset and offset times were manually checked by study personnel against daily sleep diaries completed by participants. Total sleep time, wake after sleep onset, sleep onset latency, and sleep efficiency are reported.
Study protocol
An overview of the laboratory protocol is shown in Figure 1. Participants monitored their sleep using the Actiwatch Spectrum device for 14 days prior to each laboratory visit. Participants then arrived at the sleep laboratory by 18:00 on a Friday (day 1) for study setup, after returning a negative COVID-19 rapid antigen lateral flow test. Participants underwent polysomnography recording during all sleep opportunities, with their first opportunity from 22:00 to 07:00. During the morning on day 2, participants practiced the cognitive performance tasks to help mitigate learning effects. At 18:00, participants began core body temperature and dim light melatonin onset (DLMO) assessments as markers of circadian timing.
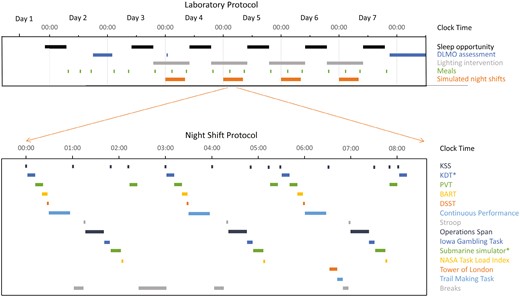
Laboratory protocol across 7 days (upper), and night shift protocol 00:00–08:00 (lower, inset), with timing of cognitive performance batteries. *denotes tasks not examined in the current study. BART, Balloon Analogue Risk Task; DLMO, Dim light melatonin onset; DSST, Digit Symbol Substitution Task; KDT, Karolinska Drowsiness Test; KSS, Karolinska Sleepiness Scale; PVT, Psychomotor Vigilance Task.
Participants then remained continuously awake for 27 hours to simulate an abrupt transition to night-shift work without a nap opportunity (as is common for submariners preparing to leave shore), from 07:00 on day 2 until the next sleep opportunity from 10:00 to 19:00 on day 3. Upon waking, participants showered, had a meal, were re-setup for polysomnography recording, and then undertook simulated night shift work from 00:00 to 08:00. This included a comprehensive cognitive test battery repeated three times across each 8-hour simulated work shift, with only brief breaks (5–15 minutes) and a single 30-minute meal break at 02:30 separating the ~7 hours of cognitive testing across the 8-hour shift (Figure 1). Participants then remained awake prior to the next sleep opportunity (10:00–19:00). This day-sleep/night shift work protocol was repeated on days 4–7. After the final sleep opportunity ended at 19:00 on day 7, participants completed a further DLMO assessment from 21:00 to 13:00 on day 8 which required participants to remain awake (monitored via polysomnography) in dim lighting conditions. Following the DLMO assessment (~13:30), participants left the laboratory.
Statistical analyses
For each cognitive performance outcome, the three-way interactions of lighting condition (circadian-informed lighting, control lighting), day (days 4–7), and time of task administration (clock time) were tested using either linear or negative binomial mixed models, according to the dependent variable distribution. The higher-order interaction was retained in the model if significant, otherwise two-way interactions were tested and retained as appropriate to achieve a parsimonious model. All models included visit number (first, second visit) and sequence (circadian-informed lighting first, or control lighting first) as fixed effects and day nested within participant ID as random effects, as this consistently improved model fits. Where relevant, estimated marginal means were examined using Bonferroni-corrected pairwise contrasts. No correction was made for comparisons across multiple performance tests. Model assumptions were examined for homoscedasticity, outliers, and normality, and appropriate distributions were used where needed in the models. p-values < .05 were considered statistically significant. All statistical analyses were conducted in R (version 4.3.2) [50] using the lmerTest [51] and glmmTMB packages [52].
Results
Participants
In total, 158 people responded to study advertisements and were assessed for inclusion. Of these, 28 met the study criteria, consented, and were randomized to allocation order. The final sample available for analysis consisted of 19 participants who completed the entire study protocol. The remaining participants withdrew due to illness (n = 4), personal matters (n = 3), scheduling conflicts (n = 1), and sleep difficulties that began after study consent, but prior to study interventions, which rendered them ineligible for participation (n = 1). Participant demographics and self-reported sleep at screening as well as actigraphy-derived sleep during the 14 days prior to each laboratory visit and polysomnography-derived sleep on Night 1 of the laboratory visit are presented in Table 2. There were no significant differences in any actigraphy-derived or polysomnography-derived sleep variable between the control and circadian-informed lighting conditions at baseline, all p > .12.
Participant and Self-reported Sleep Characteristics Collected During Screening, Actigraphy-Derived Sleep in the 14-day Baseline Prior to a Laboratory Visit, and Polysomnography Sleep on Night 1 of the Laboratory Visits
Characteristics . | Final sample (N = 19) . | |
---|---|---|
Age, mean (SD), y | 28.7 (10.4) | |
Sex, No. (%) | ||
Male | 12 (63) | |
Female | 7 (37) | |
Body mass index, mean (SD), kg/m2 | 24.8 (3.9) | |
Weekly alcohol consumption, No. (SD) | 0.3 (0.5) | |
Daily caffeine consumption, No. (SD) | 0.9 (0.9) | |
Self-reported habitual bedtime, mean (SD), min | 22:51 (01:09) | |
Self-reported habitual wake up time, mean (SD), min | 07:29 (00:56) | |
Self-reported habitual total sleep time, mean (SD) hrs | 7.7 (0.9) | |
Morningness-Eveningness Questionnaire, mean (SD) | 15.2 (2.4) | |
Insomnia Severity Index, mean (SD) | 4.0 (3.9) | |
Pittsburgh Sleep Quality Index, mean (SD) | 4.1 (2.7) | |
Epworth Sleepiness Scale, mean (SD) | 3.8 (3.2) |
Characteristics . | Final sample (N = 19) . | |
---|---|---|
Age, mean (SD), y | 28.7 (10.4) | |
Sex, No. (%) | ||
Male | 12 (63) | |
Female | 7 (37) | |
Body mass index, mean (SD), kg/m2 | 24.8 (3.9) | |
Weekly alcohol consumption, No. (SD) | 0.3 (0.5) | |
Daily caffeine consumption, No. (SD) | 0.9 (0.9) | |
Self-reported habitual bedtime, mean (SD), min | 22:51 (01:09) | |
Self-reported habitual wake up time, mean (SD), min | 07:29 (00:56) | |
Self-reported habitual total sleep time, mean (SD) hrs | 7.7 (0.9) | |
Morningness-Eveningness Questionnaire, mean (SD) | 15.2 (2.4) | |
Insomnia Severity Index, mean (SD) | 4.0 (3.9) | |
Pittsburgh Sleep Quality Index, mean (SD) | 4.1 (2.7) | |
Epworth Sleepiness Scale, mean (SD) | 3.8 (3.2) |
Actigraphy-derived Sleep during 14-day baseline . | Control lighting . | Circadian-informed lighting . |
---|---|---|
Total sleep time, mean (SD), hrs | 6.8 (1.1) | 6.7 (0.9) |
Sleep onset latency, mean (SD), min | 19.6 (13.0) | 20.6 (13.5) |
Wake after sleep onset, mean (SD), min | 39.1 (19.1) | 37.9 (16.5) |
Sleep efficiency, mean (SD), min | 84.4 (4.7) | 84.2 (4.4) |
Actigraphy-derived Sleep during 14-day baseline . | Control lighting . | Circadian-informed lighting . |
---|---|---|
Total sleep time, mean (SD), hrs | 6.8 (1.1) | 6.7 (0.9) |
Sleep onset latency, mean (SD), min | 19.6 (13.0) | 20.6 (13.5) |
Wake after sleep onset, mean (SD), min | 39.1 (19.1) | 37.9 (16.5) |
Sleep efficiency, mean (SD), min | 84.4 (4.7) | 84.2 (4.4) |
Polysomnography-derived sleep on night 1 of the laboratory visit . | Control lighting . | Circadian-informed lighting . |
---|---|---|
Total sleep time, mean (SD), hrs | 8.6 (0.4) | 8.6 (0.4) |
Sleep onset latency, mean (SD), min | 40.8 (28.6) | 31.6 (21.9) |
Wake after sleep onset, mean (SD), min | 58.9 (38.5) | 70.2 (60.0) |
Sleep efficiency, mean (SD), min | 82.3 (7.5) | 81.8 (11.0) |
N1 sleep duration, mean (SD), min | 31.5 (11.8) | 30.9 (12.6) |
N2 sleep duration, mean (SD), min | 232.0 (32.2) | 215.9 (39.2) |
N3 sleep duration, mean (SD), min | 113.5 (31.5) | 119.5 (34.4) |
REM sleep duration, mean (SD), min | 86.7 (32.8) | 93.1 (34.5) |
Polysomnography-derived sleep on night 1 of the laboratory visit . | Control lighting . | Circadian-informed lighting . |
---|---|---|
Total sleep time, mean (SD), hrs | 8.6 (0.4) | 8.6 (0.4) |
Sleep onset latency, mean (SD), min | 40.8 (28.6) | 31.6 (21.9) |
Wake after sleep onset, mean (SD), min | 58.9 (38.5) | 70.2 (60.0) |
Sleep efficiency, mean (SD), min | 82.3 (7.5) | 81.8 (11.0) |
N1 sleep duration, mean (SD), min | 31.5 (11.8) | 30.9 (12.6) |
N2 sleep duration, mean (SD), min | 232.0 (32.2) | 215.9 (39.2) |
N3 sleep duration, mean (SD), min | 113.5 (31.5) | 119.5 (34.4) |
REM sleep duration, mean (SD), min | 86.7 (32.8) | 93.1 (34.5) |
N1, non-REM sleep stage 1; N2, Non-REM sleep stage 2; N3, Non-REM sleep stage 3; REM, rapid eye movement; SD, standard deviation.
Participant and Self-reported Sleep Characteristics Collected During Screening, Actigraphy-Derived Sleep in the 14-day Baseline Prior to a Laboratory Visit, and Polysomnography Sleep on Night 1 of the Laboratory Visits
Characteristics . | Final sample (N = 19) . | |
---|---|---|
Age, mean (SD), y | 28.7 (10.4) | |
Sex, No. (%) | ||
Male | 12 (63) | |
Female | 7 (37) | |
Body mass index, mean (SD), kg/m2 | 24.8 (3.9) | |
Weekly alcohol consumption, No. (SD) | 0.3 (0.5) | |
Daily caffeine consumption, No. (SD) | 0.9 (0.9) | |
Self-reported habitual bedtime, mean (SD), min | 22:51 (01:09) | |
Self-reported habitual wake up time, mean (SD), min | 07:29 (00:56) | |
Self-reported habitual total sleep time, mean (SD) hrs | 7.7 (0.9) | |
Morningness-Eveningness Questionnaire, mean (SD) | 15.2 (2.4) | |
Insomnia Severity Index, mean (SD) | 4.0 (3.9) | |
Pittsburgh Sleep Quality Index, mean (SD) | 4.1 (2.7) | |
Epworth Sleepiness Scale, mean (SD) | 3.8 (3.2) |
Characteristics . | Final sample (N = 19) . | |
---|---|---|
Age, mean (SD), y | 28.7 (10.4) | |
Sex, No. (%) | ||
Male | 12 (63) | |
Female | 7 (37) | |
Body mass index, mean (SD), kg/m2 | 24.8 (3.9) | |
Weekly alcohol consumption, No. (SD) | 0.3 (0.5) | |
Daily caffeine consumption, No. (SD) | 0.9 (0.9) | |
Self-reported habitual bedtime, mean (SD), min | 22:51 (01:09) | |
Self-reported habitual wake up time, mean (SD), min | 07:29 (00:56) | |
Self-reported habitual total sleep time, mean (SD) hrs | 7.7 (0.9) | |
Morningness-Eveningness Questionnaire, mean (SD) | 15.2 (2.4) | |
Insomnia Severity Index, mean (SD) | 4.0 (3.9) | |
Pittsburgh Sleep Quality Index, mean (SD) | 4.1 (2.7) | |
Epworth Sleepiness Scale, mean (SD) | 3.8 (3.2) |
Actigraphy-derived Sleep during 14-day baseline . | Control lighting . | Circadian-informed lighting . |
---|---|---|
Total sleep time, mean (SD), hrs | 6.8 (1.1) | 6.7 (0.9) |
Sleep onset latency, mean (SD), min | 19.6 (13.0) | 20.6 (13.5) |
Wake after sleep onset, mean (SD), min | 39.1 (19.1) | 37.9 (16.5) |
Sleep efficiency, mean (SD), min | 84.4 (4.7) | 84.2 (4.4) |
Actigraphy-derived Sleep during 14-day baseline . | Control lighting . | Circadian-informed lighting . |
---|---|---|
Total sleep time, mean (SD), hrs | 6.8 (1.1) | 6.7 (0.9) |
Sleep onset latency, mean (SD), min | 19.6 (13.0) | 20.6 (13.5) |
Wake after sleep onset, mean (SD), min | 39.1 (19.1) | 37.9 (16.5) |
Sleep efficiency, mean (SD), min | 84.4 (4.7) | 84.2 (4.4) |
Polysomnography-derived sleep on night 1 of the laboratory visit . | Control lighting . | Circadian-informed lighting . |
---|---|---|
Total sleep time, mean (SD), hrs | 8.6 (0.4) | 8.6 (0.4) |
Sleep onset latency, mean (SD), min | 40.8 (28.6) | 31.6 (21.9) |
Wake after sleep onset, mean (SD), min | 58.9 (38.5) | 70.2 (60.0) |
Sleep efficiency, mean (SD), min | 82.3 (7.5) | 81.8 (11.0) |
N1 sleep duration, mean (SD), min | 31.5 (11.8) | 30.9 (12.6) |
N2 sleep duration, mean (SD), min | 232.0 (32.2) | 215.9 (39.2) |
N3 sleep duration, mean (SD), min | 113.5 (31.5) | 119.5 (34.4) |
REM sleep duration, mean (SD), min | 86.7 (32.8) | 93.1 (34.5) |
Polysomnography-derived sleep on night 1 of the laboratory visit . | Control lighting . | Circadian-informed lighting . |
---|---|---|
Total sleep time, mean (SD), hrs | 8.6 (0.4) | 8.6 (0.4) |
Sleep onset latency, mean (SD), min | 40.8 (28.6) | 31.6 (21.9) |
Wake after sleep onset, mean (SD), min | 58.9 (38.5) | 70.2 (60.0) |
Sleep efficiency, mean (SD), min | 82.3 (7.5) | 81.8 (11.0) |
N1 sleep duration, mean (SD), min | 31.5 (11.8) | 30.9 (12.6) |
N2 sleep duration, mean (SD), min | 232.0 (32.2) | 215.9 (39.2) |
N3 sleep duration, mean (SD), min | 113.5 (31.5) | 119.5 (34.4) |
REM sleep duration, mean (SD), min | 86.7 (32.8) | 93.1 (34.5) |
N1, non-REM sleep stage 1; N2, Non-REM sleep stage 2; N3, Non-REM sleep stage 3; REM, rapid eye movement; SD, standard deviation.
Cognitive performance
PVT performance.
The performance outcomes from the PVT for each condition and day are shown in Figure 2. There was a significant condition-by-day-by-time task interaction effect on PVT lapses (X2(3) = 19.78, p < .001). Participants had significantly fewer lapses in the circadian-informed lighting condition during the latter task administrations on days 6 and 7 than in the control lighting. There were also significant condition-by-day-by-time of task interaction effects on median reaction time (F(3,816) = 2.97, p = .03, partial η2 = 0.44), reaction speed (F(3,816) = 3.49, p = .02, partial η2 = 0.49), and slowest 10% reaction times (F(3,817) = 6.03, p = .01, partial η2 = 0.39). Compared to the control lighting condition, participants had faster reaction speeds during the latter task administrations on days 6 and 7. Despite the significant condition-by-day-by-time of task interaction, post hoc tests did not find significant differences in median reaction time. There were significant marginal effects of lighting condition on PVT lapses (X2(1) = 3.47, p = .04), median reaction time (F(1,816) = 12.10, p = .001, partial η2 = 0.27), reaction speed (F(1,784) = 70.31, p < .001, partial η2 = 0.08), and slowest 10% reaction times (F(1,780) = 91.44, p < .001, partial η2 = 0.10), such that performance was better in the circadian-informed lighting condition compared to the control lighting condition. Across PVT performance models, there were significant visit number effects (p < .001), with poorer performance found on the second laboratory visit compared to the first visit (Supplementary Figures S1 and S2). Sequence was not significant in any model (p > .35). Further analyses found that greater circadian phase delay on core body temperature minimum timing was associated with greater improvement in PVT lapses across simulated night shifts in the circadian-informed lighting condition, but no other associations were found between circadian phase shift and PVT performance (Supplementary File).
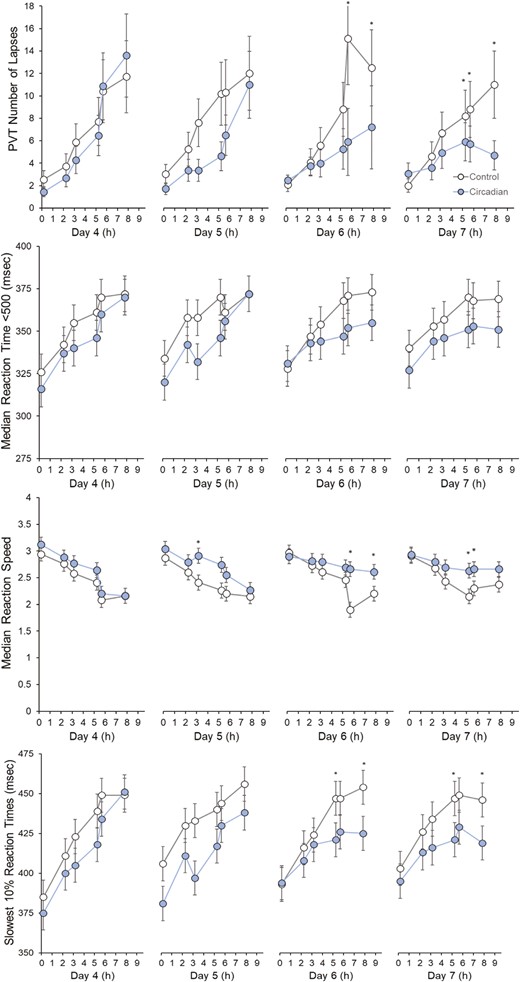
Psychomotor vigilance task (PVT) performance on lapses, median reaction time, reaction speed, and slowest 10% reaction times across condition, day, and time of a task. Asterisks denote significant differences between circadian-informed lighting versus control light. Values are EMM ± SEM.
Other performance task outcomes.
The performance outcomes from all other performance tasks for each condition and day are shown in Supplementary Figures S3–S10). There was a significant condition-by-day interaction effect on the number of errors combined on each trail of the Trail Making Task (X2(3) = 12.37, p = .006). Participants made significantly fewer errors in the circadian-informed lighting condition (M = 1.26, [95% CIs: 0.77, 2.08]) than in the controlled lighting (M = 2.30, [1.49, 3.55]) on day 5 (p < .001), but there were no significant differences on days 4, 6, or 7 (p > .22). The marginal effect of condition was also significant (X2(3) = 5.28, p = .02) such that performance was better in the circadian-informed lighting condition compared to the control lighting condition. Visit number and sequence effects were not significant (p > .13).
There was a significant condition effect on the proportion of incorrect classifications on the Stroop task (X2(1) = 4.99, p = .03) such that participants performed marginally better in the control condition (M = 2.65, [2.22, 3.08]) than the circadian-informed lighting condition (M = 3.05, [2.56, 3.54]). No other effects were significant (p > .08). There was also a significant day-by-time task interaction effect on the mean response time on the Stroop task (F(3,365) = 10.94, p < .001) such that the declining rate of response times over the course of the night increased with each day. Condition effect was not significant (p = .24). There were also significant visit number effects on mean response time (p < .001) but not incorrect classifications (p = .18), with poorer performance observed on the second laboratory visit compared to the first laboratory visit. The sequence was not significant on either outcome, p > .19.
On all other cognitive performance tasks, the condition did not have a significant main effect or included in any significant interactions. There was a significant day effect on the Operation Span total score (F(3,419) = 4.07, p < .001), such that total scores increased with each day. There was significant day (X2(3) = 11.31, p = .01) and time of task effects (X2(1) = 4.56, p = .03) on the number of Operation Span errors, with participants making fewer errors with each day and towards the end of the night shift. There were also significant visit number effects on total scores (p < .001) and number of errors (p < .001), with poorer performance observed on the second laboratory visit compared to the first laboratory visit. No other effects were significant on either outcome (p > .24).
Significant day effects were found on the mean solution time of the Tower of London (F(3,124) = 6.32, p < .001), such that participants solved the task faster with each day. A significant visit number effect was also found (F(1,124) = 10.06, p = .002), with participants solving the task faster during the second visit than the first visit. No other effects were significant (p > .20). There were also no significant effects on the number of moves to solve the Tower of London (p > .20).
Significant time of task effects were found on the mean reaction time (F(1,362) = 27.06, p < .001) and number of errors (X2(1) = 17.85, p < .001) on the Continuous Performance Test. Participants took longer to respond and made more errors towards the end of the night shift. No other effects were significant for either outcome (p > .06).
There were no significant main effects or interactions on the number of correctly or incorrectly classified symbols on the DSST (p > .12), the mean pumps of the balloon on total number of balloon explosions on the BART (p > .21), or the NET scores on the Iowa Gambling Task (p > .38).
Subjective sleepiness.
Figure 3 shows the KSS ratings across each night shift in both conditions. There was a significant condition-by-day-by-time task interaction effect on KSS, F(3,1161) = 5.40, p = .001. Participants reported lower KSS ratings near the midpoints of the simulated night shifts on days 6 and 7. Visit number and sequence effects were not significant (p > .58).
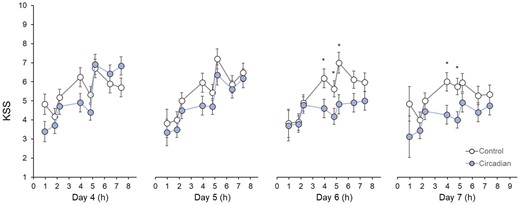
Karolinska Sleepiness Scale (KSS) ratings across condition, day, and time of task. Asterisks denote significant differences between circadian-informed lighting versus control light. Values are EMM ± SEM.
Polysomnography-derived Sleep
Figure 4 shows the polysomnography-derived sleep parameters for each sleep opportunity in both conditions. There were significant condition-by-day interaction effects on total sleep time (F(5,103) = 2.85, p = .02), sleep onset latency (F(5,191) = 2.57, p = .03), wake after sleep onset (F(5,102) = 2.68, p = .03), and sleep efficiency (F(5,104) = 3.13, p = .01). By day 7, participants slept 52.0 minutes longer [95% CI: 27.5, 76.5 minutes], spent 64.0 minutes [38.5, 89.5] less time awake during the sleep period, and showed higher sleep efficiency (difference 9.0% [4.5, 13.4]) under circadian-informed lighting compared to control lighting.
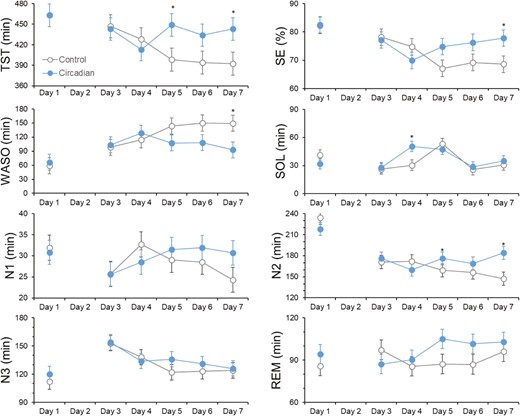
Polysomnography-derived sleep continuity and sleep stages across condition and day. Asterisks denote significant differences between circadian-informed lighting versus control light. Values are EMM ± SEM. N1, Non-REM Stage 1; N2, Non-REM Stage 2; N3, Non-REM Stage 3; REM, rapid eye movement sleep; SE, Sleep efficiency; SOL, Sleep onset latency; TST, total sleep time; WASO, Wake after sleep onset.
A significant condition-by-day interaction effect was found for the duration of N2 sleep (F(5,103) = 2.85, p = .02), with 52.0 [38.5, 65.5] minutes more N2 sleep by day 7 under circadian-informed lighting than the controlled lighting. No effects were significant for other sleep stages (p > .12).
Discussion
The main findings of this study are that circadian-informed lighting significantly reduces attention-related failures and improves PVT reaction times and reaction speed compared to control lighting. These were large effects, with a two-fold difference in PVT lapses between lighting conditions during the fourth and final simulated night shift. Other cognitive performance assessments, including working memory, classification accuracy, task switching, and cognitive flexibility did not show any consistent evidence of lighting intervention effects. However, sleep was also markedly improved, with almost 1 hour of additional N2 sleep, that replaced wakefulness during the final sleep opportunity with circadian-informed versus control lighting. Self-reported sleepiness during the work shift also progressively improved across night shifts with circadian compared to control lighting, specifically during the midpoints of the simulated night shifts on days 6 and 7. These findings are consistent with lighting intervention effects on the circadian-related timing of the core body temperature minimum time in these participants [30] and well-known prominent circadian effects on vigilance and sleepiness, which are typically poorest around the core body temperature nadir [53, 54].
As reported previously, the circadian timing shifts achieved through this minimally intrusive and largely automated lighting schedule were quite remarkable [30]. Both DLMO and core body temperature-based assessments of circadian timing shifted approximately 4 hours more, by around 1.5 hours versus 0.5 hours per day, over the course of 4 work-shift lighting exposure periods than the control lighting. Thus, by the final day of the study, participants had achieved a phase delay of around 6.5 hours versus 2 hours with circadian compared to control lighting. Longer studies and exposure periods are required to examine a full circadian adjustment and maintenance of the adjustment to a 12-hour work-rest schedule change. However, if daily changes continue, circadian lighting would be expected to achieve full circadian re-adjustment within approximately 7 days compared to around 3 weeks with conventional lighting, assuming dim lighting is sufficient to achieve circadian entrainment to the wake–sleep schedule change at all. This markedly accelerated circadian shift would be highly beneficial for improving performance and sleep in shift workers who frequently have difficulty adapting to prolonged or permanent night shift work schedules.
These findings add to a growing body of evidence for the efficacy of lighting-based interventions as a circadian realignment and performance enhancement strategy in shift-work contexts. Prior studies have found benefits from light interventions to improve sleep and/or performance, including overhead lighting and individually worn light administration devices [55–59], and in workers on night shift work schedules [60–64]. This study is amongst the first tightly controlled in-laboratory trials to have simultaneously evaluated circadian-lighting effects on circadian timing, work-shift cognitive performance, and sleep following an abrupt transition to night-shift work. Further, to help contextualize these findings, by the end of the final day, participants showed attention-related failures at a level equivalent to that of 3 days of sleep deprivation in the control lighting condition versus 1 day of deprivation in the circadian-informed lighting condition [12]. Interestingly, performance deficits did not appear to accumulate across successive days of shorter-than-normal sleep in either lighting condition, as found previously [12, 22]. It may be the case that the transition to the night shift (27 hours extended wake) resulted in significant performance decrements during the first-night shift work, and the additional sleep restriction thereafter did not produce a discernible cumulative effect.
In line with prior research, the cognitive performance benefits of this circadian-informed lighting intervention were only clearly prominent in vigilance, with other cognitive domains not as responsive to the circadian-informed lighting intervention. To some extent, individuals also reported higher levels of sleepiness with control versus circadian lighting, following a similar pattern to the PVT findings. Other studies on the acute alerting effects of light have also found that the most prominent, reliable effects are observed on PVT performance and subjective alertness measures, especially at nighttime [65–67]. Taken together, circadian lighting interventions may not produce robust, discernible improvements in some cognitive functions. Alternatively, practice effects may mask any potential differences. While alternate task versions were used for repeat cognitive task administrations in the current study, it is a possibility that participants may quickly learn and employ the same strategy consistently during each repeat administration, resulting in similar levels of performance over time. Nonetheless, the findings of the current study also support that vigilance is particularly sensitive, and higher executive functions are more robust, to the acute and circadian effects of light.
The relative contribution of circadian versus sleep deficits remains unclear, although both are likely to help explain these findings. Furthermore, given incomplete circadian adjustment after 96 hours, it is likely that the benefits of circadian-informed lighting would continue until circadian re-entrainment to the new shift schedule was complete, at which point daily changes in circadian light exposure timing would no longer be appropriate. Benefits to other cognitive domains may well also occur as circadian timing and sleep deficits continue to diminish, especially given that sleep benefits did not clearly emerge until the final sleep opportunity. Studies with longer lighting intervention protocols are warranted to test this hypothesis. Nonetheless, consistent with prior studies with submariners [55, 57], the current findings support that fully automated overhead lighting can enhance work performance, particularly for duties that rely on sustained attention in work environments without normal solar-like lighting cues. Accordingly, circadian-based lighting strategies clearly warrant consideration as an effective method to improve shift worker performance among other human capability enhancement strategies, including strategically timed vigilance-optimized shift rosters, rest intervals, nap opportunities, and wake-promoting interventions such as caffeine.
Given the influence of sleep and circadian rhythms on performance, and their major dysregulation with physiologically challenging work-shift schedules [4], regulating sleep and circadian rhythms remains a key high-value target to maximize shift worker performance. Successfully doing so in real-world environments will have several logistical and operational challenges, particularly in high-risk contexts. Strenuous work-rest-sleep schedules, imperative for an always-ready workforce, stress associated with work duties, and challenging sleeping conditions (comfort, temperature, noise, light, etc.) will clearly inhibit sleep. Rotating work shift schedules, long shifts, and environmental conditions also disrupt circadian rhythms, which will further impair performance. Nevertheless, even within these unavoidable constraints, a range of sleep promotion and circadian regulation strategies could feasibly be implemented to enhance shift worker performance and longer-term health, well-being, and work-force retention. Automated overhead lighting to provide appropriately timed blue-enriched versus blue-depleted light stimuli to facilitate alerting effects and favorable circadian adjustments to suit work-rest schedules is perhaps one of the most feasible and high-value strategies for implementation.
Despite the strengths of this tightly controlled, within-participants cross-over study design, there are several limitations to consider. While best efforts were made to retain participants through both lighting conditions, 7/26 participants withdrew after consent. Thus, the absence of lighting effects on several cognitive performance markers could potentially reflect type II errors. On the other hand, large effects on lapses and median reaction time, circadian timing markers, and sleep outcomes, clearly indicate large and consistent effects on key relevant outcomes. While a key goal was to comprehensively evaluate multiple cognitive functions under challenging simulated shift-work conditions, the high cognitive load from ~7 hours of demanding cognitive tasks administered at a physiologically challenging time of day may have reduced participant motivation to complete the tasks to their best ability, particularly on the second visit. Consistently lower PVT performance on the second compared to the first visit supports the presence of important motivational influences, which could have masked more subtle circadian influences on cognitive performance outcomes and/or any cumulative deficits in performance across successive days. To some extent, motivation is an important aspect of real-world work performance. Nonetheless, motivation in real-world settings is likely to be consistently higher than in laboratory simulation studies, so further testing is warranted in more real-world work settings.
A further notable limitation is that the circadian-informed lighting intervention relied on model-based estimates of “average” circadian system responses to light exposure at a group level. Thus, interindividual variability in circadian timing and sensitivity to light exposure, and other factors that influence the rate of circadian adjustment following a 12-hour sleep and work-shift schedule change, should be expected to strongly influence individual responses. Better-individualized lighting interventions are likely to be more effective at accelerating circadian re-adjustment. On the other hand, the group-level lighting in this study was clearly effective and may well be more practical to implement in some workplace contexts, as accomplished in prior studies of lighting interventions in workplace settings [61, 62, 68]. Further research is required to determine the practicality and potential benefits of sleep and performance with group-level versus individualized circadian lighting strategies.
In addition to experimental studies to evaluate the potential benefits of a personalized circadian-informed lighting strategy, studies to examine the potential benefits of this lighting strategy across markers of physical performance, health, and well-being would be insightful. Further research is also warranted to adapt and test this circadian-informed lighting strategy under different submariner watch schedules (e.g. 6 hours on/6 hours off), to facilitate the deployment of circadian-informed lighting in shift work environments where lighting interventions may be practical to implement, such as hospitals, factories, and submarines. Furthermore, studies in real-world workplaces are warranted to test whether circadian-informed lighting administered for a portion of the day while at work (with potentially limited control over light exposure outside work hours) could yield benefits to real-world work performance and health. Given the importance of sleep and circadian systems for optimal human function, circadian-based lighting may serve as a practical and deployable strategy to improve work performance and longer-term health and well-being.
Supplementary material
Supplementary material is available at SLEEP online.
Acknowledgments
This research is supported by the Maritime Division of the Defence Science and Technology Group through the Research Network for Undersea Decision Superiority. This research was also financially supported by the office of the Deputy Vice Chancellor of Research (Flinders University), the College of Medicine and Public Health (Flinders University), and REDARC. DJE is funded by a National Health and Medical Research Council of Australia Leadership Fellowship (1196261). We gratefully acknowledge the support from DSTG personnel and our colleagues at Flinders University, University of South Australia, and University of Adelaide.
Disclosures
Financial disclosures: HS reports consultancy and/or research support unrelated to this work from Re-Time Pty Ltd, Compumedics Ltd, the American Academy of Sleep Medicine Foundation, and Flinders University. In addition to direct support for this project from the Australian Government Department of Defence, Flinders University and REDARC, PC reports grant and equipment support unrelated to this work from the National Health and Medical Research Council of Australia, Flinders Foundation, Garnett Passe and Rodney Williams Foundation, Invicta Medical, and a Flinders University collaboration grant with Compumedics Ltd. DJE reports grants from Bayer, Apnimed, Invicta Medical, Takeda, Eli Lilly, Withings, SomnoMed, Openairway, and has served as a consultant for Bayer and Takeda and on the Scientific Advisory Boards for Invicta Medical, Mosanna and Apnimed outside the submitted work. Nonfinancial disclosures: None to disclose.
Data Availability
The data underlying this article will be shared on reasonable request to the corresponding author.
Comments