-
PDF
- Split View
-
Views
-
Cite
Cite
Wan-Ni Wang, Yu-Ting Wei, Sheng-Ting Zhao, Fu-Huan Yu, Jing-wen Wang, Cheng-yue Gu, Xin-Ran Liu, Na Sai, Jin-Lei Zhu, Qi-Meng Wang, Qin-Xin Bao, Xin-Rong Mu, Yu-xin Liu, Gary J Loake, Ji-hong Jiang, Lai-Sheng Meng, ABSCISIC ACID-INSENSITIVE 5-KIP-RELATED PROTEIN 1-SHOOT MERISTEMLESS modulates reproductive development of Arabidopsis, Plant Physiology, Volume 195, Issue 3, July 2024, Pages 2309–2322, https://doi.org/10.1093/plphys/kiae146
- Share Icon Share
Abstract
Soil (or plant) water deficit accelerates plant reproduction. However, the underpinning molecular mechanisms remain unknown. By modulating cell division/number, ABSCISIC ACID-INSENSITIVE 5 (ABI5), a key bZIP (basic (region) leucine zippers) transcription factor, regulates both seed development and abiotic stress responses. The KIP-RELATED PROTEIN (KRP) cyclin-dependent kinases (CDKs) play an essential role in controlling cell division, and SHOOT MERISTEMLESS (STM) plays a key role in the specification of flower meristem identity. Here, our findings show that abscisic acid (ABA) signaling and/or metabolism in adjust reproductive outputs (such as rosette leaf number and open flower number) under water-deficient conditions in Arabidopsis (Arabidopsis thaliana) plants. Reproductive outputs increased under water-sufficient conditions but decreased under water-deficient conditions in the ABA signaling/metabolism mutants abscisic acid2-1 (aba2-1), aba2-11, abscisic acid insensitive3-1 (abi3-1), abi4-1, abi5-7, and abi5-8. Further, under water-deficient conditions, ABA induced-ABI5 directly bound to the promoter of KRP1, which encodes a CDK that plays an essential role in controlling cell division, and this binding subsequently activated KRP1 expression. In turn, KRP1 physically interacted with STM, which functions in the specification of flower meristem identity, promoting STM degradation. We further demonstrate that reproductive outputs are adjusted by the ABI5–KRP1–STM molecular module under water-deficient conditions. Together, our findings reveal the molecular mechanism by which ABA signaling and/or metabolism regulate reproductive development under water-deficient conditions. These findings provide insights that may help guide crop yield improvement under water deficiency.
Introduction
Drought stress has a major impact on crop production outweighing crop yield losses from all other natural hazards (Lv et al. 2019). Under drought stress, a delay of reproductive timing can sometimes be observed (Zhang et al. 2016). However, the most prevalent response to terminal drought stress is to accelerate reproductive outputs (Su et al. 2013; Brodribb et al. 2016; Du et al. 2018; Meng 2018).
Arabidopsis reproductive outputs are determined by multiple factors including rosette leaf number, the number of flowers produced, flower and ovary development, open flower number, fruit maturation, ovary abortion, seed size reduction (seed filling rate), and fruit drop (Su et al. 2013). All these factors determine a final total seed weight after completion of the Arabidopsis life cycle. In this study, for simplification, we only focused on the two most relevant factors, rosette leaf number and open flower number, to determine a final total seed weight (plant reproductive outputs).
The molecular mechanisms triggering the modulation of reproductive outputs are complex. Firstly, the term, drought stress, is loosely used and may denote many different types of stresses including those resulting from shadow, light intensity, temperature, and wind. Moreover, abundant literature shows that both soil water deficit and plant water status are typically measured to score for “water deficiency” (Su et al. 2013). Therefore, understanding these physiological responses may help identify approaches that may contribute to improving crop yield under specific drought regimes (Su et al. 2013; Du et al. 2018; Meng 2018; Yu et al. 2020a). Moreover, abscisic acid (ABA) is an important plant hormone, involved in a wide variety of regulatory processes, including growth, development, and responses to stress. Changes in resource and energy allocation toward processes that increase survival are integrated into these stress responses (Robinson and Hill 1999; Yamaguchi-Shinozaki and Shinozaki 2005; Su et al. 2013; Du et al. 2018). Further, the evolutionary role of ABA has evolved to help improve plant fitness under stress (including water deficiency), where it integrates many different processes. Adjustments to plant reproductive outputs are complex under unpredictable environmental changes, including water deficiency. Therefore, the functions of ABA signaling in the context of water deficiency and associated responses are intricate and multifarious.
Arabidopsis lines defective in the function of the basic leucine zipper transcription factor ABSCISIC ACID-INSENSITIVE 5 (ABI5), involved in ABA signal transduction, presented large seed size (Wang et al. 2013; Cheng et al. 2014) and is involved in seed development and the regulation of maturity and subsequent plant cell death (Sakuraba et al. 2014; Su et al. 2016; Zinsmeister et al. 2016). The KIP-RELATED PROTEINs (KRPs) cyclin-dependent kinases (CDKs), cyclin-dependent kinase inhibitor proteins, also play a key regulatory role in cell size. These proteins have essential functions in the regulation of cell division and cell cycle progression (Wang et al. 1998, 2000). Therefore, KRPs/ICKs function as a negative regulators of cell proliferation, for example, regulation of seed size (Cheng et al. 2013) and mitotic cell cycle progression during Arabidopsis gametogenesis (Liu et al. 2008) and both ABI5 and KRPs/ICKs are closely connected to adjustments of reproductive outputs. Also, the functions of both ABI5 and KRPs/ICKs are associated with ABA signaling (Wang et al. 1998; Cheng et al. 2014; Su et al. 2016). However, whether either ABI5 or KRP1 are involved in ABA signaling and/or metabolism mediated-adjustment of reproductive outputs under water-deficient conditions remains to be determined.
In this study, we adopted a method for adjusting plant survival and plant reproductive outputs under constant water-deficient conditions (25% to 35% soil water content (SWC), which was maintained by using frequent irrigation) to explore the molecular mechanism of associated reproductive outputs (Su et al. 2013). Genotypic variation in “drought stress” requires careful interpretation because frequent irrigation to “constant” low SWC attenuates ABA accumulation (Puértolas et al. 2017). Our results indicate that under constant water-deficient conditions, leaf relative water content (RWC) was closely related to relative SWC, leaf ABA status, transpiration rate, daily water loss, and stomatal conductance. This suggests that plant water relations are coordinated with leaf ABA status. Therefore, constant water-deficient conditions may be suitable for studying adjustment of reproductive outputs in plants. We, therefore, employed this approach to test the hypothesis that ABA metabolism and associated signaling can adjust Arabidopsis reproductive outputs under different water stress environments (soil water deficit and plant water status). Our results indicated that under constant water-deficient conditions, ABA signals to decrease plant reproductive outputs, namely, rosette leaf number, open flower number, and final total seed weight. Further analysis revealed that under constant water-deficient conditions, ABI5 activated KRP1 transcription by direct binding to the KRP1 promoter. In turn, KRP1 interacted with SHOOT MERISTEMLESS (STM) to promote STM degradation and thereby inhibited inflorescence meristem activities. Our findings, therefore, reveal a molecular mechanism by which plant reproductive outputs are adjusted under constant water-deficient conditions. Moreover, this study identified several cognate components of this regulatory machinery that may have utility to help guide the breeding drought-tolerant crops.
Results
ABA metabolism and associated signaling mediate plant reproductive outputs under water-deficient conditions
Plant reproductive outputs are determined by multiple factors, which determine a final total seed weight after completion of the Arabidopsis life cycle. In this study, for simplification, we only focused on two key factors, rosette leaf number and open flower number, to determine a final total seed weight.
We employed the loss-of-function mutants abi3-1, abi4-1, abi5-7, abi5-8, aba2-1, aba2-11, and an ABI5-overexpression (ABI5ox) lines grown under long-day conditions (16-h light/8-h dark). Wild-type, abi5-7, abi5-8, ABI5ox, abi4-1, aba2-1 and aba2-11 plants all survived at high (∼91% to ∼78% considered as water-sufficient conditions) or low (34% to ∼29% considered as water-deficient conditions) relative SWC (Supplementary Fig. S1, A and B). Under water-sufficient conditions, leaf RWC (∼83% to ∼74%) was not significantly different within all genotypes (Supplementary Fig. S1C), whereas under water-deficient conditions, this parameter was increased in the ABI5ox plants (∼65%) and decreased in abi5-7, abi5-8, abi4-1, aba2-1, and aba2-11 (∼29% to ∼24%) mutants relative to wild-type (∼45%) (Supplementary Fig. S1C). This might be caused by different transpiration rates. Indeed, the transpiration rates were consistently decreased in the ABI5ox plants and increased in the abi5-7, abi5-8, abi4-1, aba2-1, and aba2-11 lines relative to wild-type, at the three time points assayed during the day (Supplementary Fig. S1D). This resulted in decreased daily water loss from ABI5ox plants and in contrast, increased daily water loss from abi5-7, abi5-8, abi4-1, aba2-1, and aba2-11 mutants relative to wild-type (Supplementary Fig. S1E). These findings may result from changes in stomatal conductance (Supplementary Fig. S1F) but not leaf area (Supplementary Fig. S1G).
Rosette leaf number is slightly reduced with increasing age (Robinson and Hill 1999). Under water-sufficient conditions, rosette leaf number was decreased in abi5-7, abi5-8, and abi4-1 plants and increased in ABI5ox, aba2-1, and aba2-11 lines relative to wild-type (Fig. 1A, Supplementary Fig. S2A). However, under water-deficient conditions, rosette leaf number was decreased in abi5-7, abi5-8, aba2-1, aba2-11, and abi4-1 plants and increased in the ABI5ox line relative to wild-type (Fig. 1B, Supplementary Fig. S2B). Further, the expression of the flowering repressor FLOWERING LOCUS C (FLC) (Xiong et al. 2019) was consistent with rosette leaf number (Fig. 1, A to D). Moreover, under water-sufficient conditions, aba2-1, aba2-11, abi4-1, abi5-7, and abi5-8 plants presented an increased open flower number, whereas ABI5ox plants exhibited a decreased open flower number (Fig. 1E, Supplementary Fig. S2C). By contrast, under water-deficient conditions, our findings were the complete opposite (Fig. 1F, Supplementary Fig. S2D). As a result, under water-sufficient conditions, a final total seed weight after completion of the Arabidopsis life cycle was increased in abi5-7, abi5-8, aba2-1, aba2-11, and abi4-1 plants but decreased in the ABI5ox line relative to wild-type (Fig. 1G, Supplementary Fig. S2E). By contrast, under water-deficient conditions, total seed weight was decreased in abi5-7, abi5-8, aba2-1, aba2-11, and abi4-1 plants and increased in the ABI5ox lines relative to wild-type (Fig. 1H, Supplementary Fig. S2F).
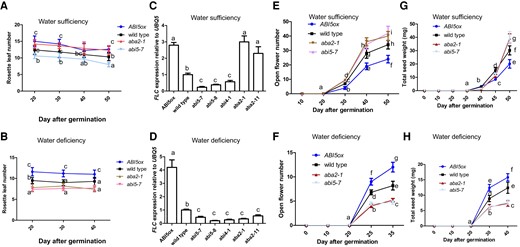
Reproductive outputs of ABA signaling mutant plants were adjusted under water-deficient conditions. A, B) Bar graph illustrating rosette leaf number of wild-type (Col-0), aba2-1, abi5-7, and 35S:ABI5-MYC (ABI5ox) plants by using a time-course analysis. Seedlings were grown in soil under water-sufficient (A) or -deficient (B) conditions. C, D) Bar graph illustrating FLC expression in all genotypes (wild-type (Col-0), aba2-1, aba2-11, abi4-1, abi5-7, abi5-8, and 35S:ABI5-MYC (ABI5ox)) for 40 DAG were grown in soil under water-sufficient (C) or -deficient (D) conditions. The expressions of FLC gene in wild-type plants were set as 1.0. Quantification was normalized to the expression of UBQ5.E, F) Bar graph illustrating open flower number of wild-type (Col-0), aba2-1, abi5-7, and ABI5ox plants by using a time-course analysis. Seedlings were grown in soil under water-sufficient (E) or -deficient (F) conditions. G, H) Bar graph illustrating total seed weight of wild-type, aba2-1, abi5-7, and ABI5ox plants by using a time-course analysis. Seedlings were grown in soil under water-sufficient (G) or -deficient (H) conditions. Seeds were harvested from wild-type and different genotypes determined. Please note: total seed weight indicates a final total seed weight after completion of plant life cycle. The ANOVA of two-way was utilized to calculate significant differences (n = 3). Tukey's post-test was used, and different lowercase letters above the bars indicate significant differences at *P < 0.05. Three replicate samples of 18 seedlings were harvested for analysis. The statistical analyses described apply to all statistical analyses in this figure. Error bars represent Sd (n = 3). Student's t-test (*P < 0.05).
abi3-1 plants partitioned more resources for seed development than wild-type (Ler) plants (Robinson and Hill 1999). Time-course analysis revealed that the dry weight and number of fruits per plant, total leaf area per plant and the number of rosette and caulinar leaves per plant in abi3-1 mutants were all higher than those in the wild-type (Ler) plants under water-sufficient conditions (Robinson and Hill 1999), indicating that abi3-1 plants may have more reproductive output than wild type. Indeed, the abi3-1 plants had more reproductive outputs under water-sufficient conditions but less reproductive outputs under water-deficient conditions relative to wild type (Supplementary Fig. S3). Therefore, ABI3 adjusts Arabidopsis reproductive outputs under water-deficient conditions.
We speculate that abi mutations in turn may elevate ABA levels under water-sufficient conditions. In this context, ABA could influence some developmental pathways independently of ABI4 or ABI5, thus explaining why we observed a phenotypic response mimicking that established by high ABA levels in these mutants.
In summary, our findings indicate that ABA metabolism and associated signaling mediates plant reproductive outputs under water-deficient conditions.
ABI5 adjusts reproductive outputs by controlling inflorescence meristem activity under water-deficiency
In this study, for simplification, we only focused on ABI5, not ABI3 and ABI4. This is because ABI5 is involved in the regulation of seed development and maturity and subsequent plant death by modulating cell division/number and thus is a key regulator of reproductive output (Cheng et al. 2014; Zinsmeister et al. 2016). Further, flower/fruit number is determined by shoot apical meristem (SAM) activities (Balanzà et al. 2018), suggesting that reproductive outputs might be affected by inflorescence meristem activities. Therefore, we determined whether the ABI5 genotype adjusts reproductive outputs by regulating inflorescence meristem activities under water-deficient conditions.
WUSCHEL (WUS), a homeodomain transcription factor, can be clearly detected in the SAM, where this factor is essential in maintaining the stem cell pool (Laux et al. 1996; Mayer et al. 1998). The STM gene encodes a class I knotted-like homeodomain protein required for SAM formation during embryogenesis and has a key function in the specification of flower meristem identity (Kirch et al. 2003). Therefore, WUS and STM can be considered as marker genes to detect inflorescence meristem activities under water-deficient conditions.
We thus determined whether ABI5 adjusts reproductive outputs by regulating inflorescence meristem activities under a given water status. Indeed, WUS expression in inflorescence meristems in the abi5-7 plants at 30-, 35-, and 40-days after germination (DAG) was elevated under water-sufficient conditions and decreased under water-deficient conditions relative to wild type (Fig. 2, A and B). Consistent with these data, reverse transcription quantitative PCR (RT-qPCR) analysis of wild-type and abi5-7 inflorescence meristems at 30, 40, and 50 DAG (water-sufficient conditions) or at 25, 30, and 35 DAG (water-deficient conditions) produced similar results (Fig. 2, C to F). Therefore, under water-sufficient conditions, the enhancement of abi5-7 inflorescence meristem activities may elevate open flower number, and thus raise a final total seed weight relative to wild type, whereas under water-deficient conditions, the opposite is the case (Fig. 1, E and F).
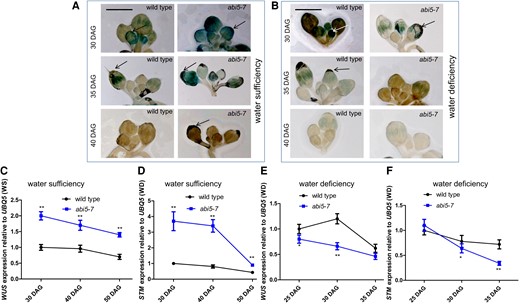
The activities of abi5-7 inflorescence meristems were raised or reduced under water-sufficient or -deficient conditions, respectively. A, B) Images illustrating expressions of WUSpro-GUS in SAMs of inflorescences from wild-type and abi5-7 plants at 30, 35, and 40 DAG grown in soil under water-sufficient (A) or -deficient (B) conditions. Arrows indicate SAM. Bar = 200 µm. C to F) Bar graph illustrating time-course RT-qPCR for the differential expression of WUS and STM genes between wild-type and abi5-7 plants at 30, 40, and 50 DAG grown in soil under water-sufficiency (C, D) or 25, 30, and 35 DAG grown in soil under water-deficiency (E, F) conditions. Inflorescence meristems were collected as materials for time-course RT-qPCR. The expressions of the WUS and STM genes in wild-type plants were set as 1.0. Quantification was normalized to the expression of UBQ5. Error bars represent Sd (n = 3). Student's t-test (*P < 0.05; **P < 0.01). WS, water sufficiency; WD, water deficiency.
Overall, our findings indicate that ABI5 adjusts reproductive outputs by regulating inflorescence meristem activities under water-deficient conditions.
Transcription factor ABI5 activates KRP1 expression
We firstly analyzed the expression levels of key genes, KRP1, KRP2, and KRP3, involved in regulating cell proliferation (Wang et al. 1998) in the abi5-7 and 35S:ABI5 lines to identify the downstream target genes of ABI5. RT-qPCR analysis indicated that the KRP1/2/3 transcripts were less abundant in abi5-7 plants but more abundant in the 35S:ABI5 line relative to wild type (Fig. 3A). Time-course RT-qPCR analysis of the wild-type plants showed that ABI5 transcripts increased with age under water-sufficient or -deficient conditions (Fig. 3B). A similar analysis of wild-type and abi5-7 plants indicated that KRP1 transcript abundance over time was considerably reduced in both abi5-7 whole plants (Fig. 3C) and abi5-7 inflorescence meristems (Fig. 3D) relative to that in wild type, implying that ABI5 may promote KRP1 expression with increasing age. Also, both KRP1 and ABI5 were expressed in SAMs (Ren et al. 2008; Yuan et al. 2014), suggesting that KRP1 and ABI5 functions overlap. Further, the transient expression data in tobacco (Nicotiana benthamiana) showed that ABI5 co-expression increased the expression of a KRP1pro-LUC reporter gene (Fig. 3E), indicating that ABI5 activates KRP1 expression. Overall, our findings suggest that ABI5 activates KRP1 expression.
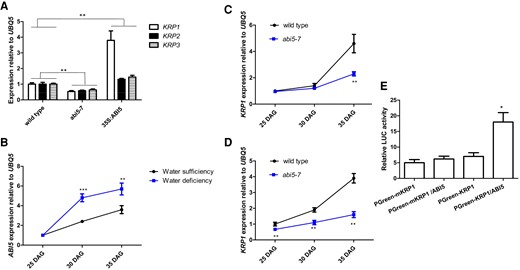
ABI5 activates KRP1 expression. A) Bar graph illustrating the differences in the expression levels of KRP1, KRP2, and KRP3 among wild-type, 35S:ABI5, and abi5-7 plants for 45 DAG. Quantifications of the expression levels of these genes in wild-type plants were set as 1.0. Error bars represent Sd (n = 3). Student's t-test (**P < 0.01). B) Bar graph illustrating differential expressions of ABI5 gene in wild-type plants at 25, 30, and 35 DAG grown in soil under water-sufficient or -deficient conditions. Quantifications of wild-type plants at 25 DAG were set as 1.0 in RT-qPCR analysis. Relative SWC is provided in Supplementary Fig. S1. Inflorescence meristems were collected as materials for time-course RT-qPCR. Error bars represent Sd (n = 3). Student's t-test (***P < 0.001; **P < 0.01). C, D) Bar graph illustrating differential expression of KRP1 in wild-type and abi5-7 whole plants (C) and inflorescence meristems (D) at 25, 30, and 35 DAG grown in soil under water-sufficient conditions. Quantifications of wild-type plants at 25 DAG were set as 1.0 in RT-qPCR analysis. Inflorescence meristems were collected as materials for time-course RT-qPCR. Error bars represent Sd (n = 3). Student's t-test (**P < 0.01). E) Bar graph illustrating transient expression of the 35S:ABI5 effector construct with the KRP1pro-LUC reporter construct in N. benthamiana leaves. Note PGreen-mKRP1 indicates the conserved sites (ACGT) of the K3–K4 region in KRP1 promoter were mutated. The activity of relative LUC represents arbitrary luminescence units, that is, expressing KRP1-LUC is ∼5, other expressions was quantified by using Adobe Photoshop CS (Adobe Systems) software, as described previously by Meng et al. (2015). Quantifications were normalized to UBQ5 expression. Error bars represent Sd (n = 3). Student's t-test (***P < 0.001; **P < 0.01; *P < 0.05). The statistical analyses described apply to all statistical analyses in this figure.
ABI5 directly binds to the promoter of KRP1
Our findings prompted us to examine whether ABI5 directly regulates KRP1 transcription. Examination of the KRP1 promoter revealed the presence of putative ABI5 binding sites (ABREs) (ACGT) (Cheng et al. 2014) at three or four possible locations in the KRP1 or KRP3 promoters that we labeled as sequences K1 to K4 and K1 to K3, respectively (Fig. 4A, Supplementary Fig. S4A). These DNA sequences within the KRP1 or KRP3 promoters were utilized for chromatin immunoprecipitation (ChIP) analyses. We employed transgenic plants expressing a MYC-tagged ABI5 for ChIP-qPCR assays. The DNA primers located at regions K3 and K4 of the KRP1 promoter (Fig. 4A) produced transcripts of greatest abundance (Fig. 4B). However, a KRP1 construct, 35S:ABI5–GFP/KRP1(mABREs)pro:KRP1 (which was transformed into the krp1/3 mutant with a mutant binding site for ABI5 [mABREs]) decreased ABI5 binding at the K3 and K4 regions of the KRP1 promoter containing mABREs (Fig. 4C).
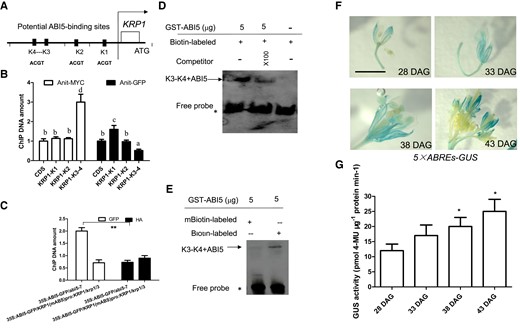
ABI5 directly binds to KRP1 promoter. A) Schematic of the KRP1 loci and a few amplicons initiating from ATG of KRP1. K1: −1 to −150 bp; K2: −120 to −280 bp; K3–K4: −540 to −690 bp. Red bars indicate that potential ABI5-binding sites. B) Bar graph illustrating ChIP-qPCR. ChIP-qPCR was performed to analyze the in vivo interaction between ABI5 and the KRP1 promoter. Anti-MYC antibody was used to facilitate precipitation of chromatin associated with 35S:ABI5-MYC/abi5-7. A two-way ANOVA was utilized to calculate significant differences (n = 3). Tukey's post-test was used, and different lowercase letters above the bars indicate significant differences at **P < 0.01. C) Bar graph illustrating ChIP-qPCR. ChIP-qPCR was performed to analyze the in vivo interaction between ABI5 and K3–K4 of KRP1 promoter. Enrichment of particular chromatin regions of K3–K4 in KRP1 promoter with anti-GFP antibody or anti-HA antibody (control) by using 35S:ABI5-GFP/KRP1(mABREs)pro::KRP1/krp1/krp3 or 35S:ABI5-GFP/abi5-7 seedlings at 28 DAG as materials, as detected by ChIP-qPCR analysis. D, E) Images illustrating unlabeled KRP1 promoter and unlabeled probes were used as competitors to determine the specificity of DNA-binding activity for ABI5. Free probe and ABI5 probe complexes are indicated by an asterisk and arrows, respectively (D). A mutant version of the KRP1 promoter (TGCA) was labeled with biotin and used for EMSA with ABI5 polypeptides (E). F) Images illustrating 5× ABREs (5× ABI5 binding site):GUS flowers of seedlings at 28, 33, 38, and 43 DAG grown on soil under constant water-sufficient conditions. GUS staining was performed to mark associated gene expression. Twenty flowers were hand pollinated at identical positions on secondary inflorescences of 5× ABREs:GUS plants to generate even-aged fruits. Bar = 1.0 cm. G) Bar graph illustrating ABI5 activities (indicated through 5× ABS-GUS activity) of the indicated seedlings in (F) were assayed quantitatively at the indicated time point. GUS activity was measured by picomoles of 4-methyl umbelliferone (4-MU) per mg protein per min. GUS activity of 8 DAG was used as control. Quantifications were normalized to the expression of UBQ5. Error bars represent Sd (n = 3). Student's t-test (**P < 0.01; *P < 0.05).
Further, an electrophoretic mobility shift assay (EMSA) was performed to determine if ABI5 can directly bind to the K3 and K4 sequences of KRP1 in vitro. The recombinant ABI5 protein bound to the labeled K3 and K4 elements in vitro (Fig. 4D). An excessive amount of unlabeled competitor K3 and K4 DNA effectively abolished this binding in a dose-dependent manner (Fig. 4D). The GST–ABI5 protein was unable to bind to mutated DNA probes of KRP1 (Fig. 4E). These data indicated that ABI5 directly bound the KRP1 promoter at ACGT motifs between the K3 and K4 sequences in vitro. Whereas K1 to K3 sequences in the KRP3 promoter contained at least one ABRE (Supplementary Fig. S4A), our ChIP-qPCR assays could not detect ABI5 association with this sequence of the KRP3 promoter (Supplementary Fig. S4B).
We further utilized a transgenic line containing the GUS reporter gene driven by tandem repeats of the ABRE sequence (ABI5 binding site), followed by a minimal 35S promoter (5 × ABREs:GUS/wild-type), which was utilized to check ABI5 transcriptional activities. GUS staining intensity in the flowers of the same stage of the plants at 28, 33, 38 and 43 DAG gradually increased over time under constant water-sufficient conditions (Fig. 4, F and G). Together, these data suggest that ABI5 directly binds to the promoter of KRP1 gene.
KRP1 is epistatic to ABI5 in the regulation of reproductive outputs
Since ABI5 directly binds to the KRP1 promoter to transcriptionally activate KRP1 expression (Figs. 3 and 4) and ABI5 function was associated with reproductive outputs of Arabidopsis plants (Fig. 1), we determined if KRP1 activity might regulate reproductive outputs.
The krp1-KO and krp3-KO single mutants were crossed to generate the krp1/krp3 double mutant line. Under water-sufficient conditions, a final total seed weight after completion of the plant life cycle was increased in krp1/krp3 plants but not in krp1-KO and krp3-KO plants. Further, total seed weight was decreased in the KRP1ox line relative to wild type (Supplementary Fig. S5A). By contrast, under water-deficient conditions, total seed weight was decreased in krp1/krp3 plants and increased in the KRP1ox line relative to wild type (Supplementary Fig. S5B). Together, our results indicate that KRP1/3 mediates the adjustment of plant reproductive outputs under water-deficient conditions.
We next examined the genetic interaction between ABI5 and KRP1. Under water-sufficient conditions, abi5-7 plants increased total seed weight relative to wild type, whereas 35S:KRP1 reduced total seed weight (Supplementary Fig. S6A). Further, abi5/35S:KRP1 plants decreased total seed weight relative to wild type, in a similar fashion to the 35S:KRP1 line (Supplementary Fig. S6A). These results indicate that over-expression of KRP1 inhibits the increase in total seed weight observed in the abi5-7 mutant. However, under water-deficient conditions, this phenomenon was reversed (Supplementary Fig. S6B).
Together, our findings indicate that KRP1 is epistatic to ABI5 in the adjustment of reproductive outputs under water-deficient conditions.
KRP1/3 adjusts plant reproductive outputs by controlling inflorescence meristem activities under water deficiency
To determine whether KRP1/3 adjusts plant reproductive outputs by controlling inflorescence meristem activities under water-deficient conditions, we scored WUSpro–GUS expression in reproductive tissues of wild type and krp1/3 plants under water-sufficient or -deficient conditions. WUS expression was either increased or decreased in krp1/3 inflorescence meristems of Arabidopsis plants at 30, 35, and 40 DAG under water-sufficient or -deficient conditions, respectively (Fig. 5, A and B). Consistent with these data, qPCR analysis of wild-type and krp1/3 inflorescence meristems at 30, 40, and 50 DAG (water-sufficient conditions) or at 25, 30, and 35 DAG (water-deficient conditions) produced similar results (Fig. 5, C to F), which were similar with WUS and STM expression patterns in abi5-7 plants (Fig. 2).

. The activities in krp1/3 inflorescence meristems were enhanced or reduced under water-sufficient or -deficient conditions, respectively. A, B) Images illustrating the expression of WUSpro-GUS in SAMs of inflorescences from wild-type and krp1/3 plants at 30, 35, and 40 DAG grown in soil under water-sufficient (A) or -deficient (B) conditions. Arrows indicate SAM. Bar = 200 µm. C to F) Bar graph illustrating time-course RT-qPCR for the differential expression of WUS and STM genes between wild-type and krp1/3 plants at 30, 40, and 50 DAG grown in soil under water-sufficiency (C, D) or 25, 30, and 35 DAG grown in soil under water-deficiency (E, F) conditions. Inflorescence meristems were collected as materials for time-course RT-qPCR. The expression of the WUS and STM genes in wild-type plants were set as 1.0. Quantification was normalized to the expression of UBQ5. Error bars represent Sd (n = 3). Student's t-test (***P < 0.001; **P < 0.01; *P < 0.05). WS, water sufficiency; WD, water deficiency.
Together, our findings suggest that KRP1/3 adjusts plant reproductive outputs by controlling inflorescence meristem activities under water-deficient conditions.
KRP1 physically interacts with STM to regulate reproductive outputs under water-deficient conditions
We next determined if the cyclin-dependent kinase KRP1 directly interacts with WUS and STM, or whether the transcription factor ABI5 directly binds to WUS and STM promoters, to regulate plant reproductive outputs under water-deficient conditions. Our ChIP-qPCR assays indicated that ABI5 did not directly bind to either of the WUS and STM promoters (Supplementary Fig. S7).
Full-length KRP1 was expressed as a HIS fusion protein and full-length WUS and STM were expressed as glutathione S-transferase (GST) fusion proteins. Following mixing of the fusion proteins, sefinose resin was used to bind selectively to the HIS–KRP1 fusion protein. The potential presence of the co-precipitated GST fusion protein was examined using a GST antibody. Our findings indicate that KRP1 may interact specifically to STM, but not to WUS, in vitro (Fig. 6, A and B).
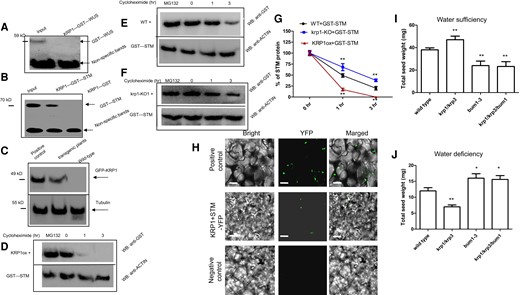
KRP1 interacts physically with STM, to inhibit STM stability. A) Images illustrating in vitro GST pull-down assays of the interaction between KRP1 and WUS. Anti-His immunoblot (IB) showed KRP1-His cannot be bound by the indicated WUS-GST protein. HIS or GST fusion proteins were mixed at 4 °C for 3 h (to guarantee full interaction between HIS and GST fusion proteins). Then, sefinose resin was added to obtain the interacting HIS fusion proteins. Western blot analysis was performed by using these purified materials. Anti-GST antibody (Abcam, China) was used for protein detection. The input (crude protein containing the GST–WUS protein) was used as the positive control. Nonspecific bands were used as control. B) Images illustrating in vitro GST pull-down assays of the interaction between KRP1 and STM. Anti-His immunoblot (IB) shows the amount of KRP-His bound by the indicated STM-GST protein. The input (crude protein containing the GST–STM protein) was used as the positive control. Nonspecific bands were used as control. C) Images illustrating in vivo put-down assays of the interaction between KRP1 and STM in transgenic Arabidopsis plants. Transgenic Arabidopsis plants containing KRP1ox–GFP (positive control) or KRP1ox–GFP/STMox-HA were extracted by using a protein extraction kit. The obtained crude protein was enriched through the addition of beads (Anti-HA Magnetic Beads, ChromoTeK, Germany) at 4 °C for 2 h. Western blot analysis was performed by using these materials. GFP-KRP1 was associated with membranes and can be detected with an anti-GFP antibody (Abcam, China). Tubulin was used a control. KRP1ox-GFP as materials for Western blot analysis was used as the positive control. Wild type was considered as the negative control in Western blot analysis. D to F) Images illustrating cell-free degradation assays proved that KRP1 destabilizes the STM protein. The recombined STM-GST protein was co-incubated with the isolated total proteins extracted from the KRP1ox (D), wild-type (WT) control (E), and krp1-KO1 (F) Arabidopsis plants. Moreover, the mixed proteins were treated with 25 μg/mL cycloheximide for 0, 1, or 3 h. The degradation of STM protein was assayed by using western blotting with an anti-GST antibody. MG132 was used as a positive control for stabilizing the STM protein. G) Bar graph illustrating the quantitation of the western blot data in (D) to (F). H) Images illustrating BiFC assay. Pairs of proteins of KRP1 and STM fused with halves of the YFP molecule were transiently expressed in wild-type N. benthamiana leaves and reconstituted YFP fluorescence was imaged in epidermis on the abaxial leaf blades. Plasmid containing YFP was used as a positive control. Only KRP1 fused with halves of the YFP molecule was used as a negative control. Bars = 40 μm. I, J) Bar graph illustrating total seed weight in in wild-type, krp1/3, bum1-3, krp1/krp3/bum1 plants were grown in soil under water-sufficient (I) or -deficient (J) conditions. Please note: total seed weight indicates a final total seed weight after completion of plant life cycle. Wild-type was used as a control in (I) and (J). Error bars represent Sd (n = 14 in I and J; n = 3 in G). Student's t-test (**P < 0.01; *P < 0.05). The statistical analyses described apply to all statistical analyses in this figure.
Further, we performed in vivo co-immunoprecipitation experiments. Following transformation and associated genetic crosses, transgenic plants expressing both HA–KRP1 and GFP–STM were generated. Subsequently, total soluble protein in wild type and GFP–STM transgenic seedlings was isolated and an anti-GFP antibody was utilized to potentially immunoprecipitate GFP–STM. Our findings indicated that the STM–green fluorescent protein (GFP) fusion interacted with KRP1 in these transgenic Arabidopsis plants in vivo, but not in wild type (Fig. 6C). KRP1 has been reported to locate to the nucleus (Bird et al. 2007) and STM is a homeodomain transcription factor (Kirch et al. 2003). To confirm that KRP1 interacts specifically to STM, we performed bimolecular fluorescence complementation (BiFC). Our results indicated that KRP1 specifically interacted with STM in BiFC experiments (Fig. 6H). Therefore, KRP1 may physically interact with STM in vivo.
To investigate if KRP1 impacts on the stability of STM, the recombinant expressed and purified GST–STM fusion proteins were incubated with plant total proteins extracted from KRP1ox, krp1-KO, and wild-type plants. Subsequently, protein degradation assays were performed. Our findings indicated that GST–STM proteins were degraded at a more rapid rate in the protein extracts from KRP1ox transgenic plants relative to the wild-type control (Fig. 6, D, E, and G). By contrast, GST–STM was more stable in the protein extracts derived from krp1-KO plants relative to wild type (Fig. 6, F and G). Together, these findings indicate that KRP1 may decrease STM stability following their interaction.
To demonstrate STM is epistatic to KRP1 in the regulation of reproductive outputs, we performed further experiments. Under water-sufficient conditions, whereas krp1/3 double mutant plants increased total seed weight, the loss-of-function mutant of STM, bum1-3, decreased total seed weight (Fig. 6I). Further, the krp1/krp3/bum1 triple mutant plants also reduced total seed weight under water-sufficient conditions (Fig. 6I). However, our findings were the opposite under water-deficient conditions (Fig. 6J). These findings indicate that STM acts downstream of KRP1 in the regulation of reproductive outputs under water-deficient conditions.
Together, our findings suggest that KRP1 physically interacts with STM to regulate reproductive outputs under water-deficient conditions.
Discussion
In this study, we focused only on rosette leaf number and open flower number to determine a final total seed weight after completion of the plant life cycle. Further, we applied an approach (Su et al. 2013) that increased plant survival and elevated plant reproductive outputs under constant water-deficient conditions (∼25% to 35% SWC). Under these parameters, we identified several key molecular components, ABI3, ABI4, ABI5, and KRP1, and an associated regulatory pathway, the ABI5–KRP1–STM module, involved in the control of plant reproductive outputs under water-deficient conditions. The cognate components and associated regulatory pathway can be considered as potential targets within the evolutionary strategies for adaptation to water-deficient conditions or specific drought regimes. Therefore, the molecular pathway identified ABA–KRP1–STM may provide opportunities to increase crop yields under water-deficient conditions or specific drought regimes.
Endogenous ABA is rapidly accumulated under water-deficient conditions (Supplementary Fig. S8D; Knight and Knight 2001), which in turn promotes the expression of the gene encoding the bZIP transcription factor, ABI5 (Wang et al. 2013). Subsequently, ABI5 directly binds to the KRP1 promoter, thereby activating its expression (Figs. 3 and 4) and in turn KRP1 interacts with STM (Fig. 6), to inhibit activities of inflorescence meristems (Figs. 2 and 5). These components thus form an ABI5–KRP1–STM module. Therefore, under water-deficient conditions, the ABI5–KRP1–STM module decreases reproductive outputs through inhibiting inflorescence meristem activities.
Under constant water-deficient conditions, stomatal aperture, stomatal conductance, and transpiration were elevated in abi5-7 and krp1/3 mutant plants relative to wild type (Supplementary Fig. S8, A to C), which leads to more water loss in abi5-7 and krp1/3 mutant plants. The resulting water-deficiency subsequently generated slightly more ABA in these mutant plants (Supplementary Fig. S8D; Léon-Kloosterziel et al. 1996) and in turn decreased inflorescence meristem activities through the ABI5–KRP1–STM module. The resulting decreased reproductive outputs in these mutants are produced under water-deficient conditions (Fig. 1, Supplementary Fig. S5). However, we have demonstrated that ABA metabolism and associated signaling mediate these reproductive outputs under water-deficient conditions (Fig. 1). Therefore, the ABI5–KRP1–STM module regulates inflorescence meristem activities predominantly through ABA metabolism and associated signaling (Fig. 7).
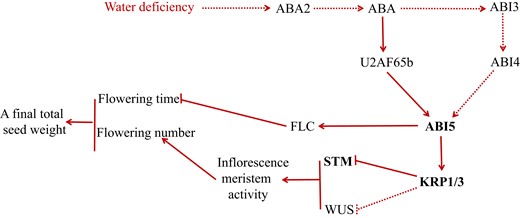
Proposed model is shown to illustrate how reproductive outputs in Arabidopsis plants are adjusted under constant water-deficient conditions. Under constant water-deficient conditions, ABA levels are enhanced and ABA signaling is transduced by the ABA2–ABA–ABI5–KRP1–STM/WUS pathway, leading to KRP1 upregulation. KRP1 upregulation with high ABA level inhibits cell proliferation, which in turn inhibits inflorescence meristem activity by directly suppressing the expression STM and WUS genes and thereby lowering flower number and a final total seed weight. As a result, reproductive outputs in plants are decreased under constant water-deficient conditions. Further, flowering time is inhibited by the ABA–U2AF65b–ABI5-FLC pathway (Xiong et al. 2019). Arrows and bars represent positive and negative regulation, respectively. Solid lines indicate direct regulation, whereas dotted lines indicate either indirect regulation or regulation in an unknown manner.
Previous observations lend some support to our model. ABA is a general negative regulator of Arabidopsis axillary bud growth and the aba2-1 mutant has more branching and bud outgrowth under water-sufficient conditions (Yao and Finlayson. 2015), suggesting the meristematic activity of the shoot apex is increased in aba2-1 mutant plants under water-sufficient conditions. ABA may inhibit plant cell division (Wang et al. 1998; Mcadam et al. 2016), implying cell division is promoted in organs such as the SAM in either aba3 or abi5 mutant plants under water-sufficient conditions. Further, time-course analysis (Robinson and Hill. 1999) revealed that the dry weight and number of fruits per plant, total leaf area per plant, and the number of rosette and cauline leaves per plant in abi3-1 mutants all were higher than those in the wild-type plants under water-sufficient conditions. Thus, suggesting that abi3-1 mutants have increased reproductive outputs under water-sufficient conditions, similar to that of the abi5-7 line (Fig. 1G). In addition, the absence of ABI5 function delayed senescence (Sakuraba et al. 2014) and increased seed size (Cheng et al. 2014) and the overexpression of KRP1 resulted in fewer flowers and fruits relative to wild type (Wang et al. 2000). Also, KRP1 is known to negatively regulate seed size (Cheng et al. 2013). Therefore, based on our data, we concluded that reproductive outputs are adjusted by the ABI5–KRP1–STM molecular module under water-deficient conditions.
In this study, we observed that the differences in soil or plant water status does not result in genotypic differences in reproductive outputs under water-sufficient conditions (Fig. 1, Supplementary Figs. S1 and S2), suggesting a direct impact of ABA signaling. By contrast, under water-deficit conditions, genotypic differences in reproductive outputs had a direct association with variation in plant water status (Fig. 1, Supplementary Figs. S1 and S2). Thus, an alternative explanation is that differences in leaf water status may modulate reproductive outputs, rather than ABA signaling per se (Sharp et al. 2000).
Materials and methods
Plant materials and growth conditions
The aba2-1 (Cheng et al. 2014), aba2-11 (Gonzalez-Guzman et al. 2002), abi5-7 (Wang et al. 2013; Yu et al. 2020b), abi3-1 (CS24) (Robinson and Hill. 1999), and abi4-1 (Shkolnik-Inbar et al. 2013) mutations were described previously.
The krp1/krp3 [krp1-KO1 (SALK_026391C) krp3-KO1 (KRP3B_144H6)] double mutant was obtained from adult-fertile F2 plants, which were krp1-KO1 (SALK_026391C) crossed with krp3-KO1 (KRP3B_144H6). The genotype was confirmed by RT-qPCR. The abi5-7/35S:KRP1 line was obtained from F2 plants, which were abi5-7 crossed with 35S:KRP1. The abi5-7/WUSpro-GUS, krp1/krp3/WUSpro-GUS, abi5-7/STMpro-GUS, and krp1/krp3/STMpro-GUS lines were obtained from F2 plants, which were WUSpro-GUS/wild-type (+) was crossed with abi5-7 and krp1/krp3 (−). These crossed lines were identified by GUS staining and the genotype confirmed by RT-qPCR. pCB308R-ABE-GUS and pMD111-KRP1pro-GFP were introduced into the wild-type background by Agrobacterium mediated-transformation and were identified and characterized. 35SABI5-GFP/KRP1(mABREs)pro::KRP1 were introduced into the krp1/krp3 background by Agrobacterium (Agrobacterium tumefaciens)-mediated transformation and then were identified and characterized, and used as material for ChIP-qPCR. 35S:ABI5-MYC were introduced into the abi5-7 background by Agrobacterium mediated-transformation, and then were identified and characterized, and used as material for ChIP-qPCR. The bum1-3 (CS3781) lines were identified by qPCR. All transgenic plants were generated using the A. tumefaciens-mediated floral dip method (Meng and Yao. 2015).
Plants grown in soil were maintained in a growth room under 16/8 h of light/dark cycle with cool white fluorescent light (140 μmol quanta PPFD m−2 s−1) at 21 ± 2 °C. Seedlings grown on MS medium were maintained in a controlled environment growth chamber under 16/8 h light/dark cycle with cool white fluorescent light at 21 ± 2 °C.
GUS assay and analysis of GUS activity
The GUS assays were described previously (Meng et al 2018c; Mu et al. 2022, 2023; Bao et al. 2023).
Water-deficient experiments
Experiments were undertaken as has been described as Su et al (2013) with minor revisions. For plants, wild-type and relative mutant/transgenic seeds were separately germinated at normal density in soil in 10 × 10 cm2 pots. All mutant/transgenic plants were well watered before bolting (the main stem was ∼1 cm high). To assay drought stress responses, two treatments (water sufficiency, ∼80% to ∼90% soil moisture or field capacity; water deficiency, ∼25% to 35% soil moisture or field capacity) were performed. Pots were randomly arranged. To water sufficient plants (control), ∼80% to 90% of the soil moisture was always kept until seed maturation or fruit dry. To water deficient plants, the content of the relative soil moisture rapidly declined; drought treatment was initiated by withholding water at 15 DAD, the content of the relative soil moisture arrived at ∼25% to 35% of the soil water-holding capacity. A controlled environment growth chamber (temperature: 23 °C [light]/19 °C [dark]; light intensity: ∼140 μmol quanta m−2 s−1 provided via fluorescent bulbs; relative humidity: ∼65%) was altered back to ∼65%. The soil water status was maintained via daily watering. In this context, pots were weighed and watered once per day until almost all fruits became dry and mature to harvest. During this process, the rosette leaf number and open flower number of wild type and mutant/transgenic plants at different rosette leaf number or sowing times was counted and analyzed. Mature seeds were weighted to gain a final total seed weight.
Assays of stomatal aperture, stomatal conductance, and transpiration
Stomatal aperture, soil RWC, leaf RWC, stomatal conductance, water loss, leaf area, and transpiration were tested, as was previously described (Meng et al. 2015; Meng and Yao. 2015).
Transient assays
To produce KRP1-LUC, its promoter was amplified by PCR, used primers are seen in Supplementary Table S1. To examine ABI5 activates KRP1 expression by LUC activity assay, we performed the below experiments. To generate proKRP1-LUC, the promoter was PCR amplified with primers proKRP1-F and proKRP1-R (ProKRP1-F-ttcctgcagcccgggggatccatgtattgatgcatgaaacctct; ProKRP1-R- tgtttttggcgtcttccatggcttcgatttaggttacgtgtgcgtg) for the gnomic DNA of Arabidopsis and inserted into the cloning site of the pGreen0800-LUC vector.
The MproKRP1-LUC construct containing mutations in the S2 sequence of the KRP1 promoter was generated using overlap extension PCR with primers (proKRP1-F: ttcctgcagcccgggggatccatgtattgatgcatgaaacctct, KRP1-mR1: gatgtcaagttgtcaacagcaGCtgtcgatcgtgatatattgggc, KRP1-mF2: gcccaatatatcacgatcgacaGCtgctgttgacaacttgacatc, KRP1-mR2: gttacggatagcctaaaaactaGCtacctgtgagtaaaataagg, KRP1-mF3: ccttattttactcacaggtaGCtagtttttaggctatccgtaac proKRP1-R: tgtttttggcgtcttccatggcttcgatttaggttacgtgtgcgtg), and inserted into pGreen0800-LUC vector.
Transient assays were performed as has been previously described (Meng et al. 2020).
Plasmid constructs
For obtaining 5× ABREs (ABS) binding site + minimal CaMV 35S promoter: this fragment (GACGCTCGTCATGCGGTACACGTGGCAATCTTGACGCTCGTCATGCGGTACACGTGGCAATCTTGACGCTCGTCATGCGGTACACGTGGCAATCTTGACGCTCGTCATGCGGTACACGTGGCAATCTTGACGCTCGTCATGCGGTACACGTGGCAATCTTGCAAGACCCTTCCTCTATATAAGGAAGTTCATTTCATTTGGAGAGAACACGGGGGACTCTTGA) was inserted into EcoRI + NcoI site of Pcambial301.
For obtaining plasmid of pMD111-KRP1pro-GFP, PHB-35S:KRP1-HA, pCB308R-KRP1-GUS, pCB308R-STM-GUS, and PHB-35S:STM-GFP, used primers are seen in Supplementary Table S1.
Endogenous ABA assay
To assay endogenous ABA contents under constant water-deficient conditions (∼25% to 35% SWC), a radioimmunoassay method was performed as previously described via Wang et al. (2011).
ChIP-qPCR
The 35S:ABI5-MYC line was used in this experiment. ChIP was performed using 6-wk-old seedlings as materials, as has been described by Meng and Yao (2015). MYC tag-specific monoclonal antibody and anti-GFP antibody were used for ChIP analysis. The ChIP DNA products were analyzed through qPCR using a few pairs of primers that were synthesized to amplify ∼100 to 300-bp DNA fragments in the promoter of KRP1, KRP3, STM, and WUS in ChIP analysis. Used primers are listed in Supplementary Table S1.
RT-qPCR
Total RNA was extracted from tissues indicated in the figures by the TRIZOL reagent (Invitrogen), as has been described in Meng et al (2018b). SYBR green was used to monitor the kinetics of qPCR product in RT-qPCR, as has been described in Meng et al (2018b).
For analyzing KRP1, KRP2, and KRP3 expression in wild type and mutant seedlings, used primers are listed in Supplementary Table S1.
Protein expression and purification
The plasmid pGEX-5X-1 was used in this experiment. The coding sequence of ABI5 was amplified by the primer pair. Used primers are shown in Supplementary Table S1. Protein expression and purification was described (Meng et al. 2020).
EMSA
The EMSA was performed by using the LightShift Chemiluminescent EMSA Kit (Pierce, 20148) according to the manufacturer's instructions. The biotin-labeled KRP1-K3-K4 DNA fragments (5′-acACGTgctgttgacaacttgacatcaggctaagcaaatacgcagggataacaaaaatataatttctagtgtcagttttacttttaccttattttactcacaggtACGTa-3′, 5′-tACGTacctgtgagagtaaaataaggtaaaagtaaaactgacact agaaattatattttttgttatccctgcgtatttgcttagcctgatgtcaagttgtcaacagcACGTgt-3′) and mutated KRP1-K3-K4 DNA fragments (5′-acACCTgctgttgacaacttgacatcaggctaagcaaatacgcagggataacaaaaatataatttctagtgtcagttttacttttaccttattttactcacaggtACCTa-3′, 5′-tAGGTacctgtgagagtaaaataaggtaaaagtaaaactgacactagaaattatattttttgttatccctgcgtatttgcttagcctgatgtcaagttgtcaacagcAGGTgt-3′) were synthesized, annealed, and used as probes, and the biotin unlabeled same DNA fragments as competitors in this assay. EMSA was performed, as was previously described (Meng et al. 2020).
GFP or YFP imaging
Confocal laser scanning microscopy with an Olympus IX-70 microscope (http://www.olympus-global.com/) for GFP or YFP imaging was performed, as was previously described (Meng et al. 2018c). That is, GFP or YFP fluorescence was observed following excitation by using a 488-nm laser and detected by using the bandpass 505 to 530 nm emission filter setting.
BiFC assays
To perform BiFC assays, pBI121-YFPC was generated through using primers; and pBI121-YFPN was produced by using primers; and pBI121-YFPN-ABI5 was generated through using primers; and pBI121-YFPC-STM was generated through using primers. Used primers are seen in Supplementary Table S1. BiFC assays were performed, as has been described (Meng et al. 2018c). That is, for performing BiFC, leaf blades of tobacco (N. benthamiana) were transiently transformed by using Agrobacterium (A. tumefaciens). The Agrobacterium strains with the BiFC constructs were grown on OD = 1.0, spun down, resuspended in infiltration buffer (10 mM MgCl2, 10 mM 2-(4-morpholino) ethanesulfonic acid (MES), and 100 mM acetosyringone) to OD = 1.3. Further, they were incubated on a shaker at room temperature for 2 to 3 h. Strains with the two assayed constructs were mixed and then injected into the middle of leaf blades. Leaf blades were imaged by laser scanning confocal microscope and DIC to 3 d after injection.
In vitro pull-down assay
HIS–KRP1 and GST–WUS or GST–STM expression constructs were prepared as described (Meng et al. 2018c). The potential for in vitro interaction between KRP1 and these GST fusion proteins was tested. In vitro pull-down assays were performed, as has been described (Meng et al. 2018a, 2018b, 2021). Used primers are listed in Supplementary Table S1.
In vivo pull-down assay (co-immunoprecipitation)
In vivo pull-down assays using wild type and transgenic plant extracts were performed according to Meng et al. (2018a, 2018b). Transgenic plants containing both HA–KRP1 and GFP–STM expression constructs were harvested. In vivo pull-down assay was performed, as has been described (Meng et al. 2018c).
Statistical analysis
Three independent experiments were performed with similar results and error bars represent Sd. Student's t-test was used to analyze the significance between two indicated samples at a significance level of 0.05 (***P < 0.001; **0.001 < P < 0.01; *0.01 < P < 0.05).
Accession numbers
Sequence data from this article can be found in the GenBank/EMBL data libraries under accession numbers ABI5 (AT2G36270), KRP1 (AT2G23430), and STM (AT1G62360).
Acknowledgments
The 35S:ABI5-MYC, aba2-1, aba2-11, abi5-7, abi5-8 (SALK_013163.24.70.X), abi3-1 (CS24) and abi4-1 seeds were kindly provided by Prof C.B. Xiang (University of Science and Technology of China, China). The krp1-KO (SALK_026391C), krp3-KO1 (KRP3B_144H6), 35S:KRP1 and 35S:KRP3 seeds were kindly provided by Prof H.G. Nam (Center for Plant Aging Research, Institute for Basic Science, and Department of New Biology, Daegu Gyeongbuk Institute of Science and Technology, Daegu, Republic of Korea). pGreen0800-LUC vector was kindly provided by Associate Prof Ziqiang Zhu (Nanjing Normal University, Nanjing, China). WUSpro-GUS seeds were kindly provided by Associate Prof Xudong Sun (Kunming Institute of Botany, Chinese Academy of Sciences, China). The bum1-3 (CS3781) seeds were obtained from the ABRC (Ohio State University). We thank that Dr Yi-Bo Wang kindly provided help.
Author contributions
L.S.M. designed experiments. L.S.M., S.T.Z., X.R.M., Y.T.W., Q.X.B., W.N.W., J.W.W., C.Y.G., X.R.L., Q.M.W., Y.X.L., J.L.Z., N.S., F.H., and Yu performed the experiments. L.S.M. and W.N.W. completed statistical analysis of data. L.S.M., J.H.J., and G.J.L. wrote, edited, and revised this manuscript.
Supplementary data
The following materials are available in the online version of this article.
Supplementary Figure S1. Relative SWC, leaf RWC, transpiration rates, water loss, and stomatal conductance were assayed.
Supplementary Figure S2. Reproductive outputs of ABA signaling mutant plants were adjusted under water-deficient conditions.
Supplementary Figure S3. Reproductive outputs of abi3-1 plants were adjusted under water-deficient conditions.
Supplementary Figure S4. ABI5 is not able to directly bind to promoters of KRP3 and CYCD3:1.
Supplementary Figure S5. Reproductive outputs of krp1/krp3 plants were adjusted under water-deficient conditions.
Supplementary Figure S6.ABI5 acts upstream of KRP1 in the regulation of reproductive outputs under water-deficient conditions.
Supplementary Figure S7. ABI5 is not able to directly bind to promoters of WUS and STM.
Supplementary Figure S8. Stomatal aperture, stomatal conductance, and transpiration in abi5-7 and krp1/3 mutant plants were enhanced under constant water-deficient conditions.
Supplementary Table S1. All primers used in this work.
Funding
This study was supported by grants from the Priority Academic Program Development of Jiangsu Higher Education Institutions. This study was also supported by National Natural Science Foundation of China (NSFC) (Grants 31960268).
Data availability
Data in this paper are available in the article and its online supplemental material.
Dive Curated Terms
The following phenotypic, genotypic, and functional terms are of significance to the work described in this paper:
References
Author notes
Wan-Ni Wang and Yu-Ting Wei contributed equally to this work.
The author responsible for distribution of materials integral to the findings presented in this article in accordance with the policy described in the Instructions for Authors (https://dbpia.nl.go.kr/plphys/pages/General-Instructions) is Lai-Sheng Meng ([email protected]).
Conflict of interest statement. None declared.