-
PDF
- Split View
-
Views
-
Cite
Cite
Dan Chen, Yarui Shi, Peng Zhang, Wenya Xie, Shuxin Li, Jinghua Xiao, Meng Yuan, Deletion of the sugar importer gene OsSWEET1b accelerates sugar starvation-promoted leaf senescence in rice, Plant Physiology, Volume 195, Issue 3, July 2024, Pages 2176–2194, https://doi.org/10.1093/plphys/kiae098
- Share Icon Share
Abstract
Leaf senescence is a combined response of plant cells stimulated by internal and external signals. Sugars acting as signaling molecules or energy metabolites can influence the progression of leaf senescence. Both sugar starvation and accumulation can promote leaf senescence with diverse mechanisms that are reported in different species. Sugars Will Eventually be Exported Transporters (SWEETs) are proposed to play essential roles in sugar transport, but whether they have roles in senescence and the corresponding mechanism are unclear. Here, we functionally characterized a sugar transporter, OsSWEET1b, which transports sugar and promotes senescence in rice (Oryza sativa L.). OsSWEET1b could import glucose and galactose when heterologously expressed in Xenopus oocytes and translocate glucose and galactose from the extracellular apoplast into the intracellular cytosol in rice. Loss of function of OsSWEET1b decreased glucose and galactose accumulation in leaves. ossweet1b mutants showed accelerated leaf senescence under natural and dark-induced conditions. Exogenous application of glucose and galactose complemented the defect of OsSWEET1b deletion-promoted senescence. Moreover, the senescence-activated transcription factor OsWRKY53, acting as a transcriptional repressor, genetically functions upstream of OsSWEET1b to suppress its expression. OsWRKY53-overexpressing plants had attenuated sugar accumulation, exhibiting a similar phenotype as the ossweet1b mutants. Our findings demonstrate that OsWRKY53 downregulates OsSWEET1b to impair its influx transport activity, leading to compromised sugar accumulation in the cytosol of rice leaves where sugar starvation promotes leaf senescence.
Introduction
As the final stage of plant leaf development, leaf senescence is characterized by developmentally programed leaf yellowing (Woo et al. 2019). Leaf senescence is a combined response of leaf cells to internal developmental signals and external environmental signals. The major internal factors are hormonal and nutritional signals. Phytohormones including salicylic acid, ethylene, abscisic acid (ABA), jasmonic acid, brassinosteroid, and strigolactone function as senescence inducers, whereas auxin, cytokinin, and gibberellic acid (GA) act as senescence inhibitors (Woo et al. 2019). Nutrition such as sugars not only functions as primary signaling molecules but also acts as essential cellular energy metabolites that can initiate and influence the progression of leaf senescence (Kim 2019).
Although sugars play critical roles in the regulation of leaf senescence, the effects of sugars on the modulation of senescence are debatable (van Doorn 2008; Asim et al. 2023). Some papers support that sugar starvation can induce or enhance leaf senescence which is supported by a series of evidence that either low sugar levels in leaf cells or prolonged dark treatment of detached leaves could accelerate senescence responses, while blocking sugar exportation from leaf cells or exogenous supply of sugars onto leaf disks could delay senescence responses (Li et al. 2020; Ni et al. 2020; Wu et al. 2023). Conversely, some papers show that sugar accumulation can promote leaf senescence which is backed by pieces of genetic or physiological evidence in some species (Zhou et al. 2014). The negative effects of sugar on the onset of leaf senescence are mainly evidenced by changes of the expression of senescence-associated genes (SAGs) and chlorophyll content or photosynthetic activity (Kim 2019). Moreover, the negative roles of sugar on leaf senescence regulation are mostly evident in dark-induced leaf senescence while rarely noticeable in sugar starvation-promoted leaf senescence (Li et al. 2020).
In general, sugars transported inside or outside of cells depend on sugar transporters. A class of sugar transporters named Sugars Will Eventually be Exported Transporters (SWEETs) which are conserved from archaebacteria to eukaryotes can facilitate the diffusion of sugar molecules across different cell membranes (Eom et al. 2015; Chen, Cheung, et al. 2015; Chen, Lin, et al. 2015). In plants, SWEETs play roles in multiple physiological processes including nutritional homeostasis (pollen, nectar, grain, and senescence), abiotic (cold, drought, heat, and salinity) stresses, and biotic (pathogen, insect) stresses (Eom et al. 2015; Chen, Cheung, et al. 2015; Chen, Lin, et al. 2015). SWEETs have been validated to function in remobilization of different sugars during plant senescence. In Arabidopsis (Arabidopsis thaliana), AtSWEET11, AtSWEET12, and AtSWEET15 act as sucrose exporters. The atsweet11; 12 double mutant contained higher levels of sucrose (Chen et al. 2012), while the AtSWEET11, AtSWEET12, or AtSWEET15 overexpression plants showed accelerated leaf senescence (Seo et al. 2011; Eom et al. 2015; Chen, Cheung, et al. 2015; Chen, Lin, et al. 2015). Rice (Oryza sativa L.) OsSWEET5 appeared to act as a galactose transporter when heterologously expressed in yeast. The OsSWEET5-overexpressing rice lines displayed precocious senescence and exhibited disordered sugar distribution with more contents of galactose, glucose, and fructose but less level of sucrose in leaves (Zhou et al. 2014). Similarly, the heterologous expression of pear (Pyrus bretschneideri) PbSWEET4 in strawberry (Fragaria vesca) resulted in reduced leaf sugar contents and accelerated leaf senescence which might be caused by PbSWEET4-promoted sugar efflux from leaves (Ni et al. 2020). The maize (Zea mays) zmsweet1b knockout mutant had decreased sucrose and fructose contents and exhibited a senescence-like phenotype (Wu et al. 2023). Of the 21 OsSWEET members in rice, 6 of them showed a decreased expression pattern and 5 of them exhibited an increased expression pattern along with natural leaf senescence, indicating they might play roles in sugar-regulated senescence (Yuan et al. 2014). However, whether these OsSWEETs have transportation activity on sugars and their specific substrates toward different sugars, even the relationship between leaf senescence and OsSWEET-mediated sugar transportation, is largely unknown.
Several types of transcription factors including WRKY proteins have been reported to regulate leaf senescence by activating or repressing the transcription of their downstream genes (Woo et al. 2019; Wang et al. 2023). WRKYs positively or negatively regulate leaf senescence depending on their diverse target genes. In Arabidopsis, AtWRKY6, AtWRKY53, AtWRKY55, and AtWRKY71 can directly target and activate the expressions of receptor-like protein kinase genes, senescence-induced receptor-like kinase (SIRK) and cysteine-rich receptor-like kinase 5 (CRK5), or SAGs, senescence-associated gene 13 (SAG13), senescence-associated gene 201 (SAG201), and stay-green (SGR), to positively influence senescence (Robatzek and Somssich 2002; Zhang et al. 2018; Wang et al. 2020; Yu et al. 2021; Burdiak et al. 2022). In rice, OsWRKY42 binds to the rice Metallothionein 1d (OsMT1d) promoter and represses its expression to promote senescence (Han et al. 2014). OsWRKY53 can directly target to the promoters of 2 ABA catabolic genes rice ABA 8′-hydroxylase 1 (OsABA8ox1) and OsABA8ox2 to repress their expressions, resulting in enhanced ABA accumulation which promotes leaf senescence (Xie et al. 2022). In general, WRKYs primarily regulate the expressions of SAGs or alter hormone homeostasis such as ABA to modulate senescence. However, whether WRKYs could regulate the expression of sugar transporters to alter sugar homeostasis in leaf cells to influence senescence is rarely reported.
It was previously reported that OsWRKY53 promotes leaf senescence by enhancing ABA-triggered hormonal signals; in this study, we supply evidence that OsWRKY53 modulates senescence by altering OsSWEET1b-mediated nutritional signals. We demonstrate that OsWRKY53 could directly bind to the promoter and repress the expression of OsSWEET1b which encodes a hexose transporter harboring influx transport activity for glucose and galactose. The compromised glucose and galactose accumulation in the ossweet1b mutant or the OsWRKY53-overexpressing plant accelerates leaf senescence. The revealed OsWRKY53-regulated OsSWEET1b regulatory network provides an additional module supporting the evidence that sugar starvation can enhance leaf senescence.
Results
The ossweet1b mutant shows accelerated leaf senescence
We previously generated individual homozygous knockout mutants for 19 rice SWEET genes (Li et al. 2022), of which the ossweet1b mutant showed obviously accelerated leaf senescence at the heading stage and later on. To explore how OsSWEET1b regulates leaf senescence, we generated OsSWEET1b-overexpressing (OsSWEET1b-OE) transgenic plants in this study and evaluated leaf senescence of these transgenic plants. Transformation of rice with the OsSWEET1b-OE construct where the maize Ubiquitin promoter was used to regulate OsSWEET1b expression generated 10 independent OsSWEET1b-OE T0 plants; RT-qPCR analyses showed that transcript levels of OsSWEET1b were significantly increased in these OsSWEET1b-OE transgenic plants (Supplementary Fig. S1).
We planted the OsSWEET1b-OE plants, ossweet1b mutants, and wild type (WT) in the paddy field to evaluate their leaf phenotypes by measuring Soil Plant Analysis Development (SPAD) and Fv/Fm values, ion leakage rate, and chlorophyll content in flag leaf at different growth stages. At the tillering stage, the ossweet1b mutant leaves were slightly yellow, while the OsSWEET1b-OE plants did not display noticeable phenotypic abnormalities compared with the WT (Fig. 1A; Supplementary Fig. S2). The ossweet1b mutants exhibited slightly lower SPAD and Fv/Fm values, higher ion leakage rates, and lower contents of chlorophyll a, chlorophyll b, and total chlorophyll than the WT, while the OsSWEET1b-OE plants had unchanged SPAD reading, Fv/Fm value, ion leakage rate, and chlorophyll contents relative to those of the WT (Fig. 1, B to E). At the heading stage, the ossweet1b mutant leaves were visibly yellow, especially the lower leaves were dark yellow, while the OsSWEET1b-OE plant leaves remained green (Fig. 1F; Supplementary Fig. S2). Accordingly, the ossweet1b mutants had decreased SPAD and Fv/Fm values, lower chlorophyll contents, and higher ion leakage rates, while the OsSWEET1b-OE plants had increased SPAD and Fv/Fm values, higher chlorophyll contents, and decreased ion leakage rates compared with those of the WT (Fig. 1, G to J). At the grain filling stage, the ossweet1b mutant leaves were totally yellow, while the OsSWEET1b-OE plant leaves remained green compared with slightly yellowish for WT leaves (Fig. 1K; Supplementary Fig. S2). In agreement, the ossweet1b mutants had 3.3-fold decreased SPAD reading, 1.8-fold decreased Fv/Fm value, 3.5-fold decreased chlorophyll a, 3.3-fold decreased chlorophyll b, 3.4-fold decreased total chlorophyll, and 2.7-fold increased ion leakage rate than the WT, while the OsSWEET1b-OE plants had 1.2-fold higher SPAD reading, 1.4-fold increased Fv/Fm value, 1.3-fold increased chlorophyll a, 1.4-fold increased chlorophyll b, 1.3-fold increased total chlorophyll, and 1.7-fold lower ion leakage rate than the WT (Fig. 1, L to O). From the tillering stage to grain filling stage, the ossweet1b mutant showed obviously accelerated leaf senescence supported by altered chlorophyll content, SPAD reading, Fv/Fm value, and ion leakage rate, while the OsSWEET1b-OE plant exhibited delayed leaf senescence, suggesting that OsSWEET1b is negatively involved in leaf senescence.
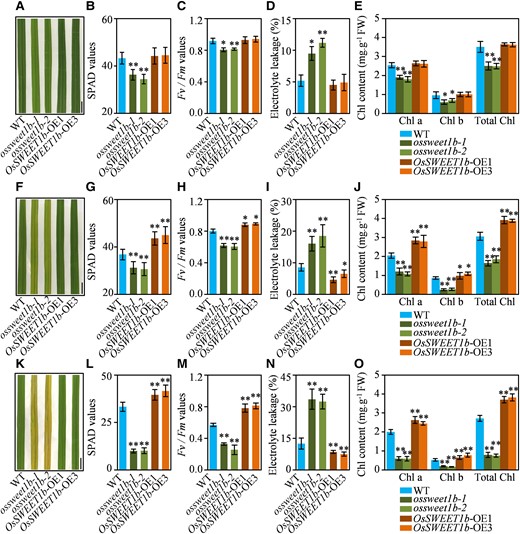
The ossweet1b mutant shows accelerated leaf senescence. A) Senescing flag leaf of OsSWEET1b-OE, ossweet1b, and WT at the tillering stage. B) SPAD values of flag leaf of OsSWEET1b-OE, ossweet1b, and WT at the tillering stage. C) Fv/Fm values of flag leaf of OsSWEET1b-OE, ossweet1b, and WT at the tillering stage. D) Electrolyte leakage analysis of flag leaf of OsSWEET1b-OE, ossweet1b, and WT at the tillering stage. E) Chlorophyll contents of flag leaf of OsSWEET1b-OE, ossweet1b, and WT at the tillering stage. F) Senescing flag leaf of OsSWEET1b-OE, ossweet1b, and WT at the heading stage. G) SPAD values of flag leaf of OsSWEET1b-OE, ossweet1b, and WT at the heading stage. H) Fv/Fm values of flag leaf of OsSWEET1b-OE, ossweet1b, and WT at the heading stage. I) Electrolyte leakage analysis of flag leaf of OsSWEET1b-OE, ossweet1b, and WT at the heading stage. J) Chlorophyll contents of flag leaf of OsSWEET1b-OE, ossweet1b, and WT at the heading stage. K) Senescing flag leaf of OsSWEET1b-OE, ossweet1b, and WT at the grain filling stage. L) SPAD values of flag leaf of OsSWEET1b-OE, ossweet1b, and WT at the grain filling stage. M) Fv/Fm values of flag leaf of OsSWEET1b-OE, ossweet1b, and WT at the grain filling stage. N) Electrolyte leakage analysis of flag leaf of OsSWEET1b-OE, ossweet1b, and WT at the grain filling stage. O) Chlorophyll contents of flag leaf of OsSWEET1b-OE, ossweet1b, and WT at the grain filling stage. Scale bars: 1 cm. Data represent mean ± Sd; n = 30 includes 3 biological replicates and 10 technical replicates for each biological replicate in B), C), D), G), H), I), L), M), and N). Data represent mean ± Se; n = 3 biological replicates in E), J), and O). Asterisks in B), C), D), E), G), H), I), J), L), M), N), and O) indicate a significant difference between transgenic plants and WT as determined by 2-tailed Student's t test at **P < 0.01 or *P < 0.05.
In order to validate that knocking out OsSWEET1b accelerates the leaf senescence, we kinetically analyzed the senescence symptoms of the transgenic rice plants from the seedling stage to the final senescence stage by measuring chlorophyll levels. There were similar chlorophyll levels between the ossweet1b mutants and WT at the seedling stage, while from the tillering stage to the senescence stage, the ossweet1b mutants had significantly decreased chlorophyll levels than the WT, and at the mature stage and senescence stage, chlorophyll levels in flag leaf of the ossweet1b mutants were almost undetected. Reversely, the OsSWEET1b-OE plants had higher chlorophyll contents than the WT in the flag leaf from the heading stage to the senescence stage (Supplementary Fig. S3). Although the ossweet1b mutants exhibited early senescence, they had similar heading dates as the WT and could undergo the whole process of growth and development (Supplementary Fig. S2). Therefore, we assume that the early senescence in the ossweet1b mutants is caused by functional loss of a senescence regulator but not developmental defect. Collectively, deletion of OsSWEET1b accelerates the leaf senescence.
Altered expression of genes involved in chlorophyll synthesis and degradation pathways
We investigated transcript levels of genes involved in chlorophyll synthesis and degradation pathways in flag leaf of the transgenic lines at the grain filling stage. Rice Mg-chelatase D subunit (OsChlD), heme biosynthesis A (OsHemA), and 3,8-divinyl(proto)chlorophyll(ide) α 8-vinyl reductase (OsDVR), encoding key enzymes for chlorophyll biosynthesis in rice (Zhang et al. 2006; Wang et al. 2010; Zeng et al. 2020), had decreased expressions in the ossweet1b mutant but increased levels in the OsSWEET1b-OE plant relative to WT levels (Fig. 2A). Reversely, rice red chlorophyll catabolite reductase 1 (OsRCCR1), nonyellow coloring 1 (OsNYC1), and stay-green (OsSGR), playing essential roles in chlorophyll degradation in rice (Jiang et al. 2007; Sato et al. 2009; Tang et al. 2011), had significantly enhanced expressions in the ossweet1b mutant but compromised transcriptions in the OsSWEET1b-OE plant than in the WT (Fig. 2B). Enhanced expression of genes associated with chlorophyll degradation and attenuated expression of chlorophyll biosynthesis-related genes in the ossweet1b mutant support accelerated leaf senescence for the mutant.
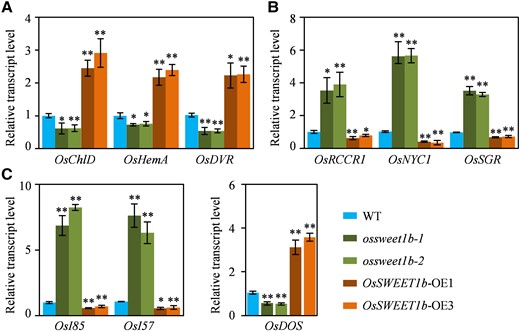
Transcript levels of representative chlorophyll synthesis genes, chlorophyll degradation genes, and SAGs in flag leaf at the grain filling stage. A) Transcript levels of chlorophyll synthesis genes. B) Transcript levels of chlorophyll degradation genes. C) Transcript levels of SAGs. Data represent mean ± Se for 3 biological replicates. Gene expression analysis was performed by RT-qPCR and normalized to Actin. Asterisks in A), B), and C) indicate a significant difference between transgenic plants and WT as determined by 2-tailed Student's t test at **P < 0.01 or *P < 0.05.
Altered expression of SAGs
We simultaneously investigated transcript level of representative SAGs in flag leaf of the transgenic lines at the grain filling stage. Rice delay of the onset of senescence (OsDOS), senescence-related indicator 57 (OsI57), and senescence-related indicator 85 (OsI85) are marker SAGs of leaf senescence. OsDOS negatively regulates leaf senescence (Kong et al. 2006). OsI57 and OsI85 expressions are induced during leaf senescence (Lee et al. 2001). RT-qPCR assays showed that both OsI57 and OsI85 had obviously enhanced expressions in the ossweet1b mutant while decreased expressions in the OsSWEET1b-OE plant than in the WT. OsDOS had reverse expressional pattern compared with OsI57 or OsI85 in the transgenic plants (Fig. 2C). Taken together, the altered expression of marker SAGs further supports that the early senescence in the ossweet1b mutants is caused by functional loss of a senescence regulator.
Abnormal ROS accumulation in the ossweet1b mutant
Since ROS generation is one of the earliest responses of plant cells under senescence, we speculated that the ossweet1b mutant may have altered ROS accumulation. To confirm our hypothesis, we stained flag leaf of the ossweet1b mutant, OsSWEET1b-OE plant, and WT with 3,3′-diaminobenzidine (DAB), a dye that has been widely used to indicate H2O2 accumulation. DAB staining showed that obvious red-brown precipitates were exclusively found in the ossweet1b mutant leaves, while no such precipitates were observed in the OsSWEET1b-OE plant or WT leaves (Fig. 3A). In agreement, there were 1.5- to 1.7-fold higher H2O2 contents in the ossweet1b mutant flag leaf than that of the WT, while the OsSWEET1b-OE plant had slightly decreased H2O2 content (Fig. 3B).
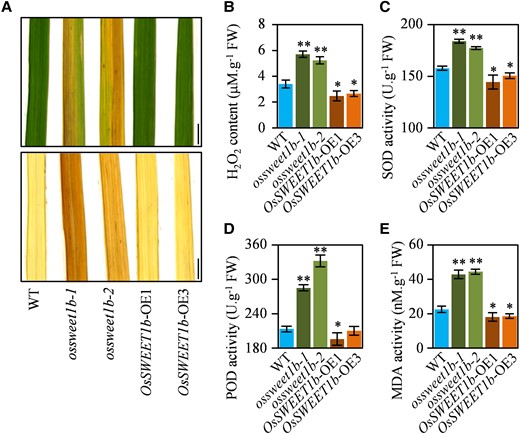
Impaired ROS homeostasis in the ossweet1b mutants. A) DAB staining for the OsSWEET1b-OE plants, ossweet1b mutants, and WT flag leaf. Scale bars: 1 cm. B) H2O2 levels in the transgenic rice plants and WT flag leaf. C) SOD activity in the transgenic rice plants and WT flag leaf. D) POD activity in the transgenic rice plants and WT flag leaf. E) MDA activity in the transgenic rice plants and WT flag leaf. Data represent mean ± Se of 3 biological replicates. Asterisks in B), C), D), and E) indicate a significant difference between transgenic plants and WT as determined by 2-tailed Student's t test at **P < 0.01 or *P < 0.05.
H2O2 accumulation is usually associated with disrupted ROS scavenging system in plants. To confirm the possibility, we measured activities of superoxide dismutase (SOD) and peroxidase (POD), 2 primary antioxidative enzymes, in flag leaf of these plants. Results showed that the ossweet1b mutant had significantly higher SOD and POD activities, while the OsSWEET1b-OE plant had slightly lower SOD and POD activities than the WT (Fig. 3, C and D). Moreover, we measured the accumulation of malondialdehyde (MDA) which indirectly reflects the changes of cell damage. Notably, the ossweet1b mutant had ∼2-fold higher MDA content, while the OsSWEET1b-OE plant had 1.2-fold lower MDA level than that of the WT (Fig. 3E). Collectively, the abnormal ROS accumulation in the ossweet1b mutant additionally suggests that knockout of OsSWEET1b accelerates rice leaf senescence.
Knockout of OsSWEET1b promotes dark-induced senescence
To further explore accelerated leaf senescence by knockout of OsSWEET1b in more detail, we evaluated the responses of transgenic plants under dark treatment. Detached leaf discs from OsSWEET1b-OE, ossweet1b, and WT plants were floated on MES solution and incubated in the dark to mimic dark-induced senescence. After 5 d of dark incubation (DDI), the ossweet1b leaf discs were yellowish, while OsSWEET1b-OE leaf discs remained green compared with light green of WT leaf discs (Supplementary Fig. S4A). Consistent with yellowing leaves, lower chlorophyll contents were observed for the ossweet1b mutant, while higher chlorophyll levels were determined for the OsSWEET1b-OE plant than the WT (Supplementary Fig. S4B), indicating that knockout of OsSWEET1b promotes dark-induced senescence.
Expression pattern of OsSWEET1b
The expression pattern of OsSWEET1b was investigated in different tissues throughout the growth period of rice planted in paddy fields. OsSWEET1b was constitutively expressed in all tissues examined (Supplementary Fig. S5A). From the vegetative growth stages including 3-wk-old seedling, the tillering and the booting stages, to the reproductive stages including the heading and grain filling stages, OsSWEET1b showed a substantially higher expression in leaves and sheaths than in other tissues. Moreover, the expressions of OsSWEET1b in leaves and sheaths were higher at the reproductive stages than at the vegetative growth stages. Simultaneously, we tested OsSWEET1b expression in leaves under natural senescence. OsSWEET1b transcripts decreased gradually along with rice leaf senescence (Supplementary Fig. S5B). In addition, we assessed OsSWEET1b expressions under biotic and abiotic stress treatments. OsSWEET1b did not respond to bacterial pathogens and fungal pathogen infection (Supplementary Fig. S5C), and also to drought and salt treatments (Supplementary Fig. S5D), while had slightly increased expression after cold treatments.
OsSWEET1b was predicted to localize to the plasma membrane, which was confirmed by transient transfection of the OsSWEET1b:GFP construct encoding a fusion between OsSWEET1b and the GFP into rice protoplasts. OsSWEET1b:GFP exclusively colocalized with secretory carrier membrane protein (SCAMP1) which was fused to the red fluorescent protein (RFP) as a plasma membrane-localized marker protein (Fig. 4A), suggesting OsSWEET1b is a plasma membrane protein.
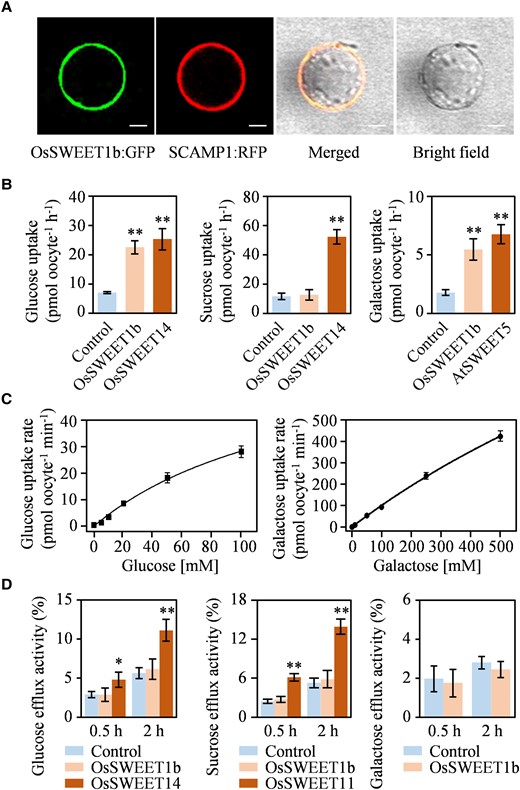
Sugar transporter activity of OsSWEET1b. A) Subcellular localization of OsSWEET1b:GFP in rice protoplasts. SCAMP1:RFP is used as a plasma membrane-localized marker protein. Scale bars: 10 μm. B) Uptake of glucose, sucrose, and galactose from Xenopus oocytes. C) Kinetics of OsSWEET1b for glucose and galactose uptake in Xenopus oocytes. D) Efflux of glucose, sucrose, and galactose from Xenopus oocytes. Data represent mean ± Sd of 8 biological replicates. Asterisks in B) and D) indicate a significant difference between control and SWEET protein as determined by 2-tailed Student's t test at **P < 0.01 or *P < 0.05.
OsSWEET1b is a hexose importer
SWEET family proteins transport various sugars across membranes (Eom et al. 2015; Chen, Cheung, et al. 2015; Chen, Lin, et al. 2015). OsSWEET1b was previously predicted functioning as a hexose transporter because it can rescue the growth of yeast mutant EBY.VW4000 which lacks sugar uptake ability in the medium supplemented with galactose (Yuan et al. 2014; Tao et al. 2015). Here, OsSWEET1b transcript increased after galactose treatment (Supplementary Fig. S5E), suggesting OsSWEET1b might be involved in sugar transportation. To determine the influx transport activity of OsSWEET1b for glucose, galactose, and sucrose, complementary RNAs (cRNAs) of OsSWEET1b, OsSWEET14 (serving as a positive control of influx transporter for glucose and sucrose; Chen et al. 2012), Arabidopsis AtSWEET5 (serving as a positive control of influx transporter for galactose; Wang et al. 2022), or water (serving as a negative control) were injected into Xenopus oocytes. The oocytes were exposed to 14C-labeled sugar ([14C]glucose, [14C]sucrose, or [14C]galactose), and the radioactivity in the oocytes was determined. OsSWEET1b showed influx activity for glucose and galactose but not for sucrose (Fig. 4B). A kinetic analysis showed that the Km values of OsSWEET1b for glucose and galactose uptake were 147 ± 29 and 2,073 ± 408 mM, respectively (Fig. 4C).
To determine whether OsSWEET1b has efflux transport activity, cRNAs of OsSWEET1b, OsSWEET14 (serving as a positive control of efflux transporter for glucose; Chen et al. 2012), OsSWEET11 (serving as a positive control of efflux transporter for sucrose; Chen et al. 2012), or water (serving as a negative control) were injected into Xenopus oocytes, and then, efflux activities were detected by monitoring time-dependent release of [14C]glucose, [14C]sucrose, or [14C]galactose from oocytes after injection of the 14C-labeled sugars and were expressed as percentage (sugar effluxed/total sugar injected × 100) to normalize the variations in injection, oocyte size, or cellular status. Results revealed that OsSWEET1b did not show efflux transport activity for glucose, galactose, and sucrose (Fig. 4D). Collectively, these results indicate that OsSWEET1b is a hexose transporter harboring influx transport activity for glucose and galactose.
Attenuated sugar contents in the ossweet1b mutant
To investigate the uptake activity of OsSWEET1b for sugar in planta, we measured contents of glucose, fructose, galactose, mannose, and sucrose in the flag leaf of these transgenic plants at different growth stages. From the tillering stage to grain filling stage, there were significantly higher glucose and galactose contents in the OsSWEET1b-OE plant but obviously lower levels of these 2 sugars in the ossweet1b mutant than the WT. However, comparable contents of fructose, mannose, and sucrose were observed between in the OsSWEET1b-OE plant or ossweet1b mutant and WT (Fig. 5A). In plants, glucose is largely converted into starch which is stored as energy. In line with higher glucose content in the OsSWEET1b-OE plant, we found the OsSWEET1b-OE flag leaf accumulated more starch at different growth stages than the WT. In contrast, the ossweet1b mutant flag leaf had less starch relative to WT (Supplementary Fig. S6A). Moreover, rice ADP-glucose pyrophosphorylase-encoding genes OsAGPS1 encoding the small catalytic subunit and OsAGPL1 and OsAGPL3 encoding the larger regulatory subunits of rice AGPase which is a key enzyme for starch synthesis (Ohdan et al. 2005) had significantly lower expressions in the ossweet1b mutant flag leaf while higher expressions in the OsSWEET1b-OE flag leaf than the WT at the grain filling stage (Supplementary Fig. S6B). These results suggest that OsSWEET1b is involved in glucose and galactose import in planta.
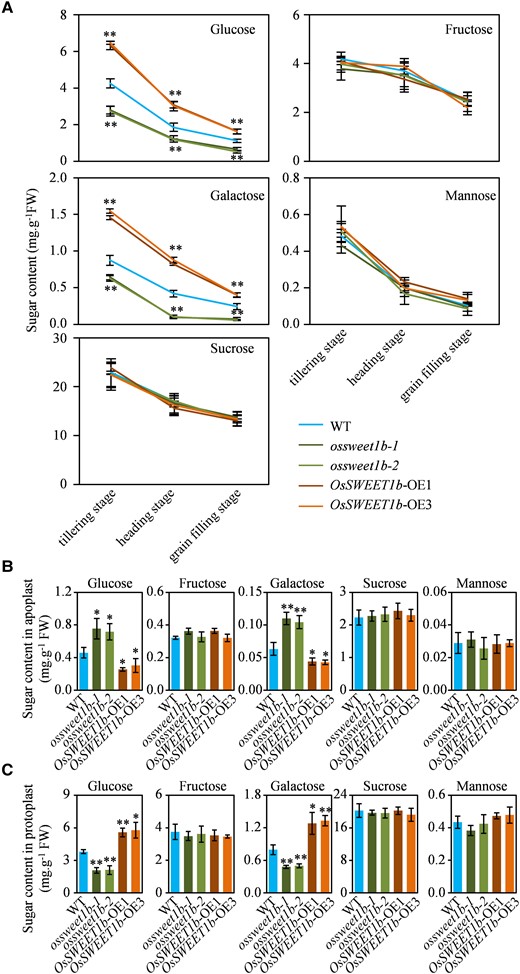
Sugar contents in leaves of the ossweet1b mutant and OsSWEET1b-OE plant. A) Sugar contents in the transgenic rice plants and WT flag leaf at the different growth stages. B) Apoplastic sugar contents in the transgenic rice plants and WT at 3-wk-old seedling stage. C) Sugar contents in protoplasts of the transgenic rice plants and WT at 3-wk-old seedling stage. Data represent mean ± Se of 3 biological replicates. Asterisks in A), B), and C) indicate a significant difference between transgenic plants and WT as determined by 2-tailed Student's t test at **P < 0.01 or *P < 0.05.
SWEET transporters on the plasma membrane facilitate sugar allocation (Eom et al. 2015; Chen, Cheung, et al. 2015; Chen, Lin, et al. 2015; Xue et al. 2022). OsSWEET1b acts as a plasma membrane-localized transporter and could import glucose and galactose. The above results reminded us that OsSWEET1b may translocate sugars from the extracellular apoplast into the intracellular cytosol. To test the possibility, we collected apoplastic fluid using the well-established infiltration–centrifugation method to assess apoplastic sugar content (Nouchi et al. 2012). Along with rice growing, highly silicified leaves make infiltration and apoplastic fluid collection challenging. Because of this, we used 3-wk-old seedling leaves to measure apoplastic sugar content. We found there were 1.6-fold higher glucose and 1.8-fold higher galactose in the apoplast of the ossweet1b mutant while 1.8-fold lower glucose and 1.4-fold lower galactose in the apoplast of the OsSWEET1b-OE plant relative to those in the WT apoplast. However, there were comparable contents of fructose, mannose, and sucrose in the apoplast of these plants (Fig. 5B). In addition, we measured sugar levels in the protoplast to indirectly evaluate sugar contents in cytosol. The were 1.9-fold less glucose and 1.7-fold less galactose in the protoplast of the ossweet1b mutant and 1.5-fold more glucose and 1.7-fold more galactose in the protoplast of the OsSWEET1b-OE plant than those in the WT protoplast. In contrast, there were comparable contents of fructose, mannose, and sucrose in the protoplast of these plants (Fig. 5C). Together, these results provide functional evidence that OsSWEET1b can translocate sugars like glucose and galactose from the extracellular apoplast into the intracellular cytosol.
Exogenous application of sugar delays the accelerated leaf senescence of ossweet1b mutant
To confirm whether the accelerated leaf senescence of the ossweet1b mutant was sugar dependent, we analyzed the response of the ossweet1b mutant to exogenous application of sugar under dark treatment. Detached leaf discs from the ossweet1b mutant and WT plants were floated on MES solution containing different sugars and incubated in the dark to mimic dark-induced senescence, with treatment without exogenous application of sugars as control. After dark incubation, the ossweet1b leaf discs were severely yellow (Fig. 6, A and D), accompanied by markedly decreased chlorophyll contents (Fig. 6, B and E), enhanced expression of OsI57, and attenuated expression of OsDOS than the WT (Fig. 6, C and F). Exogenous applications of glucose and galactose could substantially delay the senescence under dark treatment in the ossweet1b mutant and WT, as evidenced by the higher chlorophyll contents compared with mock-treated plants. However, both glucose and galactose application could retard the senescence of the ossweet1b mutant that the mutant even showed higher chlorophyll contents than mock-treated WT (Fig. 6, B and E). In agreement, the ossweet1b mutant had lower expression of OsI57 and higher expression of OsDOS after treatment of glucose or galactose than mock-treated WT (Fig. 6, C and F). Moreover, although exogenous applications of sucrose and fructose could delay the senescence under dark treatment in the ossweet1b mutant and WT, the increased chlorophyll contents were comparable in both the ossweet1b mutant and WT (Supplementary Fig. S7). Taken together, these results support the inference that accelerated leaf senescence of the ossweet1b mutant is due to the shortage of glucose or galactose.
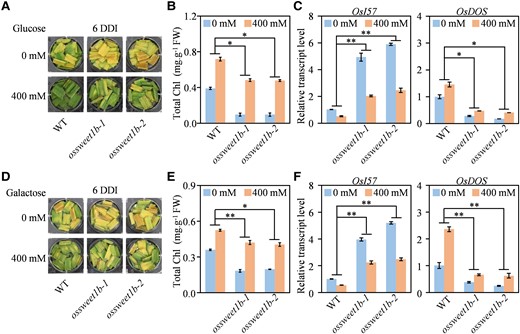
Exogenous applications of glucose and galactose delay the accelerated leaf senescence of ossweet1b mutant. A) Phenotype of detached leaf of ossweet1b and WT after dark treatment with or without additional glucose. DDI, day of dark incubation. B) Total chlorophyll content of ossweet1b and WT after dark treatment with or without additional glucose. C) Relative transcript levels of OsI57 and OsDOS in detached leaf of ossweet1b and WT after dark treatment with or without additional glucose. D) Phenotype of detached leaf of ossweet1b and WT after dark treatment with or without additional galactose. E) Total chlorophyll content of ossweet1b and WT after dark treatment with or without additional galactose. F) Relative transcript levels of OsI57 and OsDOS in detached leaf of ossweet1b and WT after dark treatment with or without additional galactose. Data represent mean ± Se of 3 biological replicates. Gene expression analysis was performed by RT-qPCR and normalized to Actin. Statistical significance was determined through 2-way ANOVA with Tukey's multiple comparisons test at **P < 0.01 or *P < 0.05.
OsWRKY53 directly represses OsSWEET1b transcription
To mine genetically upstream transcription factors that directly regulate OsSWEET1b transcription, the yeast 1-hybrid assay was performed using a 2 kb OsSWEET1b promoter and rice leaf-derived cDNA library. Yeast 1-hybrid assay showed that several transcription factors could bind to OsSWEET1b promoter (Supplementary Table S1), of which OsWRKY53, acting as a positive regulator of leaf senescence (Xie et al. 2022), was selected for further analysis in this study. In previous studies, the OsWRKY53-overexpressing plant showed accelerated leaf senescence and the oswrky53 knockout mutant exhibited delayed leaf senescence (Tian et al. 2017; Xie et al. 2022). OsWRKY53 functions as a transcription repressor by binding to the canonical W-box with TTGACC core sequence of its downstream target genes (Xie et al. 2021). Notably, the 2 kb OsSWEET1b promoter contains 3 putative W-box motifs which might be the OsWRKY53 binding sequence. To confirm OsSWEET1b is the target gene of OsWRKY53, we conducted a series of assays. Firstly, we tested OsWRKY53 expression in leaves under natural senescence. OsWRKY53 transcript levels increased gradually along with rice leaf senescence, showing an opposite pattern compared with OsSWEET1b (Supplementary Fig. S5B). We also evaluated OsWRKY53 expression under sugar treatment, with the result that OsWRKY53 transcript levels increased slightly but decreased soon after galactose treatment, while its expression did not respond to glucose treatment (Supplementary Fig. S5E). Simultaneously, we detected OsSWEET1b transcription in the flag leaf of OsWRKY53 transgenic plants. RT-qPCR results showed that OsSWEET1b had significantly decreased expression in the OsWRKY53-OE plant while increased expression in the oswrky53 mutant than the WT (Fig. 7A). Then, to determine the direct interaction between OsWRKY53 and promoter of OsSWEET1b, we employed electrophoretic mobility shift assays (EMSAs) to test the direct binding of OsWRKY53 to the W-box motif in vitro. A prokaryote-expressed and purified recombinant fusion protein consisting of His-TF and OsWRKY53 efficiently bound to W-box motif-containing probes but not mutated probes (Fig. 7B). Equal amounts of unlabeled and mutated W-box motif-containing probes could not efficiently impair the binding activity of OsWRKY53 on labeled W-box motif, while increasing amounts of unlabeled probes could weaken OsWRKY53 binding on labeled W-box motif (Supplementary Fig. S8). These EMSAs suggest that OsWRKY53 specifically binds to W-box motif within the OsSWEET1b promoter (Fig. 7B). Moreover, we performed chromatin immunoprecipitation (ChIP)-qPCR assays to test the binding of OsWRKY53 to these W-box motifs within the OsSWEET1b promoter in vivo. OsWRKY53 binding was enriched at the 2 W-box motifs (−1,048∼−1,043 and −194∼−189 bp) near the translational ATG start codon while not enriched at the third W-box (−1,733∼−1,728 bp) far away from the ATG in the OsSWEET1b promoter (Fig. 7C), suggesting OsWRKY53 bound to the OsSWEET1b promoter in vivo. In addition, to determine the effect of OsWRKY53 on the OsSWEET1b expression, we performed transient expression assays in rice protoplast cells using a construct in which OsWRKY53 is driven by the Ubiquitin promoter as an effector and a construct in which the firefly luciferase (LUC) gene is driven by the intact or mutated OsSWEET1b promoter as a reporter. The POsSWEET1b:LUC construct but not the mPOsSWEET1b:LUC construct resulted in significantly decreased LUC activity in protoplasts, confirming transcriptional repression for OsWRKY53 on OsSWEET1b (Fig. 7D). Collectively, these results indicate that OsWRKY53 can directly bind to the promoter of OsSWEET1b to suppress its transcription.
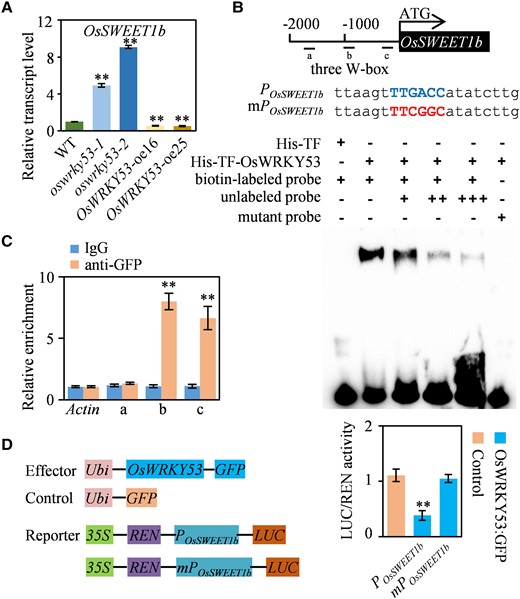
OsWRKY53 binds to OsSWEET1b promoter and suppresses OsSWEET1b transcription. A)OsSWEET1b expression in OsWRKY53-OE, oswrky53 mutants, and WT. B) DNA binding activity assay of OsWRKY53 by EMSA. The blue capital letters indicate the intact W-box, and the red capital letters represent the mutated W-box. C) Binding assay of OsWRKY53 to the promoter of OsSWEET1b by ChIP-qPCR in OsWRKY53:GFP plants using the anti-GFP antibody. Anti-GFP antibody was used for immunoprecipitation and IgG acted as a control. D) Activity assay of OsWRKY53 in regulating OsSWEET1b expression. Data represent mean ± Se of 3 biological replicates. Gene expression analysis was performed by RT-qPCR and normalized to Actin. Asterisks in A) and C) indicate a significant difference between transgenic plants and WT as determined by 2-tailed Student's t test at **P < 0.01 or in D) indicate a significant difference between control and reporter determined by 2-tailed Student's t test at **P < 0.01.
OsWRKY53 genetically acts upstream of OsSWEET1b
To assess the genetic epistasis between OsWRKY53 and OsSWEET1b in the regulation of senescence, we knocked out OsSWEET1b in the oswrky53 mutant to evaluate leaf phenotype of the oswrky53 ossweet1b double mutant (Supplementary Fig. S9). Compared with delayed leaf senescence of the oswrky53 knockout mutant, the oswrky53 ossweet1b double mutants exhibited accelerated leaf senescence, copying the ossweet1b mutant (Fig. 8A). In line with delayed leaf senescence, the oswrky53 mutant had higher SPAD reading, Fv/Fm value, and chlorophyll content and lower ion leakage rate in flag leaf than that of the WT. Reversely, the oswrky53 ossweet1b double mutants had decreased SPAD reading, Fv/Fm value, and chlorophyll content and increased ion leakage rate in flag leaf relative to that of the WT. Moreover, the oswrky53 ossweet1b double mutants had comparable parameters of senescence as the ossweet1b mutant (Fig. 8, B to E).
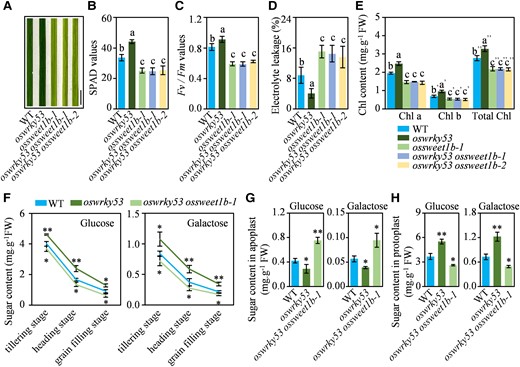
OsWRKY53 genetically acts upstream of OsSWEET1b. A) Leaf phenotype of oswrky53, ossweet1b, and oswrky53 osweet1b mutants at the heading stage. Scale bar: 1 cm. B) SPAD values of flag leaf of oswrky53, ossweet1b, and oswrky53 osweet1b mutants at the heading stage. C) Fv/Fm values of flag leaf of oswrky53, ossweet1b, and oswrky53 osweet1b mutants at the heading stage. D) Electrolyte leakage analysis of flag leaf of oswrky53, ossweet1b, and oswrky53 osweet1b mutants at the heading stage. E) Chlorophyll content of flag leaf of oswrky53, ossweet1b, and oswrky53 osweet1b mutants at the heading stage. F) Glucose and galactose contents in flag leaf of the oswrky53 and oswrky53 osweet1b mutants at the different growth stages. G) Apoplastic sugar contents in the oswrky53 and oswrky53 osweet1b mutants at 3-wk-old seedling stage. H) Sugar contents in protoplasts of the oswrky53 and oswrky53 osweet1b mutants at 3-wk-old seedling stage. Data represent mean ± Se of 3 biological replicates. The different letters above each bar in B), C), D), and E) indicate statistically significant differences, as determined by 1-way ANOVA analysis followed by Tukey's multiple test (P < 0.05). Asterisks in F), G), and H) indicate a significant difference between transgenic plants and WT as determined by 2-tailed Student's t test at **P < 0.01 or *P < 0.05.
Simultaneously, we assessed contents of glucose and galactose in the flag leaf of the oswrky53 single mutant and oswrky53 ossweet1b double mutant at different growth stages. From the tillering stage to the grain filling stage, both glucose and galactose contents decreased in all the investigated rice lines. However, the oswrky53 mutant had markedly higher and the oswrky53 ossweet1b double mutant had obviously lower glucose and galactose contents relative to them in the WT at the same growth stage (Fig. 8F). Moreover, the oswrky53 single mutant had lower glucose and galactose contents in the apoplast but more of them in the protoplast relative to WT. Reversely, the oswrky53 ossweet1b double mutant accumulated higher glucose and galactose levels in the apoplast but less of them in the protoplast compared with WT or the oswrky53 mutant (Fig. 8, G and H).
In addition, we measured different sugar contents in the flag leaf of the OsWRKY53 transgenic plants at different growth stages. From the tillering stage to heading stage and grain filling stage, higher contents of glucose and galactose were observed in the oswrky53 mutant, while lower levels of these 2 sugars were calculated in the OsWRKY53-OE plant relative to them in the WT (Supplementary Fig. S10). However, contents of fructose, mannose, and sucrose were comparable between the OsWRKY53-OE plant or oswrky53 mutant and WT (Supplementary Fig. S10). Since both the ossweet1b mutant and the OsWRKY53-OE plant accumulated less glucose and galactose, and all showed accelerated leaf senescence, we thus analyzed response of the OsWRKY53-OE plant to exogenous application of sugar under dark treatment to validate whether the accelerated leaf senescence of OsWRKY53-OE plant was sugar dependent. After dark incubation, the OsWRKY53-OE leaf discs showed severely yellowish, accompanying with decreased chlorophyll contents, activated OsI57 expression, and suppressed OsDOS expression than those in the WT (Supplementary Fig. S11). Exogenous applications of glucose and galactose could substantially delay the senescence under dark treatment in the OsWRKY53-OE plant and WT, as evidenced by the higher chlorophyll contents compared with mock-treated plants. However, both glucose and galactose application could delay the senescence of the OsWRKY53-OE plant that the transgenic plants even showed higher chlorophyll contents than mock-treated WT (Supplementary Fig. S11). In agreement, the OsWRKY53-OE plant had lower OsI57 expression and higher OsDOS expression after treatment of glucose or galactose than mock-treated WT (Supplementary Fig. S11). Collectively, these results support that OsWRKY53 genetically acts upstream of OsSWEET1b by regulating glucose and galactose import to modulate leaf senescence.
OsSWEET1b-mediated sugar import promotes agronomic traits
To investigate the effect of OsSWEET1b-mediated sugar import on rice growth and development, we planted the OsSWEET1b-OE plant and ossweet1b mutant in the paddy field along with WT and examined a set of agronomic traits of them. The ossweet1b mutants showed decreased plant height (−15%∼20%), tiller number (−43%∼52%), grain width (−9%∼11%), seed setting rate (−44%∼54%), panicle length (−16%∼18%), grain number per panicle (−50%∼54%), and 1,000-grain weight (−28% ∼ 32%) than the WT. Reversely, the OsSWEET1b-OE plants had increased panicle length (+5%∼10%) and grain number per panicle (+10%∼17%) than the WT, while no significant differences in plant height, tiller number, grain width, seed setting rate, and 1,000-grain weight were observed between the OsSWEET1b-OE plant and WT (Supplementary Fig. S12), suggesting that OsSWEET1b has positive effect on grain-related traits.
Discussion
Sugars are primary respiratory substrates in plant cells. However, along with natural leaf senescence, attenuated nutrient translocation such as insufficient sugars transportation from older leaves to young leaves can cause sugar starvation which accelerates the process (Kim 2019; Woo et al. 2019). Here, we reported that senescence-activated transcription factor OsWRKY53 suppresses the expression of OsSWEET1b, leading to deficient sugar accumulation in leaf cells where sugar starvation promotes leaf senescence.
Accumulating evidence shows that SWEETs largely prefer to transport sugars, such as sucrose, glucose, galactose, fructose, and mannose (Xue et al. 2022). It has been shown that 6 rice OsSWEETs can transport glucose and OsSWEET5 can transport galactose when tested in yeast or Xenopus oocytes (Xue et al. 2022). In this study, we demonstrate that OsSWEET1b harbors influx transport activity for glucose and galactose. The substantial evidence to support the conclusion is that (i) OsSWEET1b could transport glucose and galactose while not other sugars when tested in Xenopus oocytes using 14C-labeled sugars as substrates (Fig. 4), (ii) OsSWEET1b had influx transport activity but not efflux transport activity toward glucose and galactose when heterologously expressed in Xenopus oocytes (Fig. 4), (iii) loss-of-function of OsSWEET1b in planta could significantly decrease glucose and galactose accumulation in leaf cells (Fig. 5), and (iv) exogenous application of glucose and galactose could complement the defect of loss-of-function of OsSWEET1b-caused accelerated leaf senescence (Fig. 6). The biochemical and physiological assays together confirm that OsSWEET1b is a sugar importer.
SWEET-mediated sugar transportation alters sugar homeostasis in leaf cells which influences leaf senescence. Sugar starvation is positively associated with the process of leaf senescence that has been reported for overexpressing of sugar exporters AtSWEET11, AtSWEET12, and AtSWEET15 in Arabidopsis and PbSWEET4 in strawberry or deletion of sugar importer ZmSWEET1b in maize (Seo et al. 2011; Eom et al. 2015; Chen, Cheung, et al. 2015; Chen, Lin, et al. 2015; Ni et al. 2020; Wu et al. 2023). Our data support that OsSWEET1b acts as a sugar importer translocating glucose and galactose from the extracellular apoplast into the intracellular cytosol. Glucose and galactose could not be sufficiently transported into cytosol, resulting in sugar starvation in the ossweet1b mutants (Fig. 5). In line with previously reported that SWEET-mediated sugar starvation promotes leaf senescence, the ossweet1b mutants showed accelerated leaf senescence compared with delayed leaf senescence of the OsSWEET1b-OE plants (Fig. 1). Interestingly, both OsSWEET5 and OsSWEET1b could transport galactose testing in yeast; however, the OsSWEET5-overexpressing transgenic plants and the ossweet1b mutants had a similar precocious senescence phenotype. We suppose the conflict between these 2 rice SWEETs may be caused by their different sugar transport activity or ability as they belong to different SWEET family clades (Yuan et al. 2014). ZmSWEET1b is an ortholog of OsSWEET1b. The zmsweet1b knockout mutants accumulated less sucrose and fructose and had a senescence phenotype (Wu et al. 2023). We infer that OsSWEET1b and ZmSWEET1b might experience divergent selection during rice and maize evolution; thus, they may employ different sugars as their substrates. Moreover, senescence processes are largely linked to energy reutilization in plants; sugars have been suggested as the key signaling molecules that influence the progression of leaf senescence. Sugar sensors are proposed to sense exogenous sugars involved in signal transduction to delay senescence (Kim 2019). Moreover, numerous studies have reported that low sugar levels could accelerate senescence responses; supplementing sugars in developmentally senescing leaves could suppress or delay the senescence responses (Wingler et al. 2009; Kim 2019; Li et al. 2020). Rice Hxk1 is involved in hexose-sensing process at the early stage of leaf senescence (Li et al. 2020). OsSWEET4, OsSWEET5, and OsSWEET15 could transport galactose in yeast (Yuan et al. 2014; Tao et al. 2015); OsSWEET14 is an influx transporter for glucose (Chen et al. 2012). Whether Hxk1 or these SWEET proteins play roles in reverting the senescence for ossweet1b mutants is unclear. In addition, several plant SWEETs have also been shown to be involved in the movement of phytohormones, such as Arabidopsis AtSWEET13 and AtSWEET14, and rice OsSWEET3a could mediate cellular GA uptake (Kanno et al. 2016; Morii et al. 2020), and barley (Hordeum vulgare) HvSWEET11b could transport cytokinin (Radchuk et al. 2023). Although OsSWEET1b could not uptake GA in yeast (Morii et al. 2020), whether it can transport GA or cytokinin in planta is unclear, since GA and cytokinin are senescence inhibitors (Woo et al. 2019). Therefore, we could not rule out the possibility that OsSWEET1b might also transport GA or cytokinin to influence the progression of senescence.
Previously, we found that OsWRKY53 participates in ABA-induced leaf senescence (Tian et al. 2017; Xie et al. 2022), and it has an opposite expressional pattern compared with OsSWEET1b in leaf along with natural leaf senescence (Yuan et al. 2014; Xie et al. 2022). Here, we update the underlying molecular mechanism for OsWRKY53-regulated leaf senescence that OsWRKY53 directly targets OsSWEET1b to alter sugar homeostasis to influence senescence, which is supported by the following evidence: (i) the OsSWEET1b expression was significantly increased in the oswrky53 mutant while decreased in the OsWRKY53-overexpressing plants (Fig. 7A), (ii) the direct bind of OsWRKY53 to the promoter of OsSWEET1b was validated by EMSA and ChIP assays (Fig. 7), (iii) suppression of OsWRKY53 on OsSWEET1b expression was confirmed by transient expression assay (Fig. 7D), (iv) knocking out of OsSWEET1b in the oswrky53 mutant could impair the delayed senescence phenotype of oswrky53 mutant (Fig. 8), (v) there were comparable glucose and galactose contents in flag leaf of the oswrky53 mutant and the OsSWEET1b-OE plants or the ossweet1b mutant and the OsWRKY53-overexpressing plants (Figs. 5 and 8), and (vi) exogenous applications of glucose and galactose could comparably decelerate the senescence of both the ossweet1b mutant and the OsWRKY53-overexpressing plants (Fig. 6). These biochemical, genetic, and physiological assays synthetically confirm that OsWRKY53-regulated OsSWEET1b module is involved in leaf senescence. Moreover, we previously showed that OsWRKY53 regulates leaf senescence by enhancing ABA accumulation (Xie et al. 2022). Thus, published results and present data demonstrate that OsWRKY53 regulates different downstream target genes to alter ABA and sugar signaling pathways to modulate leaf senescence (Fig. 9). There are complex interactions between ABA and sugar modulating the onset or progress of leaf senescence (Wingler and Roitsch 2008). Although the OsSWEET1b transgenic plants had similar expression of ABA biosynthetic genes and comparable ABA content compared with WT (Supplementary Fig. S13), whether there is crosstalk between ABA and sugar in OsWRKY53-regulated leaf senescence should be deciphered further. In addition, a number of hormone-related biosynthetic or metabolic genes have altered expression levels in the OsWRKY53 transgenic plant leaves (Chujo et al. 2014; Xie et al. 2021, 2022). Therefore, functioning as a transcriptional repressor, OsWRKY53 may also target other genes or alter other hormone homeostasis to regulate leaf senescence. The corresponding target genes and the underlying mechanism are waiting for uncovering.
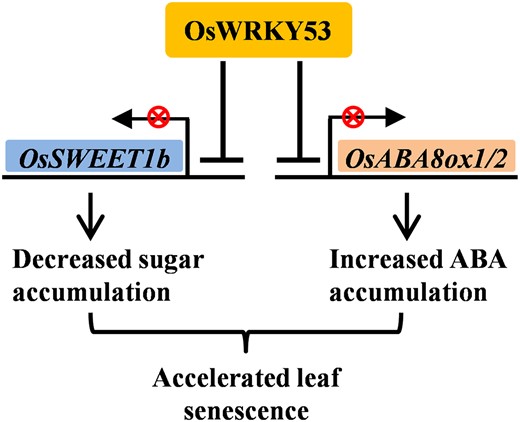
Proposed working model of OsWRKY53-regulated senescence. OsWRKY53 binds and suppresses the expressions of OsSWEET1b and OsABA8ox1 or OsABA8ox2, causing decreased sugar accumulation and increased ABA accumulation in leaf cells, respectively. The attenuated sugar content and enhanced ABA level coordinately promote leaf senescence.
Sugars, acting as the main supervisor and key sources of energy, are involved in general development of plants from the stage of embryogenesis to senescence. Evidence supports a role of sugar starvation in the initiation and/or acceleration of leaf senescence (Kim 2019; Woo et al. 2019; Li et al. 2020). Moreover, intercellular and intracellular sugars have long been considered to act as important signal molecules that can generally affect fundamental cellular processes including senescence or regulate gene expression during leaf senescence (Wingler et al. 2009; Asim et al. 2023). Knocking out of OsSWEET1b caused glucose and galactose retention in the extracellular apoplast, resulting in sugar starvation in the intracellular cytosol. We conjectured that sugar starvation-caused energy shortage and sugar starvation-initiated senescence signal might accelerate leaf senescence in the ossweet1b mutant, which should be verified in the future. Moreover, the high Km value for OsSWEET1b suggests that OsSWEET1b is a low-affinity transporter and it has influx activity in a pretty high amount of sugars. In line with that, the ossweet1b mutants exhibiting substantially accelerated senescence from the tillering stage to the senescence stage may reflect that OsSWEET1b functions mainly at the corresponding stages when rice plants accumulate abundant sugars (Kim 2019). However, whether other rice SWEET members have synergistic or antagonistic effects along with OsSWEET1b in the process of sugar starvation-promoted leaf senescence might be explored, especially at the stages when leaves accumulate a large amount of sugars.
Delaying the progress of leaf senescence has been associated with improved crop yields and utilized as an alternative breeding strategy (Thomas and Ougham 2014). It is worth noting that both the OsSWEET1b-OE plants and the oswrky53 mutants had delayed the progress of leaf senescence and increased grain-related traits (Tian et al. 2017; Xie et al. 2021, 2022). Thus, modulating the expressions of OsWRKY53 or OsSWEET1b might be an applicable strategy for genetic improvement.
In summary, our findings verify that OsSWEET1b acts as a sugar transporter capable of translocating glucose and galactose from the extracellular apoplast into the intracellular cytosol. The senescence-activated transcription factor OsWRKY53 directly binds to the promoter of OsSWEET1b and suppresses its expression, leading to compromised glucose and galactose accumulation in leaf cells where sugar starvation accelerates leaf senescence.
Materials and methods
Plant materials and growth conditions
The OsWRKY53-OE and OsWRKY53:GFP plants and oswrky53 and ossweet1b knockout mutants were generated previously in rice (O. sativa ssp. Geng) cultivar ‘Zhonghua 11’ (ZH11) background (Xie et al. 2021; Li et al. 2022). The OsSWEET1b-OE transgenic lines were generated in ZH11 as described below. The oswrky53 ossweet1b double mutants were generated by deleting OsSWEET1b in the oswrky53 mutant. The seeds were sown on the seedbed, and 1 mo later, germinated seedlings were transplanted to the paddy field in the experimental station on campus. All rice materials were planted in regular growing season from May to October with field management and fertilizer application as described previously (Chu et al. 2022).
Plant treatments
The dark-induced leaf senescence experiments were performed as described previously (Xie et al. 2022). In brief, the transgenic rice lines and WT were planted in soil and grown 1 mo in a controlled environment plant growth chamber under a 14 h light/10 h dark cycle at 28 °C. Detached leaves were cut into 1 cm pieces, immersed in 2 mM MES solution at pH 5.7, and incubated in complete darkness for 5 d. Rice plants at the tillering stage were inoculation with bacterial pathogens Xanthomonas oryzae pv. oryzae strain PXO99 and X. oryzae pv. oryzicola strain RH3 and fungal pathogen Magnaporthe oryzae isolate 99-20-2 and were sampled at the designated times as described previously (Tian et al. 2023). Seedlings at the 4-leaf stage were treated with drought (stopping the water supply), salt (irrigation with 200 mM NaCl solution), and cold (4 °C growth chamber) and were sampled at the designated times as described previously (Lv et al. 2017). The calli of ZH11 were treated with sugars (incubation with 100 mM glucose or galactose) and sampled for a certain period of time.
Chlorophyll measurement
Rice leaves from the transgenic rice lines and WT at different growth stages were sampled for chlorophyll measurement (Xie et al. 2022). About 100 mg samples were incubated in extraction solution (absolute ethanol:acetone:water, 4.5:4.5:1, v/v/v) for 12 h; then, the extraction was measured spectrophotometrically at 645 and 663 nm using a Spark Multimode Microplate Reader (Tecan, Switzerland). Total chlorophyll (mg/g) = (20.29A645 + 8.05A663) × v/m × 1,000, where v is the volume of extraction solution and m is the mass of leaves.
SPAD and Fv/Fm measurement
The uppermost fully expanded leaf from the transgenic rice lines and WT growing in the paddy field at the tillering stage, heading stage, and grain filling stage was marked for SPAD and Fv/Fm measurement (Tian et al. 2023). A chlorophyll SPAD-502 meter was used to assess SPAD value that 3 SPAD readings per leaf were taken around the midpoint and again 40 mm away on both sides of the midpoint. The OS30p+ Chlorophyll Fluorometer was used to measure Fv/Fm value. SPAD reading and Fv/Fm value for each plot was determined by taking the average of 30 readings.
Ion leakage rate measurement
The detached leaves were cut into 1 cm piece and submerged in 10 mL of deionized water in test tubes for 12 h at room temperature. A DDSJ-308A conductivity meter was used for measuring the initial conductivity (R1). Then, the test tubes were placed in boiling water for 30 min and naturally cooled to room temperature; the conductivity (R2) was measured again. The R1/R2 was calculated as ion leakage rate (Xie et al. 2022).
Sugar influx and efflux activity in Xenopus oocytes
Transport activity of OsSWEET1b was determined as previously reported (Chen et al. 2012; Wang et al. 2022). In brief, cRNA was prepared by T7 polymerase using the mMESSAGE mMACHINE kit. Fifty nanoliters of cRNA or RNase-free water was injected into each oocyte. For sugar influx assay, 2 d after injection, 8 oocytes were transferred into tubes containing 200 µL of Na-Ringer buffer (2 mM KCl, 115 mM NaCl, 1.8 mM CaCl2, 1 mM MgCl2, and 10 mM HEPES-Tris, pH 7.5) with 100 mg/L gentamycin and 100 µM sucrose (4 µCi/mL [14C]sucrose), glucose (4 µCi/mL [14C]glucose), or galactose (4 µCi/mL [14C]galactose). After incubation 2 h at 18 °C, the oocytes were washed with cold sucrose in Na-Ringer buffer 3 times and then extracted by 0.1 M HNO3, and the radioactivity was calculated by a liquid scintillation analyzer. For sugar efflux assay, 2 d after cRNA injection, oocytes were further injected with 50 nL of solution containing 1 mM sucrose (0.15 µCi/mL [14C]sucrose), glucose (0.15 µCi/mL [14C]glucose), or galactose (0.15 µCi/mL [14C]galactose). Eight oocytes per replicate were transformed into tubes and immediately washed with cold Na-Ringer buffer 3 times. The cells were then incubated in 500 µL of Na-Ringer buffer, and the external buffer was collected at 0.5 and 2 h. The radioactivity of external solution and oocytes was determined by a liquid scintillation analyzer. The efflux activity was expressed as a percentage [radioactivity in external solution/(radioactivity in external solution + radioactivity remaining in the oocytes) × 100].
Measurement of sugar and starch contents
Rice flag leaves from the transgenic rice lines and WT at the tillering stage, heading stage, and grain filling stage were sampled for sugar and starch measurement. About 200 mg samples were ground in liquid nitrogen and extracted with a solution of 1.6 mL methanol:chloroform:water (5:2:2, v/v/v) via incubation for 30 min at 70 °C. After ultrasound treatment for 90 min, the samples were centrifuged at 4,000 rpm for 10 min; then, the supernatant was collected and mixed with 200 μL of methyl-α-D-glucopyranoside as an internal standard. After centrifugation at 12,000 rpm for 15 min, 0.5 mL of supernatant were collected and dried in a vacuum concentrator at 60 °C. The dried metabolites were orderly derivatized with hydroxylamine hydrochloride, hexamethyl disilylamine, and trimethylchlorosilane and transferred to glass vials for Q Exactive Plus Hybrid Quadrupole-Orbitrap Mass Spectrometer (ThermoFisher Scientific, United States) analysis with the parameters as reported previously (Li et al. 2022). Leaves of 3-wk-old rice seedlings were harvested for apoplastic fluid extraction. Apoplastic fluid extraction was performed as described previously (Nouchi et al. 2012). In brief, leaves were cut in small fragments and soaked in a surfactant solution containing 0.05% (v/v) Triton X-100 for 2 min. After rinsing with deionized water, fragments were infiltrated using a 60 mL syringe with a 3-way stopcock for 3 min. The syringe was then set in a sealant injector with the inner pressure of the syringe kept at maximum pressurization for 5 min. Then, the leaf fragments were inserted into a centrifuge tube and centrifuged at 6,000 × g for 15 min at 4 °C. The apoplastic fluid flowed out from the leaf fragments and collected at the bottom of the centrifuge tube using a micropipette. Rice protoplasts were isolated using 3-wk-old seedlings as described previously (Xie et al. 2021). The starch content in flag leaf was extracted via the DMSO/HCl method and measured enzymatically using a starch assay kit according to the manufacturer's instruction (Megazyme, Bray, Ireland).
Gene expression analysis
The TRIzol reagent (Invitrogen, United States) was used to isolate total RNA from various rice tissues at different growth stages when rice plants ZH11 were grown in a paddy field. The mRNA was reverse-transcribed into cDNAs using MonScript RTIII All-in-One Mix (Monad, China) according to the manufacturer's protocol. qPCR was performed using MonAmp SYBR Green qPCR Mix (Monad, China) in the ABI QuantStudio (Applied Biosystems, United States). Gene-specific primers were designed using primer analysis software Primer Express v3.0 (Applied Biosystems, United States). Transcript level of rice actin gene was used to normalize expression level for target genes (Supplementary Table S2). Each RT-qPCR assay was performed with 3 independent biological replicates, with each repetition having 3 technical replicates. The differences were analyzed for statistical significance using 2-tailed Student's t test.
Yeast 1-hybrid assay
The Matchmaker Gold Yeast One-Hybrid System (Clontech, United States) was used for screening putative transcription factors binding to OsSWEET1b promoter. A 2 kb OsSWEET1b promoter upstream of the initiation codon of OsSWEET1b gene was cloned into pAbAi vector and transformed into the gold strain (Y1HGold) to generate a bait-reporter yeast. The yeast strain was then transformed with the pGAD424-based rice leaf cDNA library. Transformants were spread on SD/-Leu medium, and positive colonies were picked for plasmid extraction.
ChIP-qPCR
The ChIP assays were conducted as described previously. In brief, chromatin was extracted from the OsWRKY53:GFP seedlings (Xie et al. 2021) and fragmented to 200 to 400 bp via ultrasound. The OsWRKY53:GFP protein bonding DNAs were enriched by protein A Dynabeads (Invitrogen, United States) coupling with anti-GFP antibody or IgG as control at 4 °C overnight. After extensive washing and decrosslinking, the input and precipitated DNA samples were amplified by qPCR using gene-specific primers (Supplementary Table S2).
Recombinant protein production
The coding sequence of OsWRKY53 was amplified and cloned into the pCold vector using the gene-specific primers (Supplementary Table S2). Both the recombinant plasmid and control plasmid were transformed into Escherichia coli BL21(DE3) cells; then, cells were induced with 0.2 mM isopropyl β-D-1-thiogalactopyranoside (IPTG) overnight at 16 °C and collected by centrifugation (Xie et al. 2021). The recombinant His-TF-OsWRKY53 protein and control His-TF protein were purified using Ni Sepharose 6 fast Flow (GE Healthcare, United States).
EMSA
EMSA assays were conducted using LightShift Chemiluminescent EMSA Kit (Thermo Scientific, United States) as described previously (Xie et al. 2021). In brief, the 5-carboxyfluorescein (FAM) biotin-labeled probes were synthesized and incubated with purified His-TF-OsWRKY53 recombinant proteins for 30 min at room temperature. The unlabeled probes were mixed with labeled probes for competition reaction. The mutant probes were used as negative control. The protein–DNA complex was separated on a 6% (v/v) PAGE gel for 2 h at 4 °C in the dark. Gels were photographed using FLA-5100 (FUJIFILM, Japan).
Transient expression assays in rice protoplasts
OsSWEET1b promoter (2 kb upstream of the translational ATG start codon) was amplified by PCR using gene-specific primers (Supplementary Table S2) and then cloned into the pGreen II 0800 vector to generate the POsSWEET1b:LUC construct as reporter. Moreover, the mPOsSWEET1b:LUC construct was generated by mutating the 2 W-box motifs (−1,048∼−1,043 and −194∼−189 bp) from TTGACC to TTCGGC using the GeneTailor Site-Directed Mutagenesis System (Invitrogen Life Technologies, United States) at the OsSWEET1b promoter. The coding sequence of OsWRKY53 was amplified with gene-specific primers (Supplementary Table S2) and cloned into the pU1301 vector to generate the Ubi:OsWRKY53 construct as effector. The reporter and effector constructs were cotransfected into rice protoplasts as described previously (Xie et al. 2021). After culturing in the dark for 16 h at 25 °C, the transfected protoplasts were collected by centrifugation at 100 × g for 8 min. The LUC activities were measured by a Spark Multimode Microplate Reader (Tecan, Switzerland) using Dual Luciferase Reporter Assay System (Promega, United States) according to the manufacturer's instructions. The relative reporter gene expression levels were expressed as the ratio of firefly LUC to renilla luciferase (REN).
Subcellular localization assay
OsSWEET1b full-length cDNA without the translational stop codon was amplified using the specific primers (Supplementary Table S2); then, the amplified PCR products were inserted into the pM999-GFP vector between the KpnI and BamHI restriction sites (Yang et al. 2022), to generate the OsSWEET1b:GFP construct where OsSWEET1b was in-frame and upstream of the green fluorescent protein (GFP) that was driven by the CaMV 35S promoter. The OsSWEET1b:GFP construct was transiently transformed into rice protoplasts isolated from 20-d-old green rice leaf sheaths via polyethylene glycol (PEG)-mediated transient protoplast transformation (Yang et al. 2022). The protoplasts were incubated at 28 °C for 12 h and then were visualized for fluorescent signals by a Leica Microsystem (LAS AF, Germany) with GFP excited at 488 nm and detected at 500 to 530 nm according to a previously reported protocol (Yang et al. 2022).
DAB staining
Rice plants were grown in the paddy field till to the grain filling stage; flag leaf was sampled and used for DAB staining according to previously described protocol (Chu et al. 2022). In brief, DAB was dissolved in a buffer containing 10 mM 2-(N-morpholinol)ethanesulfonic acid and 0.2% (v/v) Tween 20 to a final concentration of 1 mg/mL. Leaves were vacuum-infiltrated with DAB solution for 12 h in the dark and then immersed in ethanol to remove chlorophyll in a water bath at 60 °C overnight.
Histochemical experiments
Flag leaf of rice plants at the grain filling stage were sampled and immediately frozen in liquid nitrogen. The contents of hydrogen peroxide (H2O2), SOD, POD, and MDA were measured using assay kits (Elabscience, United States), according to the manufacturer's instructions. All the measurements were biologically repeated with 3 times.
Statistical analysis
Statistical parameters are represented in the figures and figure legends. The differences between samples were analyzed for statistical significance using Student's t test or ANOVA.
Accession numbers
Sequence data from this article can be found in the Rice Genome Annotation Project (RGAP) data libraries under the following accession numbers: OsSWEET1b (LOC_Os05g35140), OsWRKY53 (LOC_Os05g27730), OsDOS (LOC_Os01g09620), OsI57 (LOC_Os02g57260), and OsI85 (LOC_Os07g34520).
Acknowledgments
We are grateful to Professor Shaowu Xue at Huazhong Agricultural University for assistance in Xenopus oocytes assays.
Author contributions
M.Y. designed the research. D.C. and Y.S. performed most of the experiments. W.X., P.Z., S.L., and J.X. assisted the experiments. M.Y. and D.C. analyzed the data and drafted the manuscript. M.Y. wrote and revised the manuscript.
Supplementary data
The following materials are available in the online version of this article.
Supplementary Figure S1. Relative transcript levels for OsSWEET1b in the OsSWEET1b-OE transgenic lines.
Supplementary Figure S2. Phenotype of OsSWEET1b-OE, ossweet1b, and WT at the different growth stages.
Supplementary Figure S3. Temporal changes of chlorophyll levels in the flag leaf of OsSWEET1b-OE, ossweet1b, and WT at the different growth stages.
Supplementary Figure S4. Knockout of OsSWEET1b promotes dark-induced senescence.
Supplementary Figure S5. Expression pattern of OsSWEET1b.
Supplementary Figure S6. Altered starch contents and transcript levels of starch synthesis genes in flag leaf of OsSWEET1b transgenic plants.
Supplementary Figure S7. Exogenous applications of sucrose and fructose did not delay the accelerated leaf senescence of ossweet1b mutant.
Supplementary Figure S8. Binding activity of OsWRKY53 on the W-box motif by EMSA.
Supplementary Figure S9. Characterization of the oswrky53 ossweet1b double mutant.
Supplementary Figure S10. Sugar contents in flag leaf of the oswrky53 mutant and OsWRKY53-OE plant at different growth stages.
Supplementary Figure S11. Exogenous applications of glucose and galactose delayed the accelerated leaf senescence of OsWRKY53-OE plants.
Supplementary Figure S12. Agronomic traits in grains of the ossweet1b mutant and OsSWEET1b-OE plant grown in field.
Supplementary Figure S13. Transcript levels of ABA biosynthetic genes and ABA contents in flag leaf of OsSWEET1b transgenic plants.
Supplementary Table S1. Putative transcription factors associated with OsSWEET1b promoter.
Supplementary Table S2. Primers used in this study.
Funding
This work was supported by grants from the National Natural Science Foundation of China (31821005 and 32172421), the Major Project of Hubei Hongshan Laboratory (2022hszd016), the Natural Science Foundation of Hubei Province (2023AFA043), and the Fundamental Research Funds for the Central Universities (2662023PY006).
Dive Curated Terms
The following phenotypic, genotypic, and functional terms are of significance to the work described in this paper:
References
Author notes
Dan Chen and Yarui Shi contributed equally.
Meng Yuan Senior author.
The author responsible for distribution of materials integral to the findings presented in this article in accordance with the policy described in the Instructions for Authors (https://dbpia.nl.go.kr/plphys/pages/General-Instructions) is Meng Yuan ([email protected]).
Conflict of interest statement. None declared.