-
PDF
- Split View
-
Views
-
Cite
Cite
Xin Liu, Wojciech J Nawrocki, Roberta Croce, The role of the pigment–protein complex LHCBM1 in nonphotochemical quenching in Chlamydomonas reinhardtii, Plant Physiology, Volume 194, Issue 2, February 2024, Pages 936–944, https://doi.org/10.1093/plphys/kiad555
- Share Icon Share
Abstract
Nonphotochemical quenching (NPQ) is the process that protects photosynthetic organisms from photodamage by dissipating the energy absorbed in excess as heat. In the model green alga Chlamydomonas reinhardtii, NPQ is abolished in the knock-out mutants of the pigment–protein complexes LHCSR3 and LHCBM1. However, while LHCSR3 is a pH sensor and switches to a quenched conformation at low pH, the role of LHCBM1 in NPQ has not been elucidated yet. In this work, we combined biochemical and physiological measurements to study short-term high-light acclimation of npq5, the mutant lacking LHCBM1. In low light in the absence of this complex, the antenna size of PSII was smaller than in its presence; this effect was marginal in high light (HL), implying that a reduction of the antenna was not responsible for the low NPQ. The mutant expressed LHCSR3 at the wild-type level in HL, indicating that the absence of this complex is also not the reason. Finally, NPQ remained low in the mutant even when the pH was artificially lowered to values that can switch LHCSR3 to the quenched conformation. We concluded that both LHCSR3 and LHCBM1 are required for the induction of NPQ and that LHCBM1 is the interacting partner of LHCSR3. This interaction can either enhance the quenching capacity of LHCSR3 or connect this complex with the PSII supercomplex.
Introduction
All photosynthetic organisms need to deal with constantly changing environmental factors. Changes in light intensity are particularly critical since light is the source of energy but can also become the source of damage. In low light (LL), most of the photons absorbed by the pigments associated with the photosynthetic complexes lead to charge separation in the reaction centers of Photosystem I (PSI) and II (PSII). In high light (HL), when the capacity of the photosynthetic reactions is saturated, the photons absorbed in excess can lead to PSII photoinhibition (Tyystjarvi 2013; Nawrocki et al. 2021) and the production of harmful species that can damage the photosynthetic apparatus (Erickson et al. 2015; Li et al. 2018). To adapt to the rapid light changes, all photosynthetic organisms have developed a range of photoprotection mechanisms.
A process called nonphotochemical quenching (NPQ) dissipates as heat a large part of the energy absorbed in excess (Ruban 2016, 2018). In the model green alga Chlamydomonas reinhardtii, NPQ activation depends on the presence of the light-harvesting stress-related complex 3 (LHCSR3) (Peers et al. 2009). This complex is expressed only upon exposure of the cells to HL in ambient CO2 concentration in photoautotrophic growth conditions (Allorent et al. 2013; Polukhina et al. 2016; Redekop et al. 2022; Ruiz-Sola et al. 2022). At variance with PsbS, the central protein for NPQ in plants (Li et al. 2000; Fan et al. 2015), LHCSR3 binds pigments and switches from a light-harvesting to a quenched state upon protonation of its lumen-exposed residues (Bonente et al. 2011; Liguori et al. 2013). The switch is triggered by the acidification of the lumen driven by photosynthetic electron transfer—the rate of which depends in light-limiting conditions on the antenna size of the photosystems. LHCSR3 has thus been proposed to act both as a pH sensor and quencher (Bonente et al. 2011; Tian et al. 2019). In addition to LHCSR3, another stress-related protein, LHCSR1, was also shown to induce quenching at low pH (Dinc et al. 2016).
The LHCSRs are members of the light-harvesting complex multigenic family, containing all the peripheral antenna complexes of green algae and plants (Engelken et al. 2010; Niyogi and Truong 2013). These integral membrane proteins accommodate up to 18 pigments (chlorophylls a and b and carotenoids) and serve as an antenna of both PSI and PSII (Croce and van Amerongen 2020; Pan et al. 2020). In C. reinhardtii, the major light-harvesting complexes (LHCBMs) are encoded by the lhcbm1–lhcbm9 genes (Merchant et al. 2007). The nine LHCBM complexes are divided into four subgroups according to their sequence homology: type I (LHCBM3/4/6/8/9), type II (LHCBM5), type III (LHCBM2/7), and type IV (LHCBM1) (Minagawa and Takahashi 2004).
The individual LHCBMs have similar biochemical and biophysical properties (Natali and Croce 2015) but were suggested to have different roles (Takahashi et al. 2006; Nguyen et al. 2008; Drop et al. 2014a, b; Grewe et al. 2014; Girolomoni et al. 2017). In particular, LHCBM1 was shown to be involved in photoprotection since the C. reinhardtii npq5 mutant, deficient in this subunit, exhibits low NPQ (Elrad et al. 2002; Ferrante et al. 2012). However, the role of LHCBM1 in NPQ is at present unknown. In this work, we use biochemical and physiological approaches to investigate the factors hampering the NPQ development in the absence of LHCBM1, analyzing the photoprotection capacities and photosynthetic properties in the npq5 mutant during high-light adaptation.
Results
To confirm that npq5 is an antenna mutant, we compared the antenna composition in LL-grown cells of wild-type (WT) (CC-425) and npq5 (Fig. 1). LHCBM1 was undetectable in npq5 in LL (Fig. 1A), in agreement with previous results (Elrad et al. 2002). The LHCBMs/CP47 protein ratio in npq5 was ∼20% lower than in WT (CC-425) (Fig. 1B). This difference is mainly due to the absence of LHCBM1 since the level of the other LHCBMs is similar to that of WT (CC-425) (Supplemental Figs. S1 and S2). The absence of LHCBM1 in the mutant is thus not compensated by an increase of other LHCBMs.
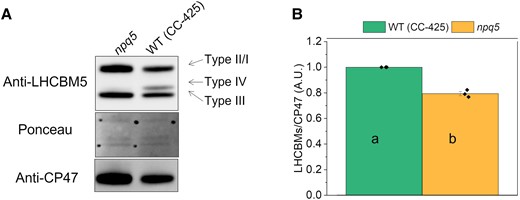
PSII antenna quantification in LL grown cells. Immunoblot with anti-LHCBM5 and -CP47 antibodies A) and protein quantification B) are shown for cells grown in low light (<15 μmol photons m−2 s−1, 0 h HL). The different types (I to IV) of LHCBMs are indicated in A). Note that the anti-LHCBM5 antibody reacts with all types of LHCBMs. Densitometry data of each protein were normalized to the WT (CC-425) before calculating the ratio in B). Data shown are mean ± the standard error of the mean (SEM), n = 3 biological replicates. Different lowercase letters between means denote a statistically significant difference (P < 0.05; see the Materials and methods section for details).
To study the role of LHCBM1 in photoprotection, we measured the photosynthetic properties of the npq5 mutant and its control strain (WT CC-425) during HL exposure. The cells pre-grown in tris-acetate-phosphate (TAP) media in LL (20 μmol photons m−2 s−1) were resuspended in high salt medium (HSM), kept for 1 h in LL (0 h HL) and then exposed to HL (500 μmol photons m−2 s−1). Aliquots were collected at 0 h HL, 24 h HL, and 48 h HL for the analysis.
PSII antenna size and composition upon high-light acclimation
Several functional parameters were measured before and during the HL treatment. The value of Fv/Fm, which is a proxy for the status of PSII, decreased significantly during HL, suggesting that the cells were partially photoinhibited (Fig. 2A). The Fv/Fm value of npq5 was identical to its control in LL and after 24 h of HL and only slightly lower after 48 h (Fig. 2A). These data indicate that npq5 is relatively well protected in HL, although less than its parental strain in the long run.
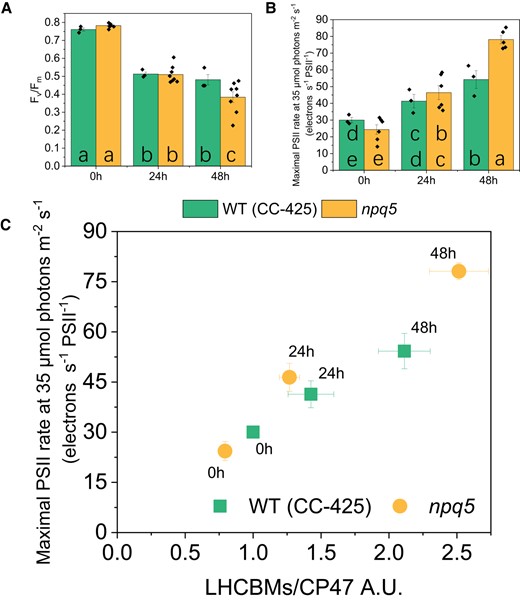
PSII's efficiency and antenna size. A)Fv/Fm; B) functional PSII antenna size; C) LHCBMs/CP47 protein ratio vs functional PSII antenna size. Densitometry data of LHCBMs and CP47 were each normalized in WT (CC-425) at 0 h before calculating the ratio in C). Data shown are mean ± SEM, n = 3 or 8 (npq5 in A and B) biological replicates. The actinic light source peaks at 630 nm. Different lowercase letters between means denote a statistically significant difference (P < 0.05; see the Materials and ethods section for details) in A) and B).
Since a change in antenna size is a well-known response to HL exposure (Neale and Melis 1986; Croce 2020), the functional antenna size of PSII was measured. Before HL treatment (i.e. in LL), the functional antenna size of PSII was around 20% smaller in npq5 than in WT (CC-425) (Fig. 2B). This value matches the immunoblot data (Fig. 1B), indicating that the absence of LHCBM1 does not influence the functional organization of the other antennae. Upon HL exposure, the functional PSII antenna size increased in both strains and, after 48 h HL, was significantly higher in npq5 than in WT (CC-425) (Fig. 2B). Immunoblots against all LHCBM types showed that the relative amount of each subtype remained unchanged in WT (CC-425) and npq5 during HL exposure (Supplemental Fig. S1), indicating that there is no specific increase of any of the subunits. Thus, the increase in antenna size upon HL exposure is likely due to a loss of functional core complexes (as substantiated by the lower Fv/Fm), which results in a larger antenna for the remaining functional ones due to the energetic connectivity (Joliot 1964).
The PSII functional antenna size (Fig. 2C, Supplemental Fig. S2) qualitatively correlates with the LHCBMs/CP47 protein ratio in both strains after 24 h of HL, while there is some deviation upon 48 h. These results suggest that, while upon 24 h HL, most antenna proteins are functionally connected to the core; after longer HL exposure, part of the antenna is no longer well connected to the photochemical trap.
Electron transport is impaired in HL in npq5
Next, we checked possible differences between WT and mutant in the components and functionality of the electron transport chain. To calculate the PSI/PSII ratio, we quantified the proteins using immunoblots and the charge separation by measuring the electrochromic shift (ECS). npq5 cells grown in LL showed a slightly lower PSI/PSII ratio than the control strain (Fig. 3A). This agrees with previous results, which demonstrated that a decrease in PSII antenna size is compensated by a change in PSI/PSII ratio (Nicol et al. 2019; Xu et al. 2020). The agreement between the functional and the protein measurements indicates that all photosystems present in the membrane work properly. The functional data showed a significant increase in the PSI/PSII ratio during HL treatment, especially in npq5 after 48 h HL (Fig. 3A). On the contrary, at the protein level, a decrease in PSI/PSII ratio was observed (Fig. 3B). These results suggest that part of PSII is not functional in HL and that this population is larger in npq5 than in the WT (CC-425).
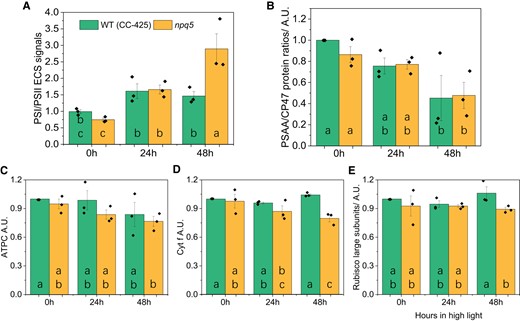
Protein composition of the photosynthetic apparatus and PSI/PSII ratios during HL acclimation. The functional measurement of PSI/PSII ratio are based on the ECS signal A), PSAA/CP47 protein ratio B), the amount of ATP gamma subunit (ATPC) C), cytochrome f (Cyt. f) D), and Rubisco large subunits E) are shown. Data shown were normalized to WT (CC-425) at 0 h in B–E and are mean ± SEM, n = 3 biological replicates with each of one technical replicate in B–E and four technical replicates in A. Different lowercase letters between means denote a statistically significant difference (P < 0.05; see the Materials and methods section for details).
To check if the electron transport rate of PSII is affected in npq5, we measured the PSII turnover rate in continuous light during HL treatment by multiplying the Y(II) parameter by the cross-section of PSII at a given light intensity (Lazar 1999; Nawrocki et al. 2019) (Fig. 4, Supplemental Fig. S3). The results show that the turnover rate (per PSII) in npq5 is lower than in WT (CC-425) in all light conditions and that it further decreases upon 48 h HL. This is consistent with partial PSII photoinhibition in the mutant and shows that the ETR is limited by factors other than the absorption cross-section, which increased under HL treatment (Fig. 2).
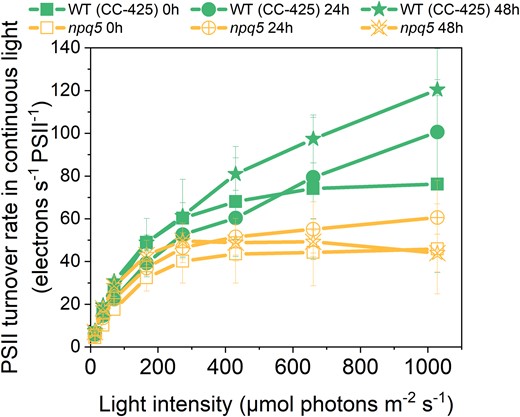
PSII turnover rate in continuous light. PSII turnover rate was measured by multiplying Y(II) and maximal PSII rate at given light intensities (x axis). Data shown are mean ± SEM, n = 3 biological replicates. See the Materials and methods section for details about the calculation.
We also compared the abundance of the other major photosynthetic complexes in npq5 and CC-425, using antibodies against ATP gamma subunit (ATPC), cytochrome f (Cyt. f), and Rubisco large subunit (Rubisco L). ATPC, Cyt. f, and Rubisco L showed only a slight difference between npq5 and WT (CC-425) in both LL and HL (Fig. 3C to E, Supplemental Fig. S2).
Photoprotection capacity after high-light acclimation
Next, we examined the capacity of NPQ in the two strains. Since the amount of LHCSRs in the cells was shown to qualitatively correlate with the level of NPQ (Bonente et al. 2012; Perozeni et al. 2018; Tian et al. 2019), we also quantified the LHCSR proteins during HL treatment.
Maximal NPQ was similarly low in both strains in LL and developed upon HL exposure only in WT (CC-425) (Fig. 5A). In npq5, the NPQ development was hampered, although there was a small increase after 48 h in HL. Interestingly, LHCSR3 was expressed in both strains in HL: the LHCSR3/CP47 ratio was similar in WT (CC-425) and npq5 at 24 h HL and increased further after 48 h HL but was less in npq5 than in the control (Fig. 5B). The LHCSR1/CP47 ratio was far lower in npq5 than in WT (CC-425) at all time points (Fig. 5C). However, in HL-exposed cells, LHCSR1 is present in very low amounts compared to LHCSR3 (Peers et al. 2009; Tian et al. 2019). To check how much the absence of LHCSR1 can affect NPQ, we examined the lhcsr1 mutant (Allorent et al. 2016) and its control strain (WT 4A+) under the same light conditions. The absence of LHCSR1 had little effect on the NPQ level (Supplemental Fig. S4), and it is thus unlikely to be the reason for the lower NPQ in npq5.
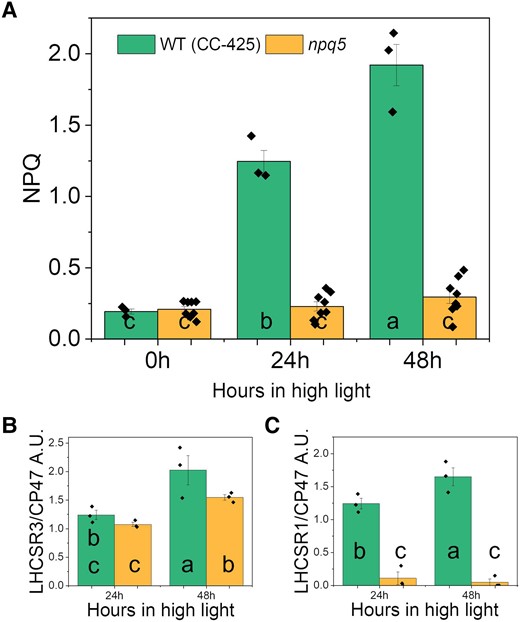
NPQ parameters in HL. Maximum value for NPQ A), LHCSR3/CP47 B), and LHCSR1/CP47 C) during HL treatment. Data shown are mean ± SEM, n = 3 or 8 (npq5 in A) biological replicates. Densitometry data of each protein were normalized in WT (CC-425) at 24 h (LHCSRs) or 0 h (CP47) before calculating the ratio in B–C. Different lowercase letters between means denote a statistically significant difference (P < 0.05; see the Materials and methods section for details).
Is lumen acidification the bottleneck of NPQ development in npq5 in vivo?
NPQ is regulated not only by the amount of LHCSR3 but also by the extent of lumen acidification. The lower PSII turnover rate in the mutant than in the control strain suggests a reduced capacity to create a proton gradient across the thylakoid membrane under light, which can be a reason for the low NPQ. To check this hypothesis, we bypassed the light-induced proton translocation to the lumen by adding acid to the cells. As shown previously, the addition of acetic acid to the cells induces stable, light-independent NPQ due to the LHCSR3 protonation (Tian et al. 2019). The quenching is reversible upon neutralization of the culture. We adopted this method to measure the maximal NPQ capacity at pH 5.5 in WT (CC-425) and npq5. In npq5, the quenching was three times larger upon acid induction than under light (Fig. 6A, Supplemental Fig. S5A). However, the value at pH 5.5 was still far lower than that of the control strain at the same pH. To test if the cells reached the maximum NPQ capacity, we added a double volume of acetic acid. No further increase in NPQ was observed (Supplemental Fig. S6). As shown by the scatterplot in Fig. 6B, the acid-induced NPQ maximum qualitatively correlates with the amount of LHCSR3 per PSII (LHCSR3/CP47) in both strains but is always lower in npq5 than in CC-425. As an additional control, we also measured acid-induced NPQ in the npq4 mutant, which does not contain LHCSR3 but contains a WT level of LHCBM1 (Supplemental Fig. S5B). At variance with npq5, the NPQ value remained very low in npq4 also upon acidification of the lumen, in agreement with the role of LHCSR3 as pH sensor and NPQ effector.
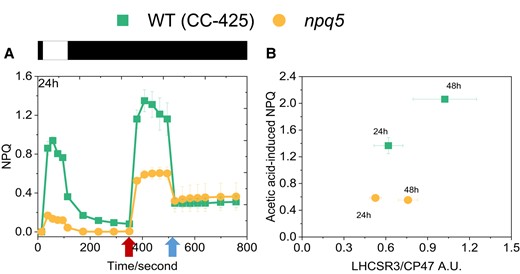
Light- and acid-induced NPQ. Comparison of light- and acid-induced NPQ in npq5 and WT (CC-425) A) and correlation between the LHCSR3 content per core in the cells (expressed as LHCSR3/CP47 ratio) and acid-induced NPQ B). The additions of 1 M acetic acid (decreases pH to 5.5) and 2 M KOH (neutralizes pH to 7.0) in A) are indicated by the first and the second arrows, respectively. Illumination (1,500 μmol photons m−2 s−1) and dark phases are indicated by white and black bars, respectively. Data shown are mean ± SEM, n = 3 (CC-425); n = 5 (npq5) biological replicates.
Discussion
The main player in NPQ in C. reinhardtii is LHCSR3 (Peers et al. 2009). This complex was shown to contain pigments (Bonente et al. 2011) and to switch from a light-harvesting to a quenched conformation in response to the protonation of its luminal residues (Liguori et al. 2013). LHCSR3 has thus been proposed to be both the pH sensor and the quencher in the membrane of C. reinhardtii (Tian et al. 2019). The absence of LHCSR3 in the cells (npq4 mutant) completely abolishes the capacity of NPQ (Peers et al. 2009), at least in the absence of UV light (Allorent et al. 2016). A similar effect on NPQ was observed in the npq5 mutant, which lacks LHCBM1 (Elrad et al. 2002), suggesting that this subunit is also involved in quenching. However, differently from LHCSR3 and LHCSR1, LHCBM1 is not a pH sensor and cannot switch from a light-harvesting to a quenched conformation in response to pH changes, as shown by both in vitro and in vivo experiments (Natali and Croce 2015; Dinc et al. 2016) and confirmed here by the analysis of the npq4 mutant. What is, thus, the reason for the substantial reduction in NPQ in the npq5 mutant? The data shown in this work indicate that this effect is not due to a low amount of LHCSR3. It is also clear that although the electron transfer chain works less efficiently and the pH of the lumen is probably slightly higher in npq5 than in the reference strain, this difference is not sufficient to explain the large difference in NPQ: acid-induced NPQ is still far smaller in the mutant than in the WT. Since upon 24 h of HL (1) the amount of LHCSR3 is similar in npq5 and its reference WT, (2) PSII antenna size is similar in those strains, and (3) when induced with acid, the lumen pH is the same, we can assume that the number of quenching centers (i.e. quenched LHCSR3) is also identical. What is, thus, the role of LHCBM1 in NPQ? We propose that the absence of LHCBM1 influences the connectivity between the quencher and the supercomplexes. LHCBM1 was shown to be part of trimer S, connecting this trimer with CP43 (Sheng et al. 2019). However, we can exclude that the absence of LHCBM1 alters the functional connectivity between the antenna and the core because the maximal PSII rate in the mutant is very similar to that of the WT, qualitatively correlating with the LHCBM content. The value of Fv/Fm is also identical in mutant and WT, excluding differences in the presence of detached antenna complexes up to 24 h of HL. It is thus likely that the absence of LHCBM1 influences the association of LHCSR3 with the supercomplex, thus limiting the quencher efficiency. Indeed, structural and spectroscopic data suggest that LHCSR3 interacts with the LHCBMs (Semchonok et al. 2017; Tian et al. 2019) and, in particular, that LHCSR3 dimer is in direct contact with the LHCII-S trimer in the C2S2 PSII–LHCII supercomplex (Semchonok et al. 2017). A quenching rate of (150 ps)−1 was measured for C. reinhardtii WT cells in physiological conditions (Tian et al. 2019). This value is very similar to the overall trapping time in PSII supercomplexes (Caffarri et al. 2011), meaning that LHCSR3 (1) is well connected to PSII and (2) is an efficient quencher (see Croce and van Amerongen 2020 for details on energy flow in the photosystems). In principle, LHCBM1 can influence both aspects. The interaction between LHCBM1 and LHCSR3 can create a more efficient quencher, for example, by stabilizing the quenched conformation of LHCSR3. The absence of LHCBM1 might also alter the association of LHCSR3 with the supercomplex, either because LHCBM1 is directly involved in the LHCSR3 docking or because its absence leads to the reorganization of the supercomplex, affecting LHCSR3 binding. As a result, less excitation energy reaches the quencher, explaining the NPQ phenotype. In this respect, it is interesting to mention that recent in vitro experiments have shown that LHCBM1 has a high tendency to interact with other LHCBMs. This property was suggested to depend on its charged N-terminus, which can help in protein–protein interactions (Kim et al. 2020), supporting the hypothesis that LHCBM1 is involved in the docking of LHCSR3.
Materials and methods
Strains and growth conditions
The null C. reinhardtii LHCBM1 mutant npq5 (Elrad et al. 2002) (alternative name CC-4073, obtained from the Chlamydomonas Center, https://www.chlamycollection.org/) was used in this work. WT (CC-425), the parental strain used for insertional mutagenesis to generate npq5 (Niyogi et al. 1997), was used as the reference strain. npq4 (Peers et al. 2009), which lacks both lhcsr3.1 and lhcsr3.2 genes, is virtually unable to perform the fast energy-dependent quenching and was used as a negative control. lhcsr1 mutant (Allorent et al. 2016) and its control 4A+ were used to test the impact of LHCSR1 knock-out on NPQ. All the strains were grown in liquid TAP medium (Gorman and Levine 1965) (25 °C 140 rpm/min) at least 72 h in LL (less than 15 μmol photons m−2 s−1) to reach the exponential phase. One day before the HL exposure, the strains were diluted in HSM (Sueoka 1960) in LL. On the day of the experiment, the cells were harvested by centrifugation and resuspended in fresh HSM. All cells were adjusted to OD750 = 0.2 and incubated in LL for 1 h before HL (500 μmol photons m−2 s−1) treatment. Aliquots were collected at different time points: 0 h HL (1 h LL), 24 h HL, and 48 h HL. In the case of CC-425, 50 mg/L arginine was added when the cells were grown in TAP.
In vivo photosynthetic measurements
Nonphotochemical quenching
To quantify light-induced NPQ, a short 5.5-min protocol was used, in which the illumination phase was 2.5 min after a 10-s dark period (when F0 was acquired), and the subsequent recovery period (in darkness) was 3 min. NPQ was calculated as (Fm/Fm′)−1, and the first 50-s fluorescence signals (during illumination) were used for maximum NPQ calculation to avoid the possible superposition of state transitions that occur on longer timescales. Fv/Fm was calculated as (Fm−F0)/Fm (Baker 2008). Actinic light 1,500 μmol photons m−2 s−1, saturating pulses of >10,000 μmol photons m−2 s−1 and 180 ms pulse duration were used. All actinic light was red, peaking at 630 nm. Weak detecting light flashes were obtained by filtering broad white light LEDs with a 10-mm interference Schott filter [520 nm, 10 nm full-width-half-maximum (FWHM)].
Acid-induced NPQ was performed as previously reported (Tian et al. 2019). In brief, 1 M acetic acid was added to the culture to decrease the pH to 5.5, and 2 M KOH was used to set the pH to 7 (Tian et al. 2019). Acid-induced quenching (Fm−F0)/Fm was recorded in darkness using a DUAL-PAM 100 (Walz, Germany) with saturating pulses of 12,000 μmol photons m−2 s−1 and 180 ms duration. Cells were dark-adapted for at least 30 min before measurements. To measure light-induced quenching, a short actinic light period was also used (∼1,500 μmol photons m−2 s−1).
Maximal PSII rate
The rate of PSII under light-limiting conditions was measured to compare the changes in effective PSII cross-section upon acclimation to HL conditions. To this end, the fluorescence rise upon transition of dark-adapted cells (QA maximally oxidized) to light (QA maximally reduced) in the presence of 10 μM dichlorobenzyl dimethyl urea (DCMU) was measured using 630 nm, 35 μmol photons m−2 s−1 actinic light according to Lazar (1999) with minor modifications (Nawrocki et al. 2019; Tian et al. 2019). This method relies on the fact that under LL, the PSII rate is limited by the rate of absorption and, thus, linearly related to the PSII functional cross-section. Note that since PSII reaction centers (RCs) are not individual entities but are energetically connected, their antenna size depends on the extent of closed RCs nearby. The DCMU method integrates the fluorescence signal over the entire time course of ∼100% open- to 100% closed RCs, effectively providing the average rate between a situation where the neighboring RCs are closed and open.
PSII turnover rate in continuous light
The PSII turnover rate was measured with a JTS-10 spectrophotometer according to Lazar (1999) and Nawrocki et al. (2019) with minor modifications. PSII turnover rate was calculated as: [(PSII operating efficiency, Y(II)) × (the extrapolated maximal PSII rate)] at the same light intensity. Y(II) was measured via a light curve in a DUAL-PAM 100 at 13, 37, 70, 166, 273, 430, 660, and 1028 μmol photons m−2 s−1. The maximal PSII rate at 35 μmol photons m−2 s−1 was measured in a JTS-10 (see above). A linear fit forced through zero and the value at 35 μmol photons m−2 s−1 was then used to extrapolate the maximal rate to each intensity used in the PAM for Y(II) measurements. Cells were kept in darkness for at least 30 min before the experiments.
Electrochromic shift
The functional PSI/PSII RC ratio was measured using single-turnover laser flashes in vivo according to Joliot and Joliot (2005) and Alric et al. (2010) with the modifications described in Nawrocki et al. (2016).
Protein analysis
Total cell protein extracts and immunoblots were performed according to Ramundo et al. (2013) and Dinc et al. (2014). Five micrograms of total cell protein extracts were loaded on each well. All antibodies were purchased from Agrisera (Sweden): LHCBM5 (AS09 408), LHCSR1 (AS14 2819), LHCSR3 (AS14 2766), CP47 (AS04 038), PsaA (AS06 172), ATPC (AS08 312), Cyt. f (AS06 119), and Rubisco large subunit (AS03 037).
Statistical analysis
Statistical analysis was performed using Data Processing System (DPS) with a two-way ANOVA test using Fisher's least significant difference (LSD) test to calculate significance (P < 0.05) (DPS software, Sinyosoft, version 9.50, http://www.dpsw.cn/dps_eng/index.html; (Tang and Zhang 2013). Lowercase letters are used to describe multiple comparisons after the LSD test (P < 0.05). Letters starting from “a” indicate the largest mean in the group of data, while “b” indicates a statistically significant group with a smaller mean compared to “a”. Shared lowercase letters indicate the absence of a statistically significant difference (P < 0.05) between two datasets.
Accession numbers
Sequence data from this article can be found in the GenBank/EMBL data libraries under the following accession numbers: LHCSR3, P0DO18, and P0DO19; LHCBM1, AAM18057.
Acknowledgments
We thank Chen Hu for helping with the analyses.
Author contributions
X.L. did all the experimental work, analyzed the data, made the figures and wrote the first version of the results. W.J.N. helped in designing the experiments, analyzing the results, and writing the manuscript. R.C. conceived and supervised the project and wrote the final version of the manuscript with help from all authors.
Supplemental data
Supplemental Figure S1. Quantification of LHCBM subtypes based on immunoblotting during HL treatment in WT (CC-425) and npq5.
Supplemental Figure S2. Immunoblots of photosynthetic proteins during HL in WT (CC-124 and CC-425) and npq5.
Supplemental Figure S3. Photosystem II efficiency Y(II) measured at different light intensities.
Supplemental Figure S4. Maximum NPQ in WT (4A+) and lhcsr1 during HL treatment.
Supplemental Figure S5. Nonphotochemical quenching measurements.
Supplemental Figure S6. NPQ induction with acid.
Funding
This work is partially supported by the Human Frontier Science Program (HRSP) grant RGP0005/2021. X.L. was supported by the Chinese Scholarship Council 201606910042.
Dive Curated Terms
The following phenotypic, genotypic, and functional terms are of significance to the work described in this paper:
References
Author notes
The author responsible for distribution of materials integral to the findings presented in this article in accordance with the policy described in the Instructions for Authors (https://dbpia.nl.go.kr/plphys/pages/General-Instructions) is Roberta Croce ([email protected]).
Conflict of interest statement. None declared.