-
PDF
- Split View
-
Views
-
Cite
Cite
Xingang Zhou, Huan Gao, Xianhong Zhang, Muhammad Khashi u Rahman, Stefano Mazzoleni, Minmin Du, Fengzhi Wu, Plant extracellular self-DNA inhibits growth and induces immunity via the jasmonate signaling pathway, Plant Physiology, Volume 192, Issue 3, July 2023, Pages 2475–2491, https://doi.org/10.1093/plphys/kiad195
- Share Icon Share
Abstract
Plants have evolved sophisticated mechanisms to detect various forms of danger. Damage-associated molecular patterns (DAMPs) are endogenous danger molecules that are released from damaged cells and activate the innate immunity. Recent evidence suggests that plant extracellular self-DNA (esDNA) can serve as a DAMP molecule. However, the mechanisms by which esDNA functions are largely unknown. In this study, we confirmed that esDNA inhibits root growth and triggers reactive oxygen species (ROS) production in a concentration- and species-specific manner in Arabidopsis (Arabidopsis thaliana) and tomato (Solanum lycopersicum L.). Furthermore, by combining RNA sequencing, hormone measurement, and genetic analysis, we found that esDNA-mediated growth inhibition and ROS production are achieved through the jasmonic acid (JA) signaling pathway. Specifically, esDNA induces JA production and the expression of JA-responsive genes. The esDNA-mediated growth inhibition, ROS production, and gene expression are impaired in the JA-related mutants. Finally, we found that the JA signaling pathway is required for the esDNA-elicited resistance against the pathogens Botrytis cinerea and Pseudomonas syringae pv. tomato DC3000. This finding highlights the importance of JA signaling in esDNA-mediated biological effects, thereby providing insight into how esDNA functions as a DAMP.
Introduction
Plants encounter attacks from various assailants such as herbivorous insects and pathogenic microbes throughout their life cycle. When under such attacks, plants need an early recognition of the invader to detect the danger so they can respond accordingly. Plants, therefore, have evolved sophisticated strategies to perceive exogenous and endogenous danger signals (Ronald and Beutler 2010; DeFalco and Zipfel 2021). Exogenous signaling molecules, called pathogen-associated molecular patterns and herbivore-associated molecular patterns released by pathogens and herbivores, respectively, activate the “nonself recognition” pathway (Choi and Klessig 2016; Gust et al. 2017; Wang et al. 2022). In contrast, the perception of host-derived endogenous molecules called damage-associated molecular patterns (DAMPs) signals the “damaged-self recognition” pathway (Duran-Flores and Heil 2016; Gust et al. 2017; DeFalco and Zipfel 2021). Examples of molecules that serve as DAMPs include cell wall fragments, cutin monomers, oligogalacturonides, oligosaccharides, adenosine 5′-triphosphate, methanol, ethanol, and systemin (Akira et al. 2006; Choi et al. 2014; Gust et al. 2017). Plants perceive most of these molecules via cell surface proteins called pattern recognition receptors. The recognition induces early immunity responses, including calcium fluxes across the plasma membrane, the production of reactive oxygen species (ROS), and the activation of mitogen-activated protein kinases, thus leading to rapid defense gene expression (Seybold et al. 2014; DeFalco and Zipfel 2021).
DNA normally exists in the living cell as genetic material, but it can be released either actively or passively from damaged or infected cells to the extracellular space. After release, these extracellular DNA (eDNA) can be degraded into fragments in a variable size range (Kuriyama and Fukuda 2002; Wu et al. 2013; De Lorenzo et al. 2018). Previously, we found that fragmented extracellular self-DNA (esDNA) (i.e. DNA originating from conspecifics) had concentration-dependent, species-specific inhibitory effects on plant growth, which provided an explanation for negative plant–soil feedbacks, i.e. a plant alters soil conditions in ways that negatively affect that plant's performance (Mazzoleni et al. 2015a, 2015b; Bonanomi et al. 2022). Moreover, there now is experimental evidence supporting esDNA as a DAMP that can cause the species specificity in plant damaged-self recognition (Barbero et al. 2016; Duran-Flores and Heil 2018). For example, exogenous esDNA can trigger the generation of ROS, elevation of cytoplasmic calcium, membrane depolarization, and the activation of the mitogen-activated protein kinase signaling cascades in plants (Barbero et al. 2016; Duran-Flores and Heil 2018; Vega-Muñoz et al. 2018; Ferrusquia-Jimenéz et al. 2022). Moreover, the application of esDNA can elicit the expression of defense genes and induce resistance against pathogens and herbivores in plants, such as Arabidopsis (Arabidopsis thaliana), chili pepper (Capsicum annuum L.), lettuce (Lactuca sativa L.), and common bean (Phaseolus vulgaris L.) (Duran-Flores and Heil 2018; Vega-Muñoz et al. 2018; Rassizadeh et al. 2021; Ferrusquia-Jimenéz et al. 2022). Although the study of plant esDNA acting as a DAMP molecule has gained much attention, the mechanisms by which esDNA activates plant immunity are largely unknown.
Plant immune responses to microbial pathogens and insect herbivores are regulated by phytohormones, such as jasmonic acid (JA), abscisic acid (ABA), ethylene (ET), and salicylic acid (SA) (Erb et al. 2012; Pieterse et al. 2012). Particularly, JA can activate plant resistance against necrotrophic pathogens and chewing insects by master transcription factor–mediated transcriptional reprogramming (Glazebrook 2005; Ballare 2011). JA has also been demonstrated to regulate various aspects of plant development and growth, including inhibiting root growth (Chen et al. 2011). Decades of studies have identified a core JA signaling module consisting of the following: an F-box protein CORONATIN INSENSITIVE1 (COI1), which is the receptor of jasmonoyl-isoleucine (JA-Ile), the main active JA derivative (Devoto et al. 2002; Xu et al. 2002); a group of jasmonate-ZIM domain (JAZ) proteins that are the coreceptors and function as transcriptional repressors (Chini et al. 2007; Thines et al. 2007); and the basic helix–loop–helix (bHLH) transcription factor MYELOCYTOMATOSIS ONCOGENE HOMOLOG 2 (MYC2), which is the master transcription factor that regulates most aspects of JA responses (Lorenzo et al. 2004; Dombrecht et al. 2007). In the steady state, JAZ proteins interact with and repress the transcriptional activity of MYC2. In response to internal or external stimuli, elevated JA-Ile levels promote COI1-dependent degradation of JAZ repressors, thereby activating (derepressing) MYC2-directed transcription of JA-responsive genes (Thines et al. 2007; Kazan and Manners 2013; Zhai et al. 2013; Du et al. 2017).
Accumulating evidence showed that JA and the wounding signals share, at least in part, a common pathway, highlighting the critical role of JA signaling in damage-related defense responses (Lorenzo et al. 2004; Howe and Jander 2008; Pieterse et al. 2012; Mousavi et al. 2013). To investigate the mechanism by which esDNA functions as a DAMP, in this study, we confirmed that esDNA can inhibit root growth and trigger ROS production in a concentration- and species-specific manner in Arabidopsis and tomato (Solanum lycopersicum L.). Furthermore, by combining RNA-sequencing, hormone measurement, and genetic analysis, we found that esDNA-mediated growth inhibition and immunity induction are achieved depending on the JA signaling pathway.
Results
esDNA inhibits root growth and induces ROS production
To investigate which plant signaling pathway is involved in esDNA-mediated growth inhibition and defense activation, we first confirmed the effects of esDNA on root growth under our experimental conditions. Five-day-old Arabidopsis Col-0 seedlings or germinated tomato cv. Dongnong (DN) seeds were transferred to liquid half-strength Murashige and Skoog (MS) medium supplemented with different concentrations of fragmented esDNA (Supplemental Fig. S1A) for the growth inhibition assays. Consistent with previous reports (Mazzoleni et al. 2015a, 2015b; Duran-Flores and Heil 2018), the esDNA showed inhibitory effects on primary root growth in a concentration-dependent manner (Fig. 1, A and B). esDNA at 20 to 500 μg mL−1 inhibited the primary root growth of Arabidopsis and tomato, while esDNA at 0.02 to 2 μg mL−1 did not generate obvious inhibitory effect. Because both 200 and 500 μg mL−1 of esDNA showed the strongest inhibition, we used the concentration of 200 μg mL−1 for further analysis.
![esDNA inhibits primary root growth and increases H2O2 content in leaves. Effects of different concentrations of esDNA on primary root length of Arabidopsis Col-0 A) and tomato cv. DN B). Effects of esDNA and nonself eDNA on primary root length and leaf H2O2 content of Arabidopsis Col-0 (C, E, respectively) and tomato cv. DN (D, F, respectively). For Arabidopsis Col-0, nonself eDNA of Arabidopsis Wassilewskija, A. pumila, canola, tomato, and wheat were used. For tomato cv. DN, nonself eDNA of tomato cv. QiYan (QY) and Hongshengnv (HS), eggplant, Arabidopsis Col-0, and wheat were used. For C to F), both esDNA and nonself eDNA were applied at 200 μg mL−1. For E) and F), the photos show H2O2 content in leaves visualized by diaminobenzidine staining. The bar plots show H2O2 content as determined by the titanium sulfate spectrophotometric method. FW, fresh weight. Data are expressed as means ± Sd [n = 18 for A) and C); n = 30 for B) and D); and n = 9 for E) and F)]. Different letters indicate significant difference between treatments (Tukey's HSD test, P < 0.05). The scale bars represent 8 mm.](https://oup.silverchair-cdn.com/oup/backfile/Content_public/Journal/plphys/192/3/10.1093_plphys_kiad195/1/m_kiad195f1.jpeg?Expires=1747857527&Signature=uSJXW5vbPre0Ajee3PgGzGtBOtv9cFvKRBYD7Tsi1bKf-QLHtf~fyhKtOAjZsBzp7iPHkP7LLCJHCOl6mj78e7the6Knb0iZBbxp7okZLjIM2pGUfc06KmXElVZSeWyk9RO1-zGEdys3vtMkdBAmd9~WTAKSAucnve1nvaGmyPJrmqw7SC4xyWd2ApM3~rZB8RIMHCGTrhYvD69WSF9T6h3Qyt3rR~Afvp7X1pKxqkEaO2CLK2FqOIXwgpjqU11M2rVJXuEV01ZdA-KmDSP86jorRO86Ma6DiaKW5SE~BjtEHenQzSXwX9MHNg3y80SKUp~hGY6VaPqdU54f4-5Ykw__&Key-Pair-Id=APKAIE5G5CRDK6RD3PGA)
esDNA inhibits primary root growth and increases H2O2 content in leaves. Effects of different concentrations of esDNA on primary root length of Arabidopsis Col-0 A) and tomato cv. DN B). Effects of esDNA and nonself eDNA on primary root length and leaf H2O2 content of Arabidopsis Col-0 (C, E, respectively) and tomato cv. DN (D, F, respectively). For Arabidopsis Col-0, nonself eDNA of Arabidopsis Wassilewskija, A. pumila, canola, tomato, and wheat were used. For tomato cv. DN, nonself eDNA of tomato cv. QiYan (QY) and Hongshengnv (HS), eggplant, Arabidopsis Col-0, and wheat were used. For C to F), both esDNA and nonself eDNA were applied at 200 μg mL−1. For E) and F), the photos show H2O2 content in leaves visualized by diaminobenzidine staining. The bar plots show H2O2 content as determined by the titanium sulfate spectrophotometric method. FW, fresh weight. Data are expressed as means ± Sd [n = 18 for A) and C); n = 30 for B) and D); and n = 9 for E) and F)]. Different letters indicate significant difference between treatments (Tukey's HSD test, P < 0.05). The scale bars represent 8 mm.
The observed inhibitory effects of fragmented eDNA showed taxonomic specificity. For the growth inhibition of Arabidopsis Col-0, eDNAs from 2 Arabidopsis ecotypes (Col-0 and Wassilewskija), and a close relative from the same genus (Arabidopsis pumila) inhibited primary root growth most strongly (by 60.74%, 59.46%, and 54.84%, respectively; Figs. 1C and S1B). eDNA from canola (Brassica napus L.), a relative from the same family: Brassica, caused a weaker but still significant inhibitory effect (36.36%) [P < 0.05, Tukey's honest significant difference (HSD) test]. However, eDNA from tomato and wheat (Triticum aestivum L.), plant species from different families, did not significantly affect the growth of the primary root. For the growth inhibition of tomato cv. DN, eDNAs from 2 cultivated tomato cultivars (cv. DN and QiYan) had the strongest inhibitory effects (62.20% and 60.67%, respectively) (Fig. 1D). eDNA from Hongshengnv, a cherry tomato (S. lycopersicum var. cerasiforme) cultivar, which is believed to be the evolutionary intermediate between the wild and cultivated tomato (Lin et al. 2014), showed a moderate inhibitory effect (46.54%). eDNA from eggplant (Solanum melongena L.) showed a relatively weaker inhibitory effect (30.89%), while no inhibitory effect was observed for the eDNAs from 2 phylogenetically distant species Arabidopsis and wheat (Figs. 1D and S1B). A very similar pattern was observed in eDNA-induced ROS production in Arabidopsis Col-0 and tomato cv. DN leaves, suggesting that eDNA-mediated biological effects (i.e. growth inhibition and ROS production) are species specific, as the effects of esDNA are related to the taxonomic distance between the source and receiver (Fig. 1, E and F).
We further confirmed that the observed biological effects of esDNA were attributed to DNA fragments but not RNA or protein impurities. Treating the esDNA preparation with DNAse abolished its inducing role in H2O2 production (Supplemental Fig. S2A), whereas treatments with RNAse or proteinase did not affect the esDNA-induced H2O2 production (Supplemental Fig. S2A). Moreover, nonfragmented esDNA was not able to induce H2O2 production (Supplemental Fig. S2B). Together, we confirmed that the fragmented esDNA can inhibit root growth and elicit H2O2 production in a concentration- and species-dependent manner.
Transcriptome profiling of esDNA-regulated genes in Arabidopsis
To understand how esDNA inhibits plant growth and triggers ROS production, we performed RNA-seq experiments with 4 biological replications to identify genes regulated by esDNA in Arabidopsis. First, we compared the global transcriptome profiles of Arabidopsis seedlings (Col-0) treated with water (mock), esDNA (Col-0), and nonself eDNA (tomato cv. DN) on 60 min after treatment. Principal component analyses revealed different gene expression patterns among these 3 treatments (Supplemental Fig. S3A). Compared with the mock, the esDNA up- and downregulated 1,807 and 1,697 genes, respectively, while the nonself eDNA up- and downregulated 504 and 222 genes, respectively [log2 fold change (log2FC) > 1 and false discovery rate (FDR)-adjusted P < 0.01]. Since tomato eDNA did not inhibit root growth and elicit H2O2 production in Col-0 (Fig. 1, C and E), we think that tomato eDNA serves as a better control than mock and defined genes differentially expressed between the esDNA and nonself eDNA treatments as genes specifically regulated by esDNA. A total of 3,343 genes were differentially expressed between the esDNA and nonself eDNA treatments (log2FC > 1 and FDR-adjusted P < 0.01) (Supplemental Data Set 1). Among these 3,343 genes, 1,822 were upregulated by esDNA (i.e. higher expression level in esDNA-treated plants vs. nonself eDNA–treated plants and FDR-adjusted P < 0.01), and 1,521 were downregulated by esDNA (i.e. lower expression level in esDNA-treated plants vs. nonself eDNA–treated plants and FDR-adjusted P < 0.01). Gene Ontology (GO) analysis indicated that esDNA-regulated genes were mainly enriched in pathways related to responses to ROS, chitin, JA, wounding, and oxidative stress (Fig. 2A). This analysis is consistent with the observation that esDNA can induce ROS production.

esDNA modulates the JA-dependent signaling pathway in Arabidopsis. A) GO term enrichment analysis of DEGs as affected by esDNA. The top 10 GO terms in each of the 3 GO categories were shown. B) Venn diagrams showing the number of shared and unique DEGs between different treatments. C) Heat map showing the expression pattern of the 1,130 DEGs coregulated by both esDNA and MeJA among different treatments. D) Heat map showing the expression pattern of the 1,070 DEGs coregulated by wounding and MeJA among different treatments. E) Heat map showing the expression pattern of genes involved in the JA biosynthesis, signaling, and responses in the esDNA and different hormone treatments. For heat maps, the color scale indicates the log2FC values of DEGs. Values greater than 0 indicate upregulated, and values less than 0 indicate downregulated. esDNA, fragmented DNA of Arabidopsis Col-0; Wounding, mechanical damage treatment; MeJA, methyl jasmonate; SA, salicylic acid; ACC, 1-aminocyclopropane-1-carboxylic acid; ABA, abscisic acid.
The finding that esDNA-regulated genes in Arabidopsis are enriched in the JA/wounding signaling pathway suggests that defensive phytohormones may be involved in esDNA-mediated biological processes. We therefore compared the esDNA-regulated genes with differentially expressed genes (DEGs) affected by some defense-related phytohormones or treatments, including methyl jasmonate (MeJA) (50 μ M), ABA (50 μ M), 1-aminocyclopropane-1-carboxylic acid (ACC, 10 μ M), and SA (0.5 mM), and mechanical wounding. Phytohormones were added into the growth medium. Dimethyl sulfoxide solution served as the control for ACC, ABA, and SA treatments, while ethanol served as the control for the MeJA treatment. For the wounding treatment, Arabidopsis Col-0 seedlings were consecutively wounded 3 times using hemostatic forceps, while nonwounded seedlings served as the control. On 60 min after treatment, about 33.80% (1,130 of 3,343) and 34.34% (1,148 of 3,343) of esDNA-regulated genes were also regulated by MeJA and mechanical wounding treatments, respectively (Fig. 2B). Moreover, about 32.38% (590 of 1,822) and 22.45% (409 of 1,822) of genes upregulated by esDNA were upregulated, and 32.38% (117 of 1,521) and 22.45% (231 of 1,521) of genes downregulated by esDNA were downregulated by MeJA and mechanical wounding treatments, respectively (Supplemental Fig. S3B). By contrast, the proportion of esDNA-regulated genes that were regulated by ABA, ACC, and SA was lower (14.48%, 6.46%, and 25.64%, respectively) (Fig. 2B). These results suggest that the JA/wounding signaling pathway is involved in esDNA-mediated biological processes. We further analyzed the expression pattern of the 1,130 MeJA and esDNA-coregulated genes under different treatments and noticed that most of the esDNA-upregulated genes (85.76%) were upregulated by MeJA (Fig. 2C), suggesting that esDNA mainly functions to modulate JA-induced genes. Interestingly, we also observed a very similar regulatory pattern by analyzing the MeJA and wounding-coregulated genes, that is, 81.91% of the wounding-induced genes were upregulated by MeJA (Fig. 2D). Because it is well demonstrated that wounding and JA share, at least partially, a common pathway (Pieterse et al. 2012), it is reasonable to hypothesize that esDNA achieves its biological effects by regulating JA-induced gene expression. Indeed, our RNA-seq data revealed that esDNA can activate many well-characterized JA-induced genes in Arabidopsis, including those involved in JA biosynthesis (e.g. FAD3, LOX3, LOX4, and AOC3), signal transduction (e.g. MYC2 and 9 JAZs), and responses (e.g. VSP1, VSP2.1, ERF1, and RBOHF; Fig. 2E).
esDNA induces JA production and the expression of JA-responsive genes
To further investigate how esDNA modulates the JA signaling pathway, we first performed RT-qPCR analysis to test the esDNA-induced expression pattern of 3 well-known JA-induced genes LOX3, MYC2, and JAZ1 in Arabidopsis. LOX3 encodes a lipoxygenase involved in JA biosynthesis, and MYC2 encodes the master transcription factor of the JA signaling, while JAZ1 is a coreceptor of JA and acts to repress transcription of JA-responsive genes (Lorenzo et al. 2004; Dombrecht et al. 2007; Thines et al. 2007). Results showed that transcription levels of these 3 genes could be significantly induced by esDNA treatment within 2 h (Fig. 3A), suggesting the esDNA activates the JA signaling pathway at the early stages.
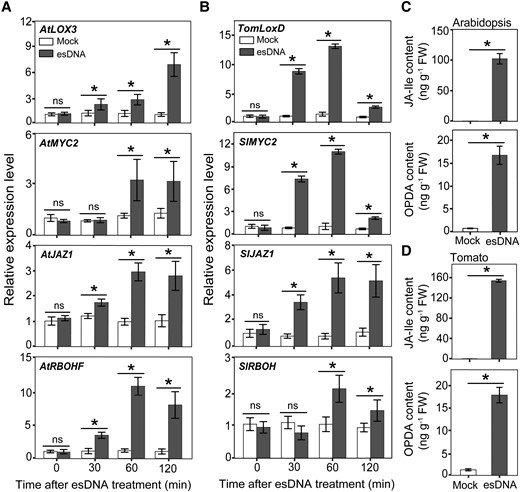
esDNA induces JA production and the expression of JA-responsive genes. Effects of esDNA on the expression of JA biosynthesis, signaling, and responsive genes in Arabidopsis Col-0 A) and tomato cv. DN B). OPDA and JA-Ile contents in Arabidopsis Col-0 C) and tomato cv. DN D) as affected by esDNA. esDNA was applied at 200 μg mL−1. FW, fresh weight. Data are expressed as means ± Sd (n = 3). Asterisks denote significant difference (Student's t test, P < 0.05).
We also examined the esDNA-affected expression pattern of RBOHF, which encodes a plasma membrane–localized NADPH oxidase contributing to ROS production in response to several stress signals, including JA (Suzuki et al. 2011). esDNA was found to induce the expression of RBOHF within 2 h (Fig. 3A), raising a possibility that esDNA-induced ROS production is achieved, at least partially, by transcription activation of RBOHF. These results were further confirmed by analyzing the expression patterns of 4 JA-induced genes in tomato (i.e. TomLoxD, SlMYC2, SlJAZ1, and SlRBOH; Fig. 3B).
Since esDNA activates the JA signaling pathway at the early stages, we tested the possibility that esDNA treatments can increase the JA content. We treated 10-d-old Arabidopsis Col-0 seedlings and 7-d-old tomato cv. DN seedlings with 200 μg mL−1 esDNA for 60 min and measured the content of the main active JA derivative JA-Ile and its biosynthetic precursor 12-oxophytodienoic acid (OPDA). esDNA treatments significantly and rapidly induced the production of JA-Ile and OPDA, both in Arabidopsis and tomato (Fig. 3, C and D). These results, together with the finding that esDNA induces the expression of several JA biosynthetic genes (Figs. 2E and 3, A and B), suggest that esDNA activates the JA signaling pathway by triggering JA biosynthesis.
JA is required for esDNA-mediated growth inhibition and ROS production
We next used a genetic strategy to corroborate the involvement of the JA signaling pathway in esDNA-mediated growth inhibition and ROS production by comparing the wild-type and several Arabidopsis mutants that are defective in hormone biosynthesis or signaling. These mutants include the JA-Ile biosynthesis mutant jar1-11, JA-insensitive mutant coi1-2, JA signaling mutant myc2-2, SA signaling mutant npr1-1, ethylene signaling mutant ein2-1, and ABA biosynthesis mutant aao3-4. We found that the esDNA-induced growth inhibition was attenuated in the 3 JA-related mutants (i.e. jar1-11, coi1-2, and myc2-2; 35.86%, 34.76%, and 30.18, respectively) when compared with the wild type (59.25%; Fig. 4A). By contrast, neither mutations in the SA and ET signaling nor in the ABA biosynthesis altered the inhibitory growth effect of esDNA. Of note, the mutation per se does not affect the ability of esDNA to inhibit root growth, as we found that eDNAs from the wild type and the mutants had comparable inhibitory effects (Supplemental Fig. S4). We also tested the effects of esDNA on the root growth of tomato JA receptor mutant (jai1) and the MYC2-RNAi transgenic plants, in which the expression of MYC2 was reduced by RNA interference (Du et al. 2017). Similarly, compared with their corresponding wild-type, esDNA-mediated growth inhibition was also impaired in the tomato jai1 mutant and the MYC2-RNAi plants (Fig. 4B). These results support that the JA signaling pathway is indeed involved in the esDNA-mediated growth inhibition.

esDNA-induced inhibition of root growth and changes in gene expression are attenuated in JA mutants. A) Effects of esDNA on primary root length of Arabidopsis Col-0 wild-type and JA, SA, ethylene, and ABA mutants. Arabidopsis mutants used were JA biosynthesis mutant jar1-11, JA-insensitive mutant coi1-2, JA signaling mutant myc2-2, SA signaling mutant npr1-1, ethylene signaling mutant ein2-1, and ABA biosynthesis mutant aao3-4. B) Effects of esDNA on primary root length of tomato wild-type and JA mutants. Tomato lines used were the JA-insensitive mutant jai1 and its wild-type line CM, and the JA signaling mutant MYC2-RNAi and its wild-type line M82. C) Effects of esDNA on the expression of genes involved in JA biosynthesis, signaling, and responses in Arabidopsis Col-0 wild-type and mutants. For A) and B), values above bars are the decreased percentage of primary root length in the esDNA treatment as compared with the mock. esDNA was applied at 200 μg mL−1. Data are expressed as means ± Sd (n = 18, 30, and 3 for A), B), and C), respectively). Different letters indicate significant difference between treatments (Tukey's HSD test, P < 0.05). Asterisks denote significant difference (Student's t test, P < 0.05).
To study the involvement of the JA signaling pathway in esDNA-mediated biological effects at the gene expression level, we performed RT-qPCR experiments to test the esDNA-induced gene expression in the abovementioned mutants. As expected, mutations in the JA biosynthesis and signaling, but not mutations in the SA and ET signaling nor in the ABA biosynthesis, showed remarkably lower esDNA-induced expression levels of LOX3, MYC2, JAZ1, and RBOHF in Arabidopsis Col-0 (Fig. 4C). Consistent with the reduced expression level of RBOHF in the JA-related mutants, we found that esDNA-induced ROS production was significantly attenuated in these JA-related mutants when compared with their wild-type plants (Supplemental Fig. S5). Previous studies have demonstrated that LOX3, MYC2, and JAZ1 are the transcriptional targets of the master transcription factor MYC2 (Lorenzo et al. 2004; Chini et al. 2007; Kazan and Manners 2013). By checking the published MYC2 chromatin immunoprecipitation (ChIP)-seq data (Wang et al. 2019), we noticed that about 63% (716 of 1,130) of esDNA and JA-coregulated genes can be bound by MYC2, including RBOHF (Supplemental Fig. S6). This analysis suggests that esDNA potentially modulates the changes in gene expression through MYC2-mediated transcriptional regulation. Together, these results provide convincing evidence to support that esDNA modulates growth inhibition and ROS production through the JA signaling pathway.
To examine the role of ROS production in the esDNA-mediated responses, we tested the effects of esDNA on the primary root growth and ROS production of Arabidopsis rbohD and rbohD mutants. We found that the esDNA-elicited primary root growth inhibition and ROS production were impaired in rbohD (17.00% and 13.14%, respectively) and rbohF (42.34% and 18.68%, respectively) when compared with the wild type (51.34% and 69.45%, respectively) (Supplemental Fig. S7, A and B). Therefore, enhanced ROS production is involved in the esDNA-mediated responses.
esDNA induces resistance to plant pathogens depending on the JA signaling pathway
Recent reports show that esDNA acts as a DAMP, which can be released by stressed cells undergoing necrosis, to trigger self-specific immunity induction (Duran-Flores and Heil 2018; Vega-Muñoz et al. 2018; Rassizadeh et al. 2021; Ferrusquia-Jimenéz et al. 2022). We therefore tested whether esDNA can elicit plant immunity against Botrytis cinerea, a necrotrophic pathogen that attacks more than 200 plant species (Van Kan et al. 2017). Leaves of 4-wk-old Arabidopsis and tomato seedlings were sprayed with 200 μg mL−1 esDNA for 24 h before systemic leaves (i.e. untreated leaves) were detached for B. cinerea infection. We found that, in wild-type plants, the B. cinerea–induced lesion area was significantly reduced by esDNA treatment (Fig. 5, A and B), suggesting that esDNA can enhance plant immunity against B. cinerea. We also performed the B. cinerea infection in the JA-related mutants (jar1-11 and coi1-2 of Arabidopsis and jai1 and MYC2-RNAi of tomato). When the leaves were not treated with esDNA, the lesion areas in the mutants were much bigger than those in the wild type, consistent with the previous finding that JA is required for plant immunity against B. cinerea (Diaz et al. 2002). However, when the mutant leaves were pretreated with esDNA, we did not detect any effect of esDNA on the disease symptoms caused by B. cinerea. These results suggest that the JA signaling pathway is required for esDNA-mediated immunity induction.
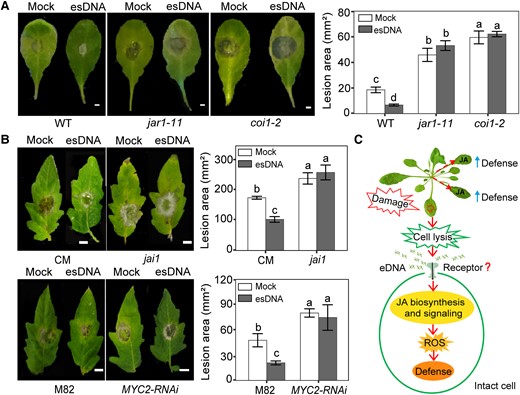
esDNA induces resistance to B. cinerea depending on the JA signaling pathway. A) Effects of esDNA on lesion area in Arabidopsis Col-0 wild-type and JA mutants. Scale bar = 2 mm. Arabidopsis mutants used were JA biosynthesis mutant jar1-11 and JA-insensitive mutant coi1-2. B) Effects of esDNA on lesion area in tomato wild-type and JA mutants. Scale bar = 8 mm. Tomato lines used were the JA-insensitive mutant jai1 and its wild-type line CM, and the JA signaling mutant MYC2-RNAi and its wild-type line M82. Leaves of 4-wk-old Arabidopsis or tomato (2 leaves per plant) were sprayed with 200 μg mL−1 of esDNA or water. After 24 h, systemic leaves (i.e. untreated leaves) were detached and inoculated with B. cinerea, and the lesion area was measured at 72 h after inoculation of B. cinerea. Data are expressed as means ± Sd (n = 15). Different letters indicate significant difference between treatments (Tukey's HSD test, P < 0.05). C) Putative mechanisms for esDNA-triggered immunity in plants. DNA fragments are released into the extracellular space upon cell damage. Then, esDNA may be perceived by unknown pattern recognition receptors in intact cell surrounding of the damaged cells. The perception of esDNA promotes the biosynthesis and signaling of JA, the formation of ROS, which finally modulates the systemic defense response of the plants.
The activation of the JA signaling pathway may repress the SA signaling, which can activate defense against biotrophic pathogens (Glazebrook 2005; Ballare 2011). Then, we determined the effects of esDNA on the immunity of Arabidopsis against the biotrophic pathogen Pseudomonas syringae pv. tomato (Pst) DC3000. Leaves of 4-wk-old Arabidopsis seedlings were sprayed with 200 μg mL−1 esDNA for 24 h, and the systemic leaves were then sprayed with a solution of PstDC3000. Consistent with previous studies (Duran-Flores and Heil 2018; Rassizadeh et al. 2021), esDNA enhanced the immunity against PstDC3000 in the wild-type Arabidopsis plants (Supplemental Fig. S7C). Moreover, this esDNA-induced immunity was impaired in JA-related mutants (jar1-11 and coi1-2; Supplemental Fig. S7C). We also measured the SA content in Arabidopsis plants on 2 h after esDNA treatment and found that esDNA did not affect SA content in wild-type Arabidopsis (Supplemental Fig. S7D). These results suggest that esDNA can enhance plant resistance against both necrotrophic and hemibiotrophic pathogens, which requires the JA signaling pathway.
Finally, we tested the role of ROS production in the esDNA-induced resistance in Arabidopsis. Results showed that, compared with the wild-type Arabidopsis plants, the esDNA-elicited resistance against both B. cinerea and PstDC3000 was impaired in rbohD and rbohF mutants (Supplemental Fig. S7, D and E). Therefore, ROS production contributes to the enhanced immunity induced by esDNA.
Discussion
esDNA functions through the JA signaling pathway
Plants require reliable mechanisms to detect injury and initiate proper defense. Danger signals or DAMPs are released from stressed host cells undergoing necrosis or injury and allow self-specific immunity induction (Duran-Flores and Heil 2016; Gust et al. 2017; DeFalco and Zipfel 2021). Recent studies have identified esDNA as a DAMP that inhibits plant growth and triggers immunity induction (Barbero et al. 2016; Duran-Flores and Heil 2018; Vega-Muñoz et al. 2018; Ferrusquia-Jimenéz et al. 2022). In this study, we confirm that esDNA inhibits plant growth and induces ROS production in a concentration- and species-dependent manner. More importantly, we provide several lines of evidence supporting that esDNA functions through the JA signaling pathway. Firstly, an investigation of the esDNA-affected transcriptional profile revealed that esDNA-regulated genes are mainly enriched in the JA signaling pathway. Secondly, esDNA can induce the increases in JA-Ile and OPDA contents and activate several well-characterized JA-induced genes, such as LOX3, MYC2, and JAZ1. Thirdly, genetic analysis showed that esDNA-mediated growth inhibition, ROS production, and gene expression are impaired in the JA-related mutants jar1-11, coi1-2, and myc2-2. Finally, esDNA-triggered immunity induction to pathogens requires JA signaling.
A recent study performed a whole-plant transcriptome profiling in Arabidopsis exposed to esDNA and nonself eDNA [DNA of the animal common herring (Clupea harengus)] for 1, 8, and 16 h (Chiusano et al. 2021). In contrast to our comparison between esDNA and nonself eDNA treatments (1 h after treatment) to identify genes that are specifically regulated by esDNA, Chiusano et al. (2021) compared eDNA treatments at each stage with the mock (water) and identified 1,473 and 5,977 DEGs in esDNA and nonself eDNA treatments, respectively. When compared with the mock, we found that about 16.80% and 14.33% of the genes up- and downregulated by esDNA at 1 h identified by Chiusano et al. (2021) were also up- and downregulated by esDNA in our experiment though the method to identify DEGs is different. Of note, Chiusano et al. (2021) also observed that, among the upregulated DEGs by esDNA at 1 h, genes involved in JA signaling were enriched. In the case of nonself eDNA treatment, the authors did not find DEGs for JA, neither among upregulated nor among downregulated genes for nonself eDNA treatment at 1 h (Chiusano et al. 2021). This finding is consistent with our analysis that JA signaling is specifically activated by the esDNA treatment. As an important defensive phytohormone, JA has been well demonstrated to play critical roles in inhibiting plant growth and inducing defense responses through the master transcription factor MYC2 (Lorenzo et al. 2004; Dombrecht et al. 2007). JA was reported to inhibit root growth through MYC2-mediated transcriptional repression of PLETHORA1 (PLT1) and PLT2 in root stem cell niche (Chen et al. 2011). Our recent study has demonstrated that MYC2 orchestrates hierarchical transcriptional cascades to activate the JA-mediated plant immunity against B. cinerea (Du et al. 2017). These previous reports, together with the fact that esDNA can activate the JA signaling pathway, provide a reliable mechanism by which esDNA inhibits growth and induces immunity via the JA signaling pathway (Fig. 5C).
Our RT-qPCR analysis indicated that esDNA-induced JA-related gene expression is largely abolished in the JA mutants. However, these results cannot lead us to conclude that the esDNA-mediated effects are abolished, as we only analyzed 4 genes. Because esDNA-mediated effects, especially for root growth inhibition, are not fully eliminated in the JA-related mutants, we cannot rule out the possibility that other signaling pathways are also involved in this process. Brassinosteroids, a class of steroidal hormones, play vital roles in plant growth and immune responses to pathogens and insect herbivores (Erb et al. 2012). Our transcriptome analysis found that esDNA downregulated several genes encoding positive regulators of the brassinosteroid signaling pathway, such as AT1G74500 (ACTIVATION-TAGGED BRASSINOSTEROID INSENSITIVE1-SUPPRESSOR1) (Wang et al. 2010), AT3G56400 (WRKY70), and AT2G40750 (WRKY54) (Chen et al. 2017). Moreover, AT3G46290, encoding the receptor kinase HERCULES RECEPTOR KINASE 1 regulated by brassinosteroids and required for cell elongation (Guo et al. 2009), was downregulated by esDNA. The plant hormone auxin modulates cell proliferation and cell expansion (Zhao 2010). esDNA downregulated AT5G25620 (YUCCA6) and AT4G28720 (YUCCA8), which encode flavin monooxygenase-like proteins involved in the Trp-dependent auxin biosynthetic pathway (Zhao 2010), and AT1G68130 (INDETERMINATE-DOMAIN 14) and AT2G01940 (INDETERMINATE-DOMAIN 15), which positively regulate auxin biosynthesis (Cui et al. 2013). esDNA also downregulated several SMALL AUXIN UP RNA genes (AT1G16510 and AT1G29440), which are responsive to auxin and can stimulate cell growth (Nagpal et al. 2022). esDNA has been shown to induce cytosolic calcium elevation (Barbero et al. 2016; Rassizadeh et al. 2021). Here, we found that esDNA upregulated several CALMODULIN-LIKE (CML) genes, such as AT5G42380 (CML37) and AT1G76650 (CML38). CML37 is a positive regulator of herbivory-induced defense, and mutation of CML37 decreases the JA level in Arabidopsis (Scholz et al. 2014). CML38 can interact with the PEP1 RECEPTOR 2, a receptor for the DAMP plant elicitor peptide (Pep) 1 and Pep2, to regulate root development in Arabidopsis (Song et al. 2021). Therefore, it is possible that esDNA regulates calcium signaling to modulate JA levels. Nevertheless, the role of brassinosteroid, calcium, and auxin signaling in the esDNA-modulated plant growth and resistance requires further analysis.
In conformity with previous studies (Duran-Flores and Heil 2018; Rassizadeh et al. 2021), we found that esDNA induced resistance against both necrotrophic and biotrophic pathogens. The JA and SA signaling pathways are important for resistance to necrotrophic and biotrophic pathogens, respectively (Glazebrook 2005). It is generally acknowledged that there is an antagonistic relationship between SA and JA signaling pathways (Glazebrook 2005; Ballare 2011). Contrary to anticipation, though esDNA activated the JA signaling pathway, esDNA enhanced the resistance to the biotrophic pathogen PstDC3000 in Arabidopsis. Moreover, esDNA did not alter the SA content in Arabidopsis, indicating that esDNA-activated JA may not attenuate the SA signaling pathway. Interestingly, the esDNA-induced immunity to PstDC3000 is also dependent on the JA signaling pathway. Recent studies revealed a previously unrecognized collaborative role between JA and SA during biotrophic and hemibiotrophic pathogen defense (Tsuda et al. 2009; Hillmer et al. 2017). The plant DAMP Pep2 can coactivate the SA and JA signaling pathways (Ross et al. 2014). Therefore, esDNA may function through an unknown mechanism to enhance the resistance to PstDC3000, which warrants further investigation.
We also found that esDNA can induce the expression of RBOHF, which encodes a plasma membrane–localized NADPH oxidase contributing to ROS production (Suzuki et al. 2011). Moreover, esDNA-induced RBOHF expression and ROS production are significantly attenuated in the JA-related mutants. These results led us to propose that esDNA-mediated ROS production may be JA dependent (Fig. 5C). However, we must notify that esDNA still partially induces ROS production in the tomato jai1 mutant, in which the JA signaling should be fully blocked. This suggests that other mechanisms may be involved in the induction of ROS.
esDNA functions as a DAMP
Previously, we observed that esDNA had a concentration-dependent, species-specific inhibitory effect on the growth of organisms from several kingdoms, including plant, algae, animal, bacteria, fungi, and protozoa (Mazzoleni et al. 2015a, 2015b; Bonanomi et al. 2022). This esDNA-mediated growth inhibition is proposed as a mechanism for the negative plant–soil feedback phenomena (Mazzoleni et al. 2015a; Cartenì et al. 2016; Chiusano et al. 2021). DAMPs are endogenous signals generated in wounded or infected tissue under pathogen or insect attacks (Duran-Flores and Heil 2016; Gust et al. 2017; DeFalco and Zipfel 2021). Recent reports together with our results provided experimental evidence supporting esDNA as a DAMP because (i) esDNA can trigger the generation of ROS, calcium influx, induce defense gene expressions, and enhance resistance in plants against pathogens (Barbero et al. 2016; Duran-Flores and Heil 2018; Vega-Muñoz et al. 2018; Rassizadeh et al. 2021; Ferrusquia-Jimenéz et al. 2022); (ii) the effects of eDNA can depend on the taxonomic distance between the source and the receiver (Duran-Flores and Heil 2018; Vega-Muñoz et al. 2018); (iii) the esDNA mainly functions through the JA signaling pathway, a phytohormone that is critical for damage responses triggered by herbivore and pathogen attack; and (iv) wounding and esDNA induced similar changes in the transcriptome profile.
Although esDNA is a promising candidate of plant DAMPs, the underlying mechanisms through which the esDNA functions are largely unknown. For example, it is a challenge to unravel the molecular mechanism through which the esDNA in the environment can be detected and perceived by plant cells. DNA has long been known as a DAMP in mammals, and many DNA sensors, such as Toll-like receptor 9 (TLR9), DNA-dependent activator of interferon regulatory factors (DAI), inflammasome-forming receptors absent in melanoma 2 (AIM2), and receptor for advanced glycation end products (RAGE), have been identified in mammals (Hemmi et al. 2000; Takaoka et al. 2007; Fernandes-Alnemri et al. 2009; Sirois et al. 2013). esDNA of Arabidopsis is shown to remain outside the root cell while nonself DNA enters the cells at the early stage of exposure (60 min), and esDNA and nonself DNA differentially affected gene expressions in Arabidopsis (Chiusano et al. 2021), indicating that plant cells are able to perceive esDNA at the cell surface. Notably, consistent with previous reports (Duran-Flores and Heil 2018; Vega-Muñoz et al. 2018), the effects of esDNA on plant growth and ROS production are related to the taxonomic distance between the source and receiver. Thus, it is tempting to speculate that there is a sequence-specific recognition of small-sized eDNA mechanism in plants (Mazzoleni et al. 2015a). Clearly, the existence and identification of esDNA receptors in plants need to be a priority research subject, since it can help answer another critical question, that is, how the species-specific effect of esDNA is achieved.
Taken together, the results provided in this study led us to propose a scenario of how esDNA inhibits growth and induces defense through the JA signaling pathway (Fig. 5C). Plant esDNA, released from damaged cells, functions as a DAMP to induce the biosynthesis of the defensive hormone JA. The increase in JA content activates the JA signaling pathway by MYC2-mediated transcriptional reprogramming, which induces a large number of esDNA and JA-coregulated genes (e.g. LOX3, MYC2, and RBOHF) for growth inhibition and defense induction.
Materials and methods
Plant materials and pathogens
Seeds of Arabidopsis (A. thaliana) Col-0, tomato (cv. DN, QiYan, and Hongshengnv), wheat (cv. D123), canola (cv. HuaWei), and eggplant (cv. YingQi) were from laboratory stocks. Arabidopsis Wassilewskija seeds were kindly provided by Prof. Yanfeng Hu from the Northeast Institute of Geography and Agroecology, China. Mutants of Arabidopsis Col-0, including jar1-11, coi1-2, myc2-2, npr1-1, ein2-1, aao3-4, rbohD, and rbohF, and A. pumila were obtained from the Arabidopsis Biological Resource Center (The Ohio State University, United States of America). Tomato (S. lycopersicum L.) seeds of JA-insensitive mutant jai1 and its wild-type line CM and the JA signaling mutant MYC2-RNAi and its wild-type line M82 were kindly provided by Prof. Chuanyou Li from the Chinese Academy of Sciences, China.
Arabidopsis and tomato seeds were sterilized with 70% (v/v) ethanol and 5% (w/v) NaClO and then washed with sterile water 4 times. Then, these seeds were grown on half-strength MS medium [2.45 g L−1 of MS salt with vitamins, 1% (w/v) sucrose, and 1% (w/v) agar] plates in a growth chamber at 22 °C with a 12-h light/12-h dark cycle. Then, these seedlings were used for eDNA, phytohormones, and wounding treatments.
To prepare B. cinerea spores, B. cinerea was first grown on potato–dextrose–agar (5 g L−1 potato extract, 20 g L−1 glucose, and 15 g L−1 agar) for 7 d at 24 °C. Then, a 6-mm plug was inoculated into 300 mL of potato dextrose broth in a flask and incubated at 24 °C with shaking at 200 rpm. After 5 d, B. cinerea spores were harvested by filtering the culture through a nylon mesh to remove hyphae, and the concentration was adjusted to 5 × 105 spores mL−1. To prepare PstDC3000, the rifampicin-resistant PstDC3000 was grown on King's B agar supplemented with 50 μg mL−1 of rifampicin at 28 °C for 24 h. Bacterial cells were harvested by centrifugation for 5 min at 5,000 × g and resuspended in 10 mM MgSO4 at a final cell density of 107 cfu mL−1.
Extraction and preparation of plant DNA
To prepare plant tissues for DNA extraction, Arabidopsis, tomato, wheat, canola, and eggplant seedlings were grown in nutritive soils in a growth chamber at 22 °C with a 12-h light/12-h dark cycle. Plant leaves were collected from 4-wk-old plants and then frozen with liquid nitrogen and stored at −80 °C. Plant genomic DNA was extracted using a high-salt cetyltrimethylammonium bromide (CTAB) extraction protocol, and a prewash step using a buffered sorbitol solution was added before DNA extraction (Inglis et al. 2018). The quality and yield of extracted DNA were checked with 2% (w/v) agarose gel electrophoresis and a NanoDrop 2000 spectrophotometer (ThermoFisher Scientific, Wilmington, United States of America). Fragmented DNA was obtained by sonication using a SCIENTZ-950E ultrasonic processor (Ningbo Scientz Biotechnology, Ningbo, China). Briefly, a solution of DNA prepared with sterile water (500 μg mL−1) was sonicated for 8 min with a 3-s pulse “on” and 1-s pulse “off.” The fragment of DNA was verified by 2% (w/v) agarose gel electrophoresis.
Root growth inhibition assays
For experiments testing the effects of eDNA on primary root growth of Arabidopsis, 5-d-old Arabidopsis seedlings were transferred to 24-well plates containing liquid half-strength MS medium with the roots immersed in the growth medium. There were 6 seedlings per well. Then, Arabidopsis seedlings were treated with eDNA by adding fragmented DNA solution into the growth medium. To test the effects of different concentrations of esDNA on Arabidopsis Col-0 root growth, Arabidopsis Col-0 seedlings were treated with esDNA at final concentrations of 0.02, 0.2, 2, 20, 100, 200, and 500 μg mL−1, respectively. To test the effect of esDNA vs. nonself eDNA on Arabidopsis Col-0 root growth, Arabidopsis Col-0 seedlings were treated with esDNA (i.e. DNA from Arabidopsis Col-0) and nonself eDNAs from Arabidopsis Wassilewskija, A. pumila, canola, tomato cv. DN, and wheat at a final concentration of 200 μg mL−1, respectively. To compare the response between Arabidopsis Col-0 wild type and mutants to eDNA, Arabidopsis Col-0 wild-type seedlings were treated with eDNA from the wild type and each mutant (i.e. jar1-11, coi1-2, myc2-2, npr1-1, ein2-1, aao3-4, rbohD, and rbohF) at a final concentration of 200 μg mL−1. Meanwhile, seedlings of each mutant were treated with eDNA from the wild-type and the mutant itself at a final concentration of 200 μg mL−1. For each experiment, seedlings treated with sterile water served as the control. Each treatment was repeated 3 times with 6 seedlings per replicate. All seedlings were kept in a growth chamber at 22 °C with a 12-h light/12-h dark cycle. The primary root length was measured by a ruler at 3 d after treatment.
For experiments testing the effects of eDNA on tomato primary root growth, surface-sterilized tomato seeds were germinated in 9-cm Petri dishes, which contained 2 layers of sterile filter papers imbibed with 5 mL of sterile water. Then, the pregerminated tomato seeds with radicles of 2 mm length were put on sterile dry filter paper in 5-cm Petri dishes and then treated with fragmented eDNA. There were 10 seeds per Petri dish. To test the effects of different concentrations of esDNA on the root growth of tomato cv. DN, tomato cv. DN seeds were treated with esDNA at concentrations of 0.02, 0.2, 2, 20, 100, 200, and 500 μg mL−1. To test the effects of esDNA vs. nonself eDNA on tomato cv. DN root growth, tomato cv. DN seeds were treated with esDNA (i.e. DNA from DN) and nonself eDNAs from tomato cv. QiYan, tomato cv. Hongshengnv, eggplant, Arabidopsis Col-0, and wheat at a final concentration of 200 μg mL−1. To compare the response between tomato wild type and mutants to eDNA, germinated seeds of the mutant jai1 and its wild-type line CM were treated with 200 μg mL−1 of eDNA from the wild-type line CM, while germinated seeds of the mutant MYC2-RNAi and its wild-type line M82 were treated with 200 μg mL−1 of eDNA from the wild-type line M82. Germinated seeds treated with sterile water served as the control. Each treatment was repeated 3 times with 10 seeds per replicate. All tomato seeds were kept in a growth chamber at 20 °C under continuous light. The primary root length was measured by a ruler at 4 d after treatment.
esDNA-induced H2O2 production assays
Arabidopsis and tomato seedlings were grown in nutritive soils in a growth chamber at 22 °C with a 12-h light/12-h dark cycle. Leaves of 4-wk-old seedlings were treated with 200 μg mL−1 fragmented eDNA or water (the control). DNA solution or sterile water was applied on both sides of 3 randomly selected leaves until the surface was completely wet. To test the effects of esDNA vs. nonself eDNA on the H2O2 content in Arabidopsis leaves, Arabidopsis Col-0 leaves were treated with esDNA or nonself eDNA from Arabidopsis Wassilewskija, A. pumila, canola, tomato, and wheat, respectively. To test the effects of esDNA vs. nonself eDNA on H2O2 content in tomato leaves, tomato cv. DN leaves were treated with esDNA or nonself eDNA from tomato cv. QiYan, tomato cv. Hongshengnv, eggplant, Arabidopsis Col-0, and wheat, respectively. To compare the esDNA-mediated H2O2 generation between Arabidopsis Col-0 wild type and mutants, Arabidopsis Col-0 and mutants jar1-11, coi1-2, and myc2-2 were treated with eDNA from Arabidopsis Col-0. To compare the esDNA-mediated H2O2 generation between tomato wild type and mutants, tomato wild-type line CM and the mutant jai1 were treated with eDNA from wild-type line CM, while wild-type line M82 and MYC2-RNAi were treated with eDNA from wild-type line M82. Each treatment was repeated 3 times with 3 seedlings per replicate. H2O2 contents in plant leaves were visualized and quantified at 60 min after treatment.
The diaminobenzidine (DAB) staining method was used to visualize H2O2 generation in Arabidopsis and tomato leaves as previously described (Müller et al. 2009). Briefly, sampled plant leaves were transferred to the staining solution containing 1 mg mL−1 DAB in 10 mM 2-(N-morpholino)ethanesulfonic acid (MES) buffer (pH 5.6). Then, leaves were kept in the staining solution under daylight at room temperature for 7 h. The stained leaves were washed with a methanol–acetone (3:1) mixture to remove chlorophyll. Finally, stained leaves were photographed with a stereo SZX2-ILLK microscope (Olympus, Tokyo, Japan). The H2O2 content in plant leaves was quantified as previously described (Mukherjee and Choudhuri 1983; Jin et al. 2023). Briefly, leaf tissues were ground in ice-cold acetone and then added with 5% (w/v) titanyl sulfate and NH4OH solution. After being vortexed for a few seconds, the mixture was centrifuged at 12,000 × g for 8 min. Then, the supernatant was discarded and the pellet was dissolved in 2 M H2SO4. The solution absorbance was measured at 410 nm. H2O2 content was calculated based on standard curves with known concentrations of H2O2.
Testing effects of RNase, proteinase, and DNase on esDNA-induced H2O2 production
To confirm that the observed fragmented esDNA-induced H2O2 production was due to DNA but not impurities such as RNAs or proteins in the extracted DNA solution, fragmented DNA from Arabidopsis Col-0 was treated with RNase A, proteinase K, or DNase I (Solarbio, Beijing, China) according to the product manual. Briefly, fragmented DNA solutions (1,000 μg mL−1) were treated with 5 μg mL−1 RNase A and incubated at 37 °C for 12 h or treated with 50 μg mL−1 proteinase K and incubated at 58 °C for 2 h or treated with 0.067 μg μL−1 DNase I and incubated at 37 °C for 2 h, respectively. Arabidopsis Col-0 seedlings were treated with RNase A-, proteinase K-, or DNase I-treated DNA solutions containing 200 μg mL−1 DNA. Seedlings treated with 200 μg mL−1 DNA that were not treated with RNase A, proteinase K, or DNase I served as the positive control. Meanwhile, the putative direct effects of the enzymes were tested by applying enzyme solutions (5 μg mL−1 RNase A, 50 μg mL−1 proteinase K, and 0.067 μg μL−1 DNase I) on the leaves. The DNA or enzyme solutions were applied on both sides of 3 randomly selected leaves until the surface was completely wet. Each treatment was repeated 3 times with 5 seedlings per replicate. Accumulation of H2O2 in the treated leaves was analyzed at 60 min after treatment as described above.
To compare the effects of fragmented esDNA and nonfragmented esDNA on H2O2 generation, Arabidopsis Col-0 seedling leaves were treated with fragmented DNA, nonfragmented DNA, and sterile water. Each treatment was repeated 3 times with 5 seedlings per replicate. DNA solution treatment and H2O2 measurement were performed as described above.
Testing effects of eDNA, plant hormones, and wounding on gene expression and JA content
For Arabidopsis seedlings, 10-d-old Arabidopsis Col-0 seedlings were individually transferred to a single well of a 24-well plate with liquid half-strength MS medium with the roots immersed in the growth medium. The DNA or plant hormones were added into the growth medium at the following final concentrations: 200 μg mL−1Arabidopsis Col-0 fragmented self-DNA (esDNA) and tomato cv. DN fragmented eDNA (TeDNA), 10 μ M ACC (Shi et al. 2012), 50 μ M ABA (Böhmer and Schroeder 2011), 0.5 mM SA (Blanco et al. 2009), and 50 μ M MeJA (Du et al. 2017). The DNA solution was dissolved in sterile water, and thus, sterile water served as the control for DNA treatments. The ACC, ABA, and SA were dissolved in dimethyl sulfoxide, and thus, dimethyl sulfoxide with a final concentration of 0.05% (v/v) in the growth medium served as the control for these 3 treatments. The MeJA was dissolved in ethanol, and thus, ethanol was added to the growth medium at a final concentration of 0.2% (v/v) as a control. For the mechanical wounding treatment, Arabidopsis Col-0 seedlings were consecutively wounded 3 times using hemostatic forceps (5-mm round tip with the line-serrated surface; Mousavi et al. 2013), while nonwounded seedlings served as the control.
For tomato seedlings, 7-d-old tomato DN seedlings were transferred into liquid half-strength MS medium with the roots immersed in the growth medium. Then, DNA solution (200 μg mL−1) or sterile water was added in to the growth medium. Arabidopsis and tomato seedlings were kept in a growth chamber at 20 °C with a 12-h light/12-h dark cycle. Arabidopsis or tomato seedlings were harvested at 0, 30, 60, and 120 min after treatment and immediately frozen in liquid nitrogen and stored at −80 °C. At each time point, 12 seedlings in each treatment per replicate were sampled and combined to make a composite sample. Samples harvested at 60 min were used for RNA-seq (only for Arabidopsis) and JA content analysis, while samples harvested at 0, 30, 60, and 120 min were used for RT-qPCR analysis of selected genes. There were 4 composite samples for RNA-seq analysis and 3 composite samples for JA content and RT-qPCR analyses.
RNA extraction, RNA-seq, and data analysis
The total RNA was extracted with an OmniPlant RNA Kit (ComWin Biotech, Beijing, China) according to the manufacturer's instructions followed by an additional DNA digestion step using DNAse I (Sigma, Darmstadt, Germany). The quantity and quality of RNA were determined by a NanoDrop2000 spectrophotometer (ThermoFisher Scientific, Wilmington, United States of America). For transcriptome analysis, RNA libraries were constructed using a TruSeq RNA Sample Prep Kit (Illumina Inc., San Diego, United States of America) following the manufacturer's instructions. Briefly, mRNA was enriched using magnetic beads with Oligo (dT) and fragmented using divalent cations at elevated temperature. The RNA fragments were reverse transcribed into first-strand cDNA with 6-base random primers, followed by second-strand cDNA synthesis, 3′-end repair, and ligation of adapters. The ligated fragments were enriched by PCR to generate the final cDNA library. Finally, the libraries were sequenced on an Illumina HiSeq 2500 platform (Illumina Inc., San Diego, United States of America).
The raw paired-end reads were filtered to obtain high-quality clean reads using fastp 0.19.5 (Chen et al. 2018), and low-quality reads, adapter-containing reads, and poly-N reads were removed. About 6 Gb of high-quality 150-bp paired-end reads was generated from each library. RNA-seq reads were aligned to adaptor, rRNA, and tRNA sequences using Bowtie2 (2.4.1) (Langmead and Salzberg 2012), and the remaining filtered reads were aligned to the Arabidopsis reference genome TAIR10 (http://plants.ensembl.org/Arabidopsis_thaliana/Info/Index) using TopHat2 with default parameters (Trapnell et al. 2009). The counting of reads per gene was performed using HTSeq v0.9.1 with the union mode (Anders et al. 2015). Genes expressed at low levels were excluded by filtering out genes with a mean read count below 20 counts per sample. Principal component analyses were performed to compare the global expression patterns between the esDNA, TeDNA, and water treatments. DEGs between treatments were analyzed using the R package “DESeq2” (Love et al. 2014). The genes which adjusted P ≤ 0.01 and log2FC ≥ 1 were considered DEGs. The GO categories consisting of 3 structured networks, e.g. biological process, cellular component, and molecular function, of defined terms were derived from GO (www.geneontology.org). The DEGs were clustered by GO term analysis using the R package “clusterProfiler.” The log2FC values of DEGs were used for generating heat maps using the R package “pheatmap.” An integrative genomics viewer browser screenshot was obtained from the published ChIP-seq analysis (Wang et al. 2019). The G-box motif of AtRBOHF was analyzed using the National Center for Biotechnology Information (NCBI).
RT-qPCR analysis
The expression levels of selected JA-responsive genes (i.e. LoxD, MYC2, JAZ1, and RBOH) were analyzed using SYBR Green-based RT-qPCR on a qTOWER real-time PCR system (Analytik Jena, Jena, Germany). PCR assays were performed in a 20 μL reaction mixture containing 10 μL of SYBR Green qPCR Master Mix (Tiangen Biotech, Beijing, China), 8.8 μL of sterile water, 0.6 μL of cDNA, and 0.3 μL each of forward and reverse primers. PCR protocol was 10 min at 95 °C, 40 cycles of 10 s at 95 °C, 30 s at 58 °C, and 40 s at 72 °C. The actin gene was used as the reference gene. Primers used in this study are listed in Supplemental Table S1. Sterile water instead of cDNA served as the negative control. All amplifications were performed in triplicate. The relative expressions of these genes were calculated with the 2−ΔΔCT method (Livak and Schmittgen 2001).
Quantification of hormones
Fresh plant samples were frozen in liquid nitrogen and ground into powder. Then, 80 mg of plant sample powder was weighed into a 2-mL plastic microtube, frozen in liquid nitrogen, and dissolved in 1 mL of methanol/water/formic acid (15:4:1, v/v/v) mixture. Then, 10 μL of internal standards was added. The mixture was filtered through a 0.22-μm membrane filter and analyzed using an ultra-high-pressure liquid chromatography (UPLC)-electrospray ionization (ESI)-mass spectrometry (MS)/MS system (UPLC, SCIEX ExionLC AD; MS, SCIEX QTRAP 6500+), equipped with an ESI Turbo Ion-Spray interface, operating in both positive and negative ion modes, and controlled by SCIEX Analyst software 1.6.3. The liquid chromatography was as follows: column, Waters ACQUITY UPLC HSS T3 C18 (100 mm × 2.1 mm, 1.8 µm); solvent system, water with 0.04% acetic acid (A) and acetonitrile with 0.04% acetic acid (B); gradient program, started at 5% B (0 to 1 min), increased to 95% B (1 to 8 min), 95% B (8 to 9 min), and finally ramped back to 5% B (9.1 to 12 min); flow rate, 0.35 mL min−1; temperature, 40 °C; and injection volume: 2 μL (Niu et al. 2014; Xiao et al. 2018). Parameters used for mass spectrometry were as follows: electrospray ionization; ion temperature, 550 °C; ion-spray voltage, −4,500 V (negative mode)/5,500 V (positive mode); and curtain gas, 35 psi. Contents of JA-Ile, OPDA, and SA were calculated from the standard curves made with known concentrations of JA-Ile, OPDA, and SA and expressed as ng g−1 fresh weight (FW).
Pathogen infection assays
Arabidopsis or tomato plants were grown in nutritive soil in a growth chamber at 22 °C with a 12-h light/12-h dark cycle. Leaves of 4-wk-old Arabidopsis or tomato wild-type and mutants (2 leaves per plant) were sprayed with 200 μg mL−1 of eDNA from the wild type. Specifically, Arabidopsis Col-0 and mutants jar1-11, coi1-2, rbohD, and rbohF were treated with eDNA from Arabidopsis Col-0. Tomato wild-type line CM and the mutant jai1 were treated with eDNA from wild-type line CM, while wild-type line M82 and MYC2-RNAi were treated with eDNA from wild-type line M82. Control seedlings were treated with sterile water. The B. cinerea inoculation assay was performed with Arabidopsis and tomato, while the PstDC3000 inoculation assay was performed with Arabidopsis.
For the B. cinerea inoculation assay, 1 systemic leaf per plant (i.e. leaves untreated with eDNA) was detached at 24 h after DNA treatment and placed in Petri dishes containing 1% (w/v) phytoagar. Each leaf was then spotted with a single 5 μL droplet of B. cinerea spore suspension (5 × 105 spores mL−1) (Du et al. 2017). Petri dishes were covered with lids sealed with parafilm to prevent the desiccation of the inoculation drops. Each treatment was repeated 5 times with 3 seedlings per replicate. After incubating at 22 °C for 72 h, leaves were photographed using a stereo SZX2-ILLK microscope (Olympus, Tokyo, Japan) and the lesion area was measured using ImageJ software (1.8.0).
For the PstDC3000 inoculation assay, 1 systemic leaf per plant (i.e. leaves untreated with eDNA) was inoculated by spraying a solution of PstDC3000 (107 cfu mL−1) at 24 h after DNA treatment. Each treatment was repeated 5 times with 3 seedlings per replicate. Eight days after inoculation, the inoculated leaves were photographed and PstDC3000 populations were monitored by serial dilution assays on King's B agar supplemented with 50 μg mL−1 of rifampicin.
Statistical analyses
For data of plant primary root length, H2O2 content, gene expression level, hormone content, and B. cinerea lesion area, normal distribution and homogeneity of variances were checked with the Shapiro–Wilk test and Levene's test, respectively. Then, for experiments with more than 2 treatments, data were analyzed with 1-way analysis of variance (ANOVA), and means were compared between treatments by Tukey's HSD test. For experiments with 2 treatments, the comparison between 2 groups was performed using Student's t test. Differences were considered statistically significant at P < 0.05. The phylogenetic tree of the plant species used in this study was constructed using the “V.PhyloMaker2” package in R.
Accession numbers
Sequencing data from this article can be found in the GenBank data libraries under the accession number PRJNA910862.
Acknowledgments
We thank Prof. Yanfeng Hu (Northeast Institute of Geography and Agroecology, China) and Prof. Chuanyou Li (Chinese Academy of Sciences, China) for kindly providing us plant materials.
Author Contributions
X.G.Z., H.G., M.D., and F.W. designed the experiments. X.G.Z., H.G., and X.H.Z. performed the experiments. X.G.Z., H.G., and M.D analyzed the data. X.G.Z., M.K.R., S.M., and M.D. wrote the manuscript. All authors read and approved the final manuscript.
Supplemental data
The following materials are available in the online version of this article.
Supplemental Figure S1. The electropherogram of unfragmented and fragmented genomic DNA and phylogenetic distances between plant species.
Supplemental Figure S2. esDNA is required to induce H2O2 production in Arabidopsis.
Supplemental Figure S3. Comparison of gene expression patterns and overlap of upregulated and downregulated DEGs.
Supplemental Figure S4. eDNAs from the wild-type and mutant Arabidopsis Col-0 have similar inhibitory effects on primary root growth.
Supplemental Figure S5. H2O2 accumulation in response to esDNA is attenuated in JA mutants.
Supplemental Figure S6. esDNA alters the expression of MYC2-bound genes in Arabidopsis.
Supplemental Figure S7. esDNA induced resistance to PstDC3000 and the role of the RBOHD and RBOHF in eDNA-mediated biological effects.
Supplemental Table S1. Primers used for RT-qPCR analysis.
Supplemental Data Set 1. DEGs in treatments of esDNA, plant hormones, and wounding.
Funding
This study was funded by the National Natural Science Foundation of China (32072655 and 32272792) and the China Agriculture Research System of MOF and MARA (CARS-23-B-10).
Data availability
The authors confirm that all experimental data are available and accessible via the main text and/or the supplemental data.
References
Author notes
The author responsible for distribution of materials integral to the findings presented in this article in accordance with the policy described in the Instructions for Authors (https://dbpia.nl.go.kr/plphys/pages/General-Instructions) is Fengzhi Wu ([email protected]).
Conflict of interest statement. The authors have no conflict of interest.