-
PDF
- Split View
-
Views
-
Cite
Cite
Takatoshi Kiba, Kahori Mizutani, Aimi Nakahara, Yumiko Takebayashi, Mikiko Kojima, Tokunori Hobo, Yuriko Osakabe, Keishi Osakabe, Hitoshi Sakakibara, The trans-zeatin-type side-chain modification of cytokinins controls rice growth, Plant Physiology, Volume 192, Issue 3, July 2023, Pages 2457–2474, https://doi.org/10.1093/plphys/kiad197
- Share Icon Share
Abstract
Cytokinins (CKs), a class of phytohormones with vital roles in growth and development, occur naturally with various side-chain structures, including N6-(Δ2-isopentenyl)adenine-, cis-zeatin- and trans-zeatin (tZ)-types. Recent studies in the model dicot plant Arabidopsis (Arabidopsis thaliana) have demonstrated that tZ-type CKs are biosynthesized via cytochrome P450 monooxygenase (P450) CYP735A and have a specific function in shoot growth promotion. Although the function of some of these CKs has been demonstrated in a few dicotyledonous plant species, the importance of these variations and their biosynthetic mechanism and function in monocots and in plants with distinctive side-chain profiles other than Arabidopsis, such as rice (Oryza sativa), remain elusive. In this study, we characterized CYP735A3 and CYP735A4 to investigate the role of tZ-type CKs in rice. Complementation test of the Arabidopsis CYP735A-deficient mutant and CK profiling of loss-of-function rice mutant cyp735a3 cyp735a4 demonstrated that CYP735A3 and CYP735A4 encode P450s required for tZ-type side-chain modification in rice. CYP735As are expressed in both roots and shoots. The cyp735a3 cyp735a4 mutants exhibited growth retardation concomitant with reduction in CK activity in both roots and shoots, indicating that tZ-type CKs function in growth promotion of both organs. Expression analysis revealed that tZ-type CK biosynthesis is negatively regulated by auxin, abscisic acid, and CK and positively by dual nitrogen nutrient signals, namely glutamine-related and nitrate-specific signals. These results suggest that tZ-type CKs control the growth of both roots and shoots in response to internal and environmental cues in rice.
Introduction
Cytokinins (CKs), a group of plant hormones, are involved in the regulation of various aspects of plant growth and development, including cell division and differentiation, organogenesis, root and shoot growth, and environmental responses (Mok and Mok 2001; Sakakibara 2006; Werner and Schmülling 2009; Kiba et al. 2011; Kieber and Schaller 2018; Kiba et al. 2019; Sakakibara 2021). Natural CKs are mostly derivatives of adenine with a prenyl side chain at the N6 position. There are variations in the side-chain structure of CKs and the most common structures in plants are N6-(Δ2-isopentenyl)adenine (iP)-, trans-zeatin (tZ)-, and cis-zeatin (cZ)-types, which differ in the presence and stereoisomeric position of the terminal hydroxyl group on the prenyl side chain (Shaw 1994; Mok and Mok 2001; Gajdošová et al. 2011).
Our understanding of biosynthetic pathways of the side-chain variants has greatly progressed, thanks mostly to studies in Arabidopsis. The initial step of de novo iP- and tZ-type CK biosynthesis is catalyzed by adenosine phosphate-isopentenyltransferase (IPT) to produce iP-type CK nucleotide precursors, iP ribotides (iPRPs) (Kakimoto 2001; Takei et al. 2001a). tZ-type side chain is formed by the enzyme cytochrome P450 monooxygenase CYP735A, which is encoded by CYP735A1 and CYP735A2 in Arabidopsis (Takei et al. 2004; Kiba et al. 2013). CYP735A trans-hydroxylates the prenyl side chain of iPRPs to produce tZ ribotides (tZRPs). Finally, the CK-activating enzyme LONELY GUY (LOG) converts the nucleotide precursors to their active nucleobase forms, iP and tZ (Kurakawa et al. 2007; Kuroha et al. 2009; Tokunaga et al. 2012). The cZ-type CKs are biosynthesized via the prenylation of tRNA by tRNA-isopentenyltransferase (tRNA-IPT) and the degradation of the prenylated tRNA (Miyawaki et al. 2006; Gajdošová et al. 2011). Genes responsible for prenylated tRNA degradation for cZ-type CK production have not been identified yet.
CK biosynthesis is regulated by various internal and environmental factors (Hirose et al. 2008; Zwack and Rashotte 2015). Nitrogen nutrition, which is available mostly as nitrate and ammonium in soil (Miller and Cramer 2004; Kirk and Kronzucker 2005; Xu et al. 2012; Kiba and Krapp 2016; Coskun et al. 2017), is one of the major factors. Studies in Arabidopsis showed that IPT3 and CYP735As are induced by a nitrate-specific signal to boost iP- and tZ-type CK accumulation in Arabidopsis (Miyawaki et al. 2004; Takei et al. 2004; Engelsberger and Schulze 2012; Maeda et al. 2018). OsIPT4 and OsIPT5 are not induced by nitrate itself but by ammonium and glutamine with accompanying accumulation of iP-type and tZ-type CKs, indicating that de novo iP- and tZ-type CK biosynthesis is regulated by glutamine-related signals in rice (Oryza sativa) (Kamada-Nobusada et al. 2013). A CYP735A gene in Oryza longistaminata, a wild rice, was shown to be upregulated by ammonium nitrate but the signal responsible for the upregulation is not known (Shibasaki et al. 2021). Thus, plants have dual systems, nitrate-specific and glutamine-related systems, to modulate the iP-type and tZ-type CK biosynthesis in response to nitrogen availability. Although the underlying mechanism is not understood, cZ-type CK levels are generally increased in response to biotic and abiotic stresses, such as phosphate starvation, low temperature, drought, and salt treatment (Vyroubalová et al. 2009; Macková et al. 2013; Vanková et al. 2014; Silva-Navas et al. 2019).
Previous studies have suggested that there is biological relevance to side-chain variations of CKs (Schmitz and Skoog 1972; Mok et al. 1978; Werner et al. 2003; Spíchal et al. 2004; Gajdošová et al. 2011; Stolz et al. 2011; Choi et al. 2012; Osugi and Sakakibara 2015; Schäfer et al. 2015; Hluska et al. 2021). However, it is only recently that studies in Arabidopsis demonstrated it. The abundance of iP- and tZ-type CKs is higher than that of cZ-type (Osugi and Sakakibara 2015; Osugi et al. 2017; Kiba et al. 2019) and tZ-type CKs are the most active, while cZ-type CKs are the least active in bioassays in Arabidopsis (Gajdošová et al. 2011; Kudo et al. 2012). Arabidopsis CK receptors have different affinities toward side-chain variants (Spíchal et al. 2004; Romanov et al. 2006; Stolz et al. 2011). For example, ARABIDOPSIS HISTIDINE KINASE 2 (AHK2) and ARABIDOPSIS HISTIDINE KINASE 4/WOODEN LEG/CYTOKININ RESPONSE 1 (AHK4/WOL/CRE1) have high affinity to both iP and tZ, while ARABIDOPSIS HISTIDINE KINASE 3 (AHK3) binds to iP with a 10 times lower affinity than tZ in in vitro binding assays. Consistent with the bioassay, the affinity of AHKs to cZ is substantially lower compared with that of iP and tZ (Spíchal et al. 2004; Romanov et al. 2006; Stolz et al. 2011). Furthermore, characterization of the Arabidopsis cyp735a1 cyp735a2 mutant that is impaired in tZ-type side-chain modification has unequivocally shown that the function of tZ-type CKs is shoot growth promotion, and it is different from iP-type and cZ-type CKs (Kiba et al. 2013). Thus, tZ-type CKs are the major active variants possessing a specific function in shoot growth, while cZ-type CKs are the weakly active or inactive forms with minor roles in Arabidopsis.
Although the occurrence of the 3 side-chain variants is ubiquitous among plants (Gajdošová et al. 2011), several lines of evidence suggest that their functional differentiation may not be as universal. For instance, the tZ-type CKs is not consistently more abundant than the cZ-type CKs among all plants. Plants that contain higher levels of cZ-type CKs than tZ-type can be found across the whole evolutionary tree of land plants (Gajdošová et al. 2011). Interestingly, many crops, including pea (Pisum sativum), potato (Solanum tuberosum), oats (Avena sativa), maize (Zea mays), and rice, are cZ-type-abundant plants (Gajdošová et al. 2011; Schäfer et al. 2015; Hluska et al. 2021). Furthermore, cZ-type CKs are shown to be as active as tZ-type in some bioassays, including CK-responsive gene expression assay in maize cultured cell (Yonekura-Sakakibara et al. 2004) and seminal root growth inhibition assay in rice (Kudo et al. 2012). Consistently, CK receptors of maize (ZmHK1) and rice (OsHK3 and OsHK4) were shown to have similar affinity toward cZ and tZ (Lomin et al. 2011; Choi et al. 2012). However, the exact function of each of these variants in plants other than Arabidopsis, including monocot and cZ-type-abundant plants, is still unknown and remains to be determined.
Rice is a monocot plant possessing a substantially distinctive CK side-chain profile from Arabidopsis; cZ-type CKs comprise more than 80% of total CK in various tissues (Kojima et al. 2009; Kudo et al. 2012; Osugi and Sakakibara 2015). In this study, we characterized the consequence of disruption of rice CYP735As (CYP735A3 and CYP735A4) and demonstrated that these genes are vital for tZ-type CK biosynthesis. Our results also revealed that tZ-type CK biosynthesis is regulated by phytohormones and dual nitrogen signals and that tZ-type CKs act to promote root and shoot growth in rice. These results suggest that the physiological role of tZ-type CKs in rice is different from that in Arabidopsis despite the fact that the biosynthetic mechanism is conserved. The function of tZ-type CKs might be optimized for each plant species depending on the body plan and/or growth environments.
Results
CYP735A3 and CYP735A4 complement the Arabidopsis cyp735a1 cyp735a2 mutant
In the rice genome, CYP735A3 and CYP735A4 encode proteins with high amino acid sequence similarity to CYP735A1 and CYP735A2, which are cytochrome P450 monooxygenases for tZ-type CK biosynthesis in Arabidopsis (Supplemental Fig. S1) (Hansen et al. 2021). The cyp735a1 cyp735a2 mutant (atcypDM) is deficient in tZ-type CKs resulting in shoot growth retardation (Kiba et al. 2013). To assess whether these genes encode enzymes that catalyze the same reaction as CYP735A1 and CYP735A2, we expressed them in atcypDM under the control of cauliflower mosaic virus (CaMV) 35S promoter. Accumulation of CYP735A3 or CYP735A4 transcripts was confirmed by reverse transcription quantitative PCR (RT-qPCR) analysis in 2 independent lines of transgenic plants CYP735A3-ox or CYP735A4-ox, respectively (Fig. 1A). When grown on soil, all the transgenic lines developed a rosette with significantly greater diameter than atcypDM (Fig. 1B). Both CYP735A3 and CYP735A4 expression resulted in an increase of tZ-type CK (tZ and its conjugates) concentration with a concomitant decrease of iP-type CK (iP and its conjugates) concentration compared with atcypDM, but no significant differences in cZ-type CK (cZ and its conjugates) concentration were observed (Fig. 1C; Supplemental Table S1). These results demonstrate that CYP735A3 and CYP735A4 catalyze the same reaction as CYP735A1 and CYP735A2, which is the trans-hydroxylation of CK side chain to synthesize tZ-type CKs from iP-type CKs.
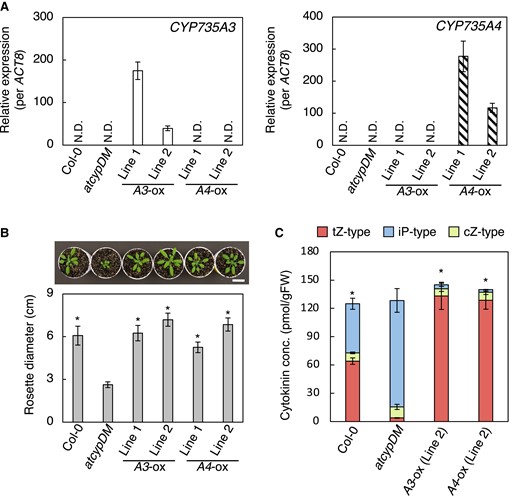
Recovery of trans-zeatin-type cytokinin deficiency in the Arabidopsis mutant cyp735a1 cyp735a2 by CYP735A3 or CYP735A4.A) RT-qPCR analysis of CYP735A3 (left) or CYP735A4 (right) expression in whole seedlings of Col-0, cyp735a1 cyp735a2 mutant (atcypDM), and transgenic plants constitutively expressing CYP735A3 (A3-ox) or CYP735A4 (A4-ox) in atcypDM background. Seedlings were grown on 1/2x MS agar plates for 12 d before harvest. Expression levels were normalized using ACT8 as an internal control. Error bars represent standard deviation (n = 4 independent pools of more than 10 plants). N.D., under the quantification detection limit. B) Rosette diameter of 28-d-old Col-0, atcypDM, and transgenic plants constitutively expressing CYP735A3 (A3-ox) or CYP735A4 (A4-ox) in atcypDM background grown on soil. A photograph of representative plants is shown. Error bars represent standard deviation (n = 7). Asterisks indicate statistically significant differences compared with atcypDM (P < 0.01, Dunnet's test). Scale bar, 3 cm. C) Cytokinin concentrations in whole seedlings of Col-0, atcypDM, and transgenic plants constitutively expressing CYP735A3 (A3-ox) or CYP735A4 (A4-ox) in atcypDM background. Seedlings were grown on 1/2x MS agar plates for 12 d before harvest. Cytokinin levels were determined using an ultra-performance liquid chromatograph coupled with a tandem quadrupole mass spectrometer with an octadecylsilyl column and were normalized to the fresh weight of each sample. Error bars represent standard deviation (n = 4 independent pools of more than 10 plants). Asterisks indicate statistically significant differences in tZ-type cytokinin concentration compared with atcypDM (P < 0.01, Dunnet's test). The concentration of each cytokinin molecular species in roots and shoots is shown in Supplemental Table S1. gFW, gram fresh weight; tZ-type, trans-zeatin and its conjugates; cZ-type, cis-zeatin and its conjugates; iP-type, N6-(Δ2-isopentenyl)adenine and its conjugates.
The spatial expression pattern of CYP735A3 and CYP735A4
Expression levels of CYP735A3 and CYP735A4 were analyzed by RT-qPCR in various organs of rice in seedling to early reproductive phases (Fig. 2). At seedling phase, transcripts of CYP735As were detected both in roots and shoots but they were more abundant in roots as reported previously (Tsai et al. 2012) (Fig. 2A). In later phases tested, the accumulation of the CYP735A3 transcript was the highest around the vegetative shoot apex, while that of CYP735A4 transcript was prominent in the leaf blade of plants at vegetative and early reproductive phases (Fig. 2B).
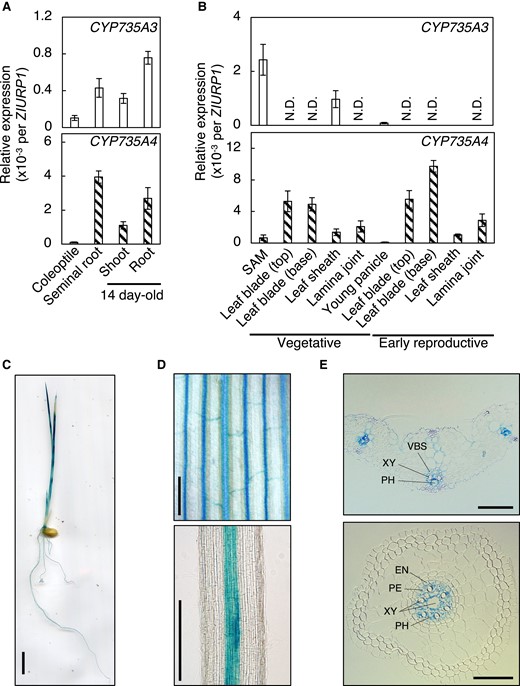
Expression pattern of CYP735A3 and CYP735A4 in various tissues. A) Expression of CYP735A3 and CYP735A4 in Nipponbare seedlings. Coleoptiles and seminal roots, and roots and shoots were harvested 4 d and 14 d, respectively, after imbibition. Error bars represent standard deviation of biological replicates (n = 4 to 5 independent pools of more than 2 plants). B) Expression of CYP735A3 and CYP735A4 in Nipponbare plants in vegetative or early reproductive stages. Forty-two-day-old (vegetative stage) and older plants (reproductive stage) were used. The shoot apex parts of 42-d-old plants were collected as shoot apical meristem (SAM). Leaf blade, leaf sheath, and lamina joint were harvested from the youngest fully expanded leaf. The young panicle is a mixture of 2 to 21 mm long young panicles collected from plants at the reproductive stage. Expression levels were quantified by RT-qPCR analysis and normalized to ZIURP1 as an internal control. Error bars represent standard deviation of biological replicates (n = 4 except for SAM and young panicle, n = 4 and n = 7 independent pools of more than 2 plants for SAM and young panicle, respectively). N.D., under the quantification detection limit. C to E) Representative images of GUS staining obtained from proCYP735A3:GUS transgenic seedlings grown for 8 d after imbibition. Pictures of a whole seedling C), first leaf D, upper), crown root D, lower), cross section of the first leaf E, upper), and cross section of seminal root E, lower) are presented. VBS, vascular bundle sheath; XY, xylem; PH, phloem; EN, endodermis; PE, pericycle. Scale bars, C) 1 cm; D) 200 µm; E) 50 µm.
In order to localize CYP735As expression at the tissue level, we used GUS staining technique. For this purpose, we generated transgenic plants harboring a fusion of the 3.6 kb CYP735A upstream sequence and the GUS reporter gene (proCYP735A3:GUS and proCYP735A4:GUS). Similar staining patterns were observed in multiple independent T1 lines of proCYP735A3:GUS (Fig. 2, C to E). However, no staining was detected in any of twelve independent proCYP735A4:GUS lines tested, suggesting that the 3.6 kb upstream sequence of CYP735A4 was not sufficient to drive expression. Consistent with the RT-qPCR results (Fig. 2A), staining was detected both in roots and shoots of proCYP735A3:GUS seedlings (Fig. 2C). The GUS activity was restricted to the vascular bundle (Fig. 2D) and observation of cross-sections revealed that the staining was pronounced in vascular parenchyma cells in the shoot, and vascular parenchyma and pericycle cells in the root (Fig. 2E).
CYP735A3 and CYP735A4 play a central role in tZ-type cytokinin biosynthesis in rice
To examine the role of CYP735As in CK side-chain modification in rice, we disrupted CYP735A3 and CYP735A4 using the CRISPR-Cas9 system. Two transfer RNA-based-multiplex CRISPR-Cas9 vectors, each harboring 3 different guides targeting unique sequences of CYP735A3 and CYP735A4, were used to edit CYP735A3 and CYP735A4 (Supplemental Fig. S2; Supplemental Fig. S3). Two independent lines with mutations in both CYP735A3 and CYP735A4 were identified. In a T1 line, the cyp735a3-1 and cyp735a4-1 alleles were found as homozygote and heterozygote, respectively (Supplemental Fig. S2). In another T1 line, the cyp735a3-2 and cyp735a4-2 alleles were found as heterozygote and homozygote, respectively (Supplemental Fig. S3). The cyp735a3-1 single, cyp735a4-2 single, cyp735a3-1 cyp735a4-1 (a3a4-1) double and cyp735a3-2 cyp735a4-2 (a3a4-2) double mutants were isolated from progenies of these lines. The cyp735a3-1 and cyp735a3-2 are 1-base insertions that shift the reading frame and yield predicted proteins with no similarity to any non-redundant UniProtKB/SwissProt sequences and without the cytochrome P450 domain (Supplemental Fig. S4). The cyp735a4-1 and cyp735a4-2 mutants are 263- and 298-base deletions, respectively. The 263- and 298-base deletions resulted in a predicted protein lacking “A helix”, a secondary structure conserved among cytochrome P450s (Midlik et al. 2021), and an in-frame stop codon at 4 codons after the mutation, respectively (Supplemental Fig. S5). Since the proteins predicted to be produced in all the mutants (except for cyp735a4-1) are apparently inactive as cytochrome P450, we concluded that cyp735a3-1, cyp735a3-2, and cyp735a4-2 are null alleles.
CK concentrations in roots and shoots were measured in wild-type (WT) strain Nipponbare (NB), vector control (VC), and mutant strains cyp735a3-1, cyp735a4-2, a3a4-1, and a3a4-2 seedlings grown in hydroponic culture (Fig. 3; Supplemental Table S2; Supplemental Table S3). The concentrations of tZ-type CK and iP-type CK were comparable to WT in cyp735a3-1 (Fig. 3A). In contrast, cyp735a4-2 had slightly decreased tZ-type CK and slightly increased iP-type CK concentrations in roots and shoots as compared to the WT (Fig. 3B). In a3a4-1 and a3a4-2, the concentration of tZ-type CKs reduced dramatically to 3.5% to 8.8% of the wild-type levels in roots and shoots, while that of iP-type CK increased more than 2-fold. In the root of double mutants, the concentration of iP-type CKs increased to a level where the loss of tZ-type CK was compensated in terms of the sum of iP- and tZ-type CK quantity (iP-/tZ-type CK quantity). On the other hand, in the shoot, iP-type CK levels were increased but the iP-/tZ-type CK quantity was significantly lower compared with WT (Fig. 3; Supplemental Table S2; Supplemental Table S3). The cZ-type CK levels were not altered in any of the mutants (Fig. 3; Supplemental Table S2; Supplemental Table S3). The dihydrozeatin-type CK levels were reduced in a3a4-1 and a3a4-2 but only to 50% at the most of WT levels (Supplemental Table S2; Supplemental Table S3). Since the CK profiles of a3a4-1 and a3a4-2 are distorted in a similar manner, cyp735a4-1 also seems to be a null allele. These results show that tZ-type CKs are mostly synthesized by CYP735A3 and CYP735A4 in rice.
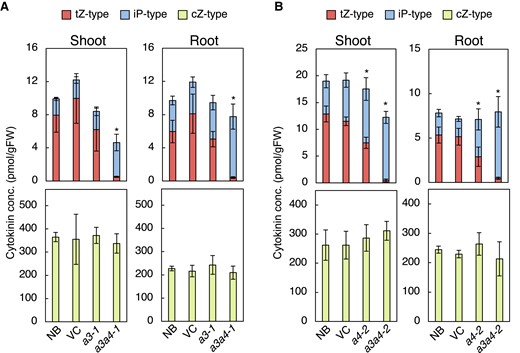
The cyp735a3 cyp735a4 double mutants show deficiency in trans-zeatin-type cytokinins. Cytokinin concentrations in roots and shoots of WT strain Nipponbare (NB), vector control (VC), and mutant strains cyp735a3-1 (a3-1), cyp735a4-2 (a4-2), cyp735a3-1 cyp735a4-1 (a3a4-1), and cyp735a3-2 cyp735a4-2 (a3a4-2). Seedlings were grown hydroponically for 14 d A) or 12 d B) before shoots and roots were harvested. Cytokinin levels were determined using an ultra-performance liquid chromatograph coupled with a tandem quadrupole mass spectrometer with an octadecylsilyl column and were normalized to the fresh weight of each sample. Error bars represent standard deviation of biological replicates (n = 3 to 6 independent pools of more than 10 plants for A, n = 6 to 8 independent pools of more than 10 plants for B). Asterisks indicate statistically significant differences in tZ-type cytokinin concentration compared with NB (P < 0.05, Dunnet's test). The concentration of each cytokinin molecular species in roots and shoots is shown in Supplemental Table S2 and Supplemental Table S3. gFW, gram fresh weight; tZ-type, trans-zeatin and its conjugates; cZ-type, cis-zeatin and its conjugates; iP-type, N6-(Δ2-isopentenyl)adenine and its conjugates.
Disruption of CYP735A3 and CYP735A4 retards growth of rice
To explore the physiological role of tZ-type CKs in rice, we examined the growth and development of cyp735a single and double mutants from seedling phase to maturation phases. Although cyp735a3-1 and cyp735a4-2 single mutants were indistinguishable from control plants (WT and VC) on our growth conditions, similar significant alterations in growth and development were observed in a3a4-1 and a3a4-2 double mutants (Fig. 4, Fig. 5, Supplemental Fig. S6).
![Disruption of CYP735As alters rice growth in seedling to vegetative phases. A) A representative image of Nipponbare (NB), vector control (VC), and cyp735a3-2 cyp735a4-2 (a3a4-2) seedlings grown 12 d in hydroponic culture. Scale bar, 5 cm. B) Quantification of shoot length, shoot fresh weight, root length, and root fresh weight in 12-d-old seedlings. NB, VC, cyp735a4-2 (a4-2), and a3a4-2 seedlings were grown in hydroponic culture. C) Leaf number at 15 d after germination D, E) Leaf sheath and leaf blade length D) and leaf width E) of the fully expanded third leaf of NB, VC, cyp735a3-1 (a3-1), cyp735a4-2 (a4-2), cyp735a3-1 cyp735a4-1 (a3a4-1), and a3a4-2 seedlings grown on soil. F) Quantification of leaf blade length, leaf sheath length, and leaf width of the fully expanded ninth leaf in NB, VC, a3a4-1, and a3a4-2 grown on soil. G) Tiller number of NB, VC, a3a4-1, and a3a4-2 grown on soil for 35 d. Error bars represent standard deviation of biological replicates [B), n = 6 to 8; C), n = 8 to 12; D), n = 8 to 10; E), n = 8 to 10; F), n = 8 to 12; G), n = 4 to 8]. Different lowercase letters indicate statistically significant differences as indicated by Tukey's HSD test (P < 0.05).](https://oup.silverchair-cdn.com/oup/backfile/Content_public/Journal/plphys/192/3/10.1093_plphys_kiad197/1/m_kiad197f4.jpeg?Expires=1750204116&Signature=iZiLKzGXwIlDXOKajCgH8Eh8m~WLvst1JS9jRcngmScIvch6~S328Pt0J0BZ~Ms0bHLWcTz0NSfy4ANgvDhVRw-Mk9YN5HA~Wck5Rk1JEGmlnw-oR7K8gFHYcpJSe48r0-O1CiGUcyIC6wVq1n8u-7pt-QuiQCn6F5sub3ewBGSD5SsQkgsRVTXYHAZlCgABNzFF-xEJo98Vj3iXgTSM-wyswLhGmEdG4WcyxgCISn6OrvPy14s0kjrR1-BFS4zMeBg7LC-jDXXyfmdN2e4pHoSSgcKQhPtzO4I5NV1s93h1zUWJ7SS07nwqcV6JCtIAqKfXqVTmgo5B4krgaqlGKQ__&Key-Pair-Id=APKAIE5G5CRDK6RD3PGA)
Disruption of CYP735As alters rice growth in seedling to vegetative phases. A) A representative image of Nipponbare (NB), vector control (VC), and cyp735a3-2 cyp735a4-2 (a3a4-2) seedlings grown 12 d in hydroponic culture. Scale bar, 5 cm. B) Quantification of shoot length, shoot fresh weight, root length, and root fresh weight in 12-d-old seedlings. NB, VC, cyp735a4-2 (a4-2), and a3a4-2 seedlings were grown in hydroponic culture. C) Leaf number at 15 d after germination D, E) Leaf sheath and leaf blade length D) and leaf width E) of the fully expanded third leaf of NB, VC, cyp735a3-1 (a3-1), cyp735a4-2 (a4-2), cyp735a3-1 cyp735a4-1 (a3a4-1), and a3a4-2 seedlings grown on soil. F) Quantification of leaf blade length, leaf sheath length, and leaf width of the fully expanded ninth leaf in NB, VC, a3a4-1, and a3a4-2 grown on soil. G) Tiller number of NB, VC, a3a4-1, and a3a4-2 grown on soil for 35 d. Error bars represent standard deviation of biological replicates [B), n = 6 to 8; C), n = 8 to 12; D), n = 8 to 10; E), n = 8 to 10; F), n = 8 to 12; G), n = 4 to 8]. Different lowercase letters indicate statistically significant differences as indicated by Tukey's HSD test (P < 0.05).
![Disruption of CYP735As alters rice growth in reproductive to maturation phases. A) A representative image of Nipponbare (NB), vector control (VC), and cyp735a3-1 (a3-1), cyp735a4-1 (a4-2), and cyp735a3-1 cyp735a4-1 (a3a4-2) plants grown 15 wk on soil. Scale bar, 20 cm. B to D) Heading date B), tiller number C), and panicle and culm length D) of NB, VC, a3-1, a4-2, cyp735a3-1 cyp735a4-1 (a3a4-1), and a3a4-2. E) A representative picture of mature panicles from NB and a3a4-2. Scale bar, 5 cm. F, G) Panicle weight on the main stem F) and number of primary branches per panicle G). Error bars represent standard deviation of biological replicates [B), n = 3 to 12; C), n = 5 to 12; D), n = 9 to 12; F), n = 5 to 10; G), n = 5 to 10]. Different lowercase letters indicate statistically significant differences as indicated by Tukey's HSD test (P < 0.05).](https://oup.silverchair-cdn.com/oup/backfile/Content_public/Journal/plphys/192/3/10.1093_plphys_kiad197/1/m_kiad197f5.jpeg?Expires=1750204116&Signature=eLo5Hdi6QCLAiFSdnKQ4t9Uvo0k6yWBt0VcPUXiI7VyW82OWaLDagcuXmk2T57uFJWl2s60oPAIZjZiIqJTZFNEHX3KeaF0JZLmhNWzm-KxxgDT6F33OZI-fcVGYc6T0uV5DBe4ny4cJPuszpPJsISKuJGB44GoDsE767XIdNsD4w0wvWbkdBEvtqHDjPkVOnyaLXPTO5-WyQXig32R5a0HVvEd31YK6UQms-UckffTr2C6mxBnobxQ50yf5bxD1P~Qxy3PK6mkPBPqDthGwFaD1huQhdkcbCvP2oeIGfk7CTAz5N~BjgRfXAdvEnd8tK964Aocj0IrGRyDE60g2eg__&Key-Pair-Id=APKAIE5G5CRDK6RD3PGA)
Disruption of CYP735As alters rice growth in reproductive to maturation phases. A) A representative image of Nipponbare (NB), vector control (VC), and cyp735a3-1 (a3-1), cyp735a4-1 (a4-2), and cyp735a3-1 cyp735a4-1 (a3a4-2) plants grown 15 wk on soil. Scale bar, 20 cm. B to D) Heading date B), tiller number C), and panicle and culm length D) of NB, VC, a3-1, a4-2, cyp735a3-1 cyp735a4-1 (a3a4-1), and a3a4-2. E) A representative picture of mature panicles from NB and a3a4-2. Scale bar, 5 cm. F, G) Panicle weight on the main stem F) and number of primary branches per panicle G). Error bars represent standard deviation of biological replicates [B), n = 3 to 12; C), n = 5 to 12; D), n = 9 to 12; F), n = 5 to 10; G), n = 5 to 10]. Different lowercase letters indicate statistically significant differences as indicated by Tukey's HSD test (P < 0.05).
The double mutant seedlings showed a decrease in the length and fresh weight of the shoot and root compared with control (Fig. 4, A and B; Supplemental Fig. S6, A and B). To determine the cause of the decrease in shoot length and fresh weight, leaf number and leaf size were measured (Fig. 4, C to E). Leaf number of double mutant seedlings at 15 d after germination was reduced compared to control (Fig. 4C), indicating that the rate of leaf formation is slower in the double mutant. Leaf blade and sheath length of fully expanded third leaf were similar for the double mutant and control (Fig. 4D), while leaf blade width was decreased in the double mutant (Fig. 4E). These results indicated that a decrease in leaf number accounts for the reduction in shoot length and a decrease in leaf number and leaf width contributes to the reduction in shoot fresh weight. Reduced shoot length phenotype of the double mutant continued into later vegetative growth stage (Supplemental Fig. S6, C and D), but it cannot be attributed only to a decrease in leaf number because leaf size, namely blade length, blade width, and sheath length, was significantly reduced at this stage as observed in the fully expanded ninth leaf (Fig. 4F). In addition, the double mutant developed significantly fewer tillers compared with control (Fig. 4G; Fig. 5C), suggesting that the activities of shoot apical and axillary meristems are impaired.
All control plants headed about 83 d after germination (n = 19) but double mutant plants either died without heading from the main stem (16 out of 24 plants) or showed delayed heading (8 out of 24 plants) (Fig. 5, A and B). Panicle length, panicle weight, and number of primary panicle branch were all significantly reduced in the double mutant compared to the control (Fig. 5, D to G), suggesting that inflorescence meristem activity is reduced. The double mutant set few but viable seeds, implying that reproductive organ development is not severely affected. Together, these results suggest that the tZ-type CKs regulate both root and shoot growth, most possibly through controlling meristem activity.
To examine whether the growth reduction was caused by a decrease in the level of tZ-type CKs, we fed a3a4 with tZ by spraying. As shown in Supplemental Fig. S7, application of tZ at 20 mM to a3a4 completely restored the shoot length and fresh weight of 14-d-old seedlings. On the other hand, iP application at the same concentration did not rescue the phenotype at all (Supplemental Fig. S7), demonstrating that the a3a4 phenotype is caused by a deficiency of tZ-type CKs.
The cytokinin activity is reduced both in roots and shoots of the cyp735a3 cyp735a4 mutant
The growth defects observed in a3a4 double mutant are reminiscent of those observed in rice mutants with diminished CK activities such as type-A RESPONSE REGULATOR (RR) OsRR6 overexpressing lines (Hirose et al. 2007), type-B RR triple mutant rr21/22/23 (Worthen et al. 2019), and CK receptor HISTIDINE KINASE (HK) double mutant hk5 hk6 (Burr et al. 2021). To examine whether the CK activity is reduced in the double mutant, we analyzed the expression levels of immediate-early CK-inducible type-A RRs by RT-qPCR (Fig. 6). The expression level of all tested type-A RRs, except for that of OsRR1 in roots, was significantly lower in the mutants compared with WT in both roots and shoots (Fig. 6A, B). On the other hand, the expression of non-CK-inducible type-B RRs, OsRR21 and OsRR23, was not reduced (Fig. 6, C and D). Thus, CK activity in roots and shoots of a3a4 is diminished, suggesting that the a3a4 growth phenotype is caused by decreased CK activity.
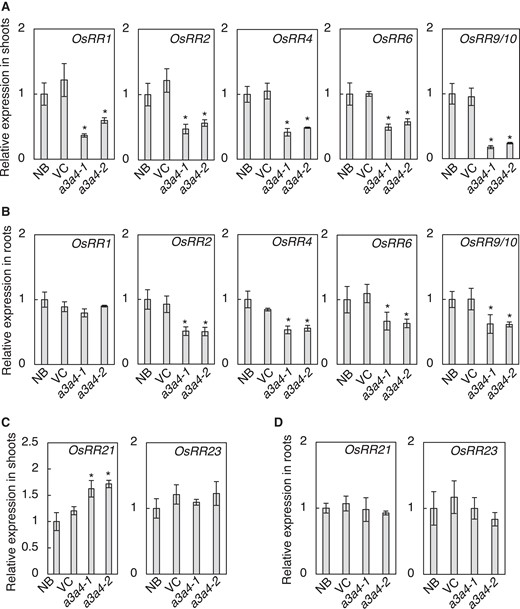
Expression of cytokinin inducible type-A response regulator genes is altered in the cyp735a3 cyp735a4 mutant. A, B) Transcript levels of cytokinin inducible type-A response regulator genes, OsRR1, OsRR2, OsRR4, OsRR6, and OsRR9/10, in shoots A) and roots B) of Nipponbare (NB), vector control (VC), cyp735a3-1 cyp735a4-1 (a3a4-1), and cyp735a3-2 cyp735a4-2 (a3a4-2) seedlings. OsRR9/10 indicates that the primer set used could not distinguish OsRR9 and OsRR10. C, D) Transcript levels of type-B response regulator genes, OsRR21 and OsRR23, in the shoot C) and in the root D) of NB, VC, a3a4-1, and a3a4-2 seedlings. Seedlings were grown hydroponically for 10 d before roots and shoots were harvested. Expression levels were quantified by RT-qPCR analysis, normalized to ZIURP1 as an internal control, and are shown relative to the value of NB. Error bars represent standard deviation of biological replicates (n = 4 independent pools of 2 plants). Asterisks indicate statistically significant differences compared to NB (P < 0.05, Dunnet's t-test).
Regulation of CYP735A3 and CYP735A4 expression by phytohormones
To obtain insights into the physiological relevance of tZ-type CK biosynthesis in rice, we analyzed the responses of CYP735As to phytohormones. In Arabidopsis, the expression of CYP735A1 and CYP735A2 was shown to be upregulated by CK and downregulated by auxin and abscisic acid (Takei et al. 2004). Tsai et al. (2012) analyzed the effect of these phytohormones on CYP735A3 and CYP735A4 expression by the NanoString nCounter system but could not detect CYP735A3 under some conditions using this system. To further characterize the response of CYP735As to these phytohormones in rice, we analyzed their expression in roots and shoots by RT-qPCR. Nipponbare seedlings treated with phytohormones were used for the analysis. OsRR4/OsRR6 (Kudo et al. 2012; Tsai et al. 2012), Oryza sativa SALT- INDUCED PP2C PROTEIN 1 (OsSIPP2C1) (Li et al. 2013), and Oryza sativa GRETCHEN HAGEN 3.2 (OsGH3.2) (Du et al. 2012) were used as positive controls for CK, IAA, and ABA responses, respectively. We found that transcript levels of CYP735A3 and CYP735A4 in roots were decreased by CK (iP and tZ), IAA, and ABA (Fig. 7, A and C). Although CYP735A3 expression in the shoot of 12-d-old seedlings was too low to reliably evaluate the changes in the transcript level by our RT-qPCR system, we found that CYP735A4 expression in shoots was downregulated by CKs, IAA, and ABA (Fig. 7, B and D). Thus, rice CYP735As and Arabidopsis CYP735As are regulated similarly by IAA and ABA, but differently by CK.
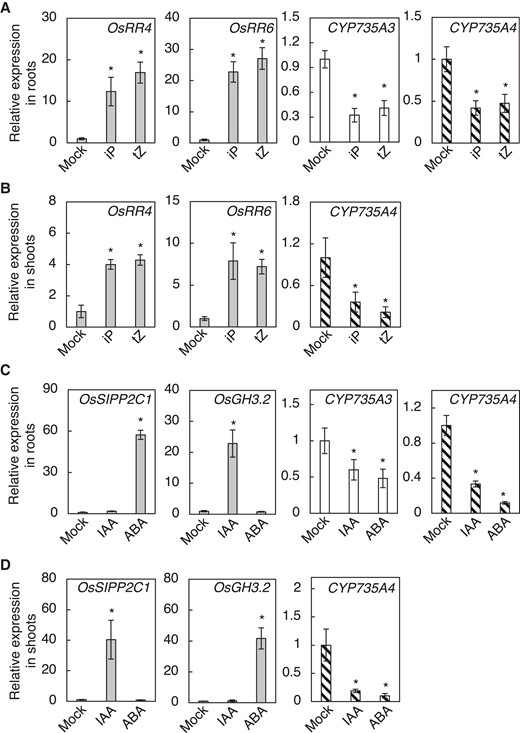
Expression of CYP735A3 and CYP735A4 in response to phytohormones. A, B) Nipponbare (NB) seedlings grown for 12 d were treated with 0.001% (v/v) DMSO (Mock), 1 µ M iP (iP), or 1 µ M tZ (tZ) and roots and shoots were harvested after 2 h. Relative expression levels of CYP735A3 and CYP735A4 in roots A) and CYP735A4 in shoots B) are shown. C, D) Expression of CYP735As in response to auxin (IAA) and abscisic acid (ABA). NB seedlings grown for 12 d were treated with 0.05% (v/v) DMSO (Mock), 10 µ M indole-3-acetic acid (IAA) or 50 µ M ABA and roots and shoots were harvested after 2 h. Relative expression levels of CYP735A3 and CYP735A4 in roots C) and CYP735A4 in shoots D) are shown. Expression levels were quantified by RT-qPCR analysis, normalized to ZIURP1 as an internal control, and are shown relative to the value in mock treatment. Asterisks indicate statistically significant differences compared with Mock (P < 0.05, Dunnet's test). Error bars represent standard deviation (n = 4 independent pools of more than 2 plants).
Regulation of CYP735A3 and CYP735A4 expression by nitrogen sources
Nitrogen nutrition is one of the major environmental factors regulating CK biosynthesis, including tZ-type CK biosynthesis, for plant growth optimization (Takei et al. 2001b; Kamada-Nobusada et al. 2013; Ohashi et al. 2017; Osugi et al. 2017; Maeda et al. 2018; Sakakibara 2021; Shibasaki et al. 2021; Kawai et al. 2022). In Arabidopsis, CYP735As are upregulated by the nitrate-specific signal and play a crucial role in nitrate-induced accumulation of tZ-type CKs (Engelsberger and Schulze 2012; Maeda et al. 2018). To test whether CYP735As of rice are nitrogen-inducible, we analyzed the expression levels of CYP735As in roots supplemented with ammonium or nitrate (Fig. 8). OsIPT4 and Oryza sativa NITRATE REDUCTASE 1 (OsNIA1) were used as indicators for glutamine-related and nitrate-specific responses, respectively (Kamada-Nobusada et al. 2013). CYP735A3 was induced by both ammonium and nitrate in a manner similar to OsIPT4, while the expression of CYP735A4 was upregulated only by nitrate like OsNIA1 (Fig. 8A). When proCYP735A3:GUS seedlings were supplemented with ammonium nitrate and GUS activity was detected in roots, an increase in the staining intensity was observed (Supplemental Fig. S8). To clarify whether CYP735As are regulated by glutamine-related and/or nitrate-specific signal, we examined the effect of methionine sulfoximine (MSX), an inhibitor of glutamine synthetase (Fig. 8B) (Lam et al. 1996). MSX pre-treatment inhibited the nitrate-triggered induction of CYP735A3 but did not affect CYP735A4. On the other hand, CYP735A3 was upregulated by glutamine supplement in the presence of MSX, while CYP735A4 was not. These responses of CYP735A3 and CYP735A4 to nitrogen sources and MSX are similar to those of OsIPT4 and OsNIA1, respectively, indicating that CYP735A3 and CYP735A4 are regulated by glutamine-related and nitrate-specific signals, respectively. Together, these results suggested that tZ-type CK biosynthesis in rice is controlled by dual nitrogen signals, which is different from that in Arabidopsis.
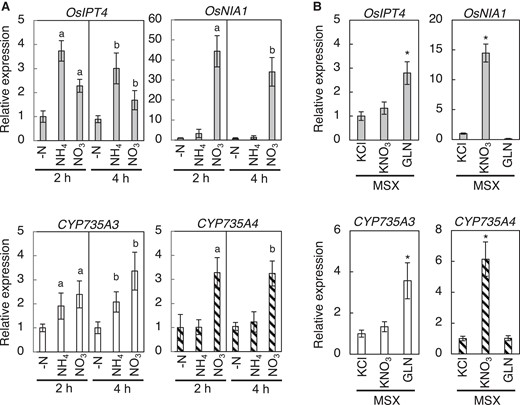
Expression of CYP735A3 and CYP735A4 in response to nitrogen sources. A) Expression of CYP735As in response to ammonium or nitrate supplement. Twelve-d-old NB seedlings hydroponically grown in nitrogen-free nutrient solution were incubated with 5 mM KCl (-N), 5 mM NH4Cl (NH4+), or 5 mM KNO3 (NO3-) for 2 h or 4 h. Expression levels were quantified by RT-qPCR analysis, normalized to ZIURP1 as an internal control, and are shown relative to the value in 2 h of -N treatment. Error bars represent standard deviation (n = 4 independent pools of 3 plants). Letters indicate statistically significant differences compared with –N of the same incubation time (a, 2 h; b, 4 h; P < 0.05, Dunnet's test). B) Effect of methionine sulfoximine on the induction of CYP735As by nitrogen. Twelve-d-old NB seedlings hydroponically grown in nitrogen-free nutrient solution were pre-treated with 2 mM MSX for 2 h and then incubated with 5 mM KCl, 5 mM KNO3, or 50 mM glutamine (GLN) for 2 h. Asterisks indicate statistically significant differences compared with KCl + MSX (P < 0.05, Dunnet's test). Expression levels were quantified by RT-qPCR analysis, normalized to ZIURP1 as an internal control, and are shown relative to the value in KCl treatment. Error bars represent standard deviation (n = 4 independent pools of 3 plants).
Discussion
Angiosperms can be divided into 4 main categories in terms of their CK side-chain profile and number of cotyledons: iP/tZ-type-abundant dicot, cZ-type-abundant dicot, iP/tZ-type-abundant monocot, and cZ-type-abundant monocot (Gajdošová et al. 2011). The function of some of these CKs has been demonstrated in a few dicotyledonous plant species. For example, in Arabidopsis, an iP/tZ-type-abundant dicot plant, CYP735As, catalyzes the biosynthesis of tZ-type CKs, which plays a specific role in shoot growth promotion (Kiba et al. 2013). Recently, it was suggested that CYP735A plays a similar role in Jatropha curcas, which is also an iP/tZ-type-abundant dicot plant (Cai et al. 2018). However, the function of CYP735A and tZ-type CKs in plants in other categories remains unknown. Here, we investigated the biosynthetic mechanism and function of tZ-type CKs in cZ-type-abundant monocot plant rice. Characterization of the a3a4 double mutant demonstrated that CYP735As play a central role in tZ-type CK biosynthesis in rice as in the iP/tZ-type-abundant dicot plants but that the physiological function of tZ-type CKs in rice is not the same as that in Arabidopsis, providing insights into the biological importance of side-chain variations in plants.
Arabidopsis CYP735As catalyze the trans-hydroxylation of iP-type CKs to form tZ-type CKs (Takei et al. 2004; Kiba et al. 2013). Complementation test of atcypDM and CK quantification of a3a4 demonstrated that CYP735A3 and CYP735A4 are the functional orthologs of Arabidopsis CYP735As (Fig. 1; Fig. 3; Supplemental Table S1; Supplemental Table S2; Supplemental Table S3). The tZ-type CKs in a3a4 was scarce but it did not completely disappear (Fig. 3; Supplemental Table S2; Supplemental Table S3). This is similar to what has been observed in the CYP735A-null mutants (atcypDM) of Arabidopsis and J. curcas (Kiba et al. 2013; Cai et al. 2018), suggesting the existence of minor alternative pathway(s) in both iP/tZ-type-abundant and cZ-type-abundant plants. The isomerization of cZ-type CKs is a possible pathway because enzymatic and/or non-enzymatic cis-to-trans isomerization activity has been detected in various plants, including rice (Bassil et al. 1993; Gajdošová et al. 2011; Kudo et al. 2012; Hluska et al. 2017). Although the isomerization pathway might be important under certain biological contexts, given that the pathway is minor even in the cZ-type-abundant rice, and that CYP735A homologs are found in all angiosperms whose genome is completely sequenced (Hansen et al. 2021), the CYP735A pathway seems to be the predominant pathway for tZ-type CK biosynthesis in angiosperms.
The a3a4 mutants displayed severe growth retardation in vegetative and maturation phases (Fig. 4; Fig. 5; Supplemental Fig. S5). We concluded that this phenotype is the result of diminished CK activity caused by a reduction in tZ-type CKs based on the following reasons: (i) the double mutant had severely reduced level of tZ-type CKs (Fig. 3; Supplemental Table S2; and Supplemental Table S3); (ii) the CK activity, measured by expression levels of immediate-early CK-inducible RR genes, was reduced in the double mutant (Fig. 6); (iii) external application of tZ recovered the growth defects of double mutant seedlings (Supplemental Fig. S7); (iv) the phenotypes of the double mutant are partly similar to those observed in rice mutants with severely reduced CK activity, such as OsRR6 overexpressors (Hirose et al. 2007), rr21/22/23 (Worthen et al. 2019) and hk5 hk6 (Burr et al. 2021); (v) our results were similar to the positive role of tZ-type CKs in rice growth suggested through the analysis of Oryza sativa ATP-BINDING CASSETTE G18 (OsABCG18), a transporter involved in root-to-shoot CK translocation (Zhao et al. 2019); (vi) a similar conclusion was drawn from the data obtained from equivalent experiments on CYP735A1 and CYP735A2 in Arabidopsis (Kiba et al. 2013).
The cZ-type CKs are the dominant variants in terms of quantity comprising more than 80% of total CK in various rice tissues and are suggested to be an active CK that plays a role in normal growth and development in rice (Kojima et al. 2009; Choi et al. 2012; Kudo et al. 2012; Osugi and Sakakibara 2015). Our CK quantification results showed that the levels of any of the cZ-type CK derivatives were not consistently altered in a3a4 (Fig. 3; Supplemental Table S2; Supplemental Table S3), indicating that cZ-type CKs are not directly relevant to the a3a4 phenotype. The dihydrozeatin-type CKs are proposed to be biosynthesized from tZ-type CKs through the activity of zeatin reductase (Martin et al. 1989). They are quantitatively minor variants in rice and their levels were only marginally affected in a3a4 compared with those of tZ-type CKs (Supplemental Table S2; Supplemental Table S3), suggesting that the role of dihydrozeatin-type CKs is minor, if any, in the development of a3a4 phenotypes. The iP-type CKs are considered to be an important variant in rice because of the identification of a receptor (OsHK6) with preferential affinity for iP (Choi et al. 2012). In a3a4, the iP-type CK levels were increased to compensate for the loss of tZ-type CK in the root but not sufficiently increased in the shoot (Fig. 3; Supplemental Table S2; Supplemental Table S3). From these results, it could be interpreted that the cause of the a3a4 phenotype is an alteration in CK side-chain profile (CK quality) but not CK quantity in the root and alteration in both CK quality and/or quantity in the shoot. Together, our results suggest that the function of tZ-type CKs is different from that of iP-type and cZ-type CKs at least in rice roots.
The phenotypes of a3a4 were observed both in roots and shoots, including reduced root proliferation, delayed plastochron, and decreased leaf size, panicle size, and tiller number (Fig. 4; Fig. 5; Supplemental Fig. S5), indicating the extensive role of tZ-type CKs as growth enhancers in various organs in rice. Although it is difficult to simply compare the phenotypes of a3a4 with those of atcypDM because monocot and dicot have remarkably different body plan, the major difference lies in the existence of phenotype in the root. The a3a4 displayed reduced CK activity and proliferation in the root (Fig. 4; Fig. 5; Supplemental Fig. S5), while no apparent difference was detected in atcypDM root (Kiba et al. 2013), suggesting that the role of tZ-type CKs in rice varies from that in Arabidopsis. The shoot-specific function of tZ-type CKs in Arabidopsis is partly explained by the ligand preference of CK receptors (Spíchal et al. 2004; Romanov et al. 2006; Stolz et al. 2011; Kiba et al. 2013). Among the 5 genes encoding CK receptors (OsHK3-6, CHARK) in rice, so far, the ligand preference of OsHK3, OsHK4, and OsHK6 has been investigated (Choi et al. 2012). However, no receptor with preferential affinity for tZ-type CKs has been identified and the mechanism underlying the functional specificity of tZ-type CKs in rice remains to be elucidated.
Consistent with the functional distinction of tZ-type CKs between rice and Arabidopsis, we also showed differences in responses to some internal and external cues of CYP735As (Fig. 2; Fig. 7; Fig. 8). Rice CYP735As are repressed by CKs (Tsai et al. 2012), while Arabidopsis CYP735As are induced (Takei et al. 2004), suggesting that rice and Arabidopsis have negative feedback and positive feedback regulation, respectively, on tZ-type CK function. The expression level of CYP735A4 is high in leaves (Fig. 2) but that of Arabidopsis CYP735As is not (Takei et al. 2004; Kiba et al. 2013). Responses of these genes to nitrogen supplement were also found to be different. CYP735A3 and CYP735A4 are upregulated by glutamine-related and nitrate-specific signals, respectively, in rice (Fig. 8) while CYP735As of Arabidopsis are induced only by nitrate-specific signal (Engelsberger and Schulze 2012; Maeda et al. 2018). Although ammonium is known to be the major form of inorganic nitrogen source in paddy field, rice utilizes not only ammonium but also nitrate generated by nitrification on the root surface as inorganic nitrogen sources (Kirk and Kronzucker 2005; Yan et al. 2011). For Arabidopsis, which grows on aerobic soil, nitrate is the major inorganic nitrogen source (Nacry et al. 2013), indicating that CYP735As of rice and Arabidopsis respond to nitrogen in a way relevant to the nitrogen source availability of the plants. Nitrogen-dependent tZ-type CK accumulation has been proposed to be involved in growth enhancement in response to nitrogen supply in various plants (Takei et al. 2001b; Kamada-Nobusada et al. 2013; Ohashi et al. 2017; Maeda et al. 2018; Sakakibara 2021; Shibasaki et al. 2021). Thus, the dual nitrogen-signal-dependent upregulation of tZ-type CK biosynthesis might play a similar role in rice. Consistent to that, there are reports suggesting that rice grows better with simultaneous supply of nitrate and ammonium than exclusive supply of nitrate or ammonium (Chanch and Ohira 1982; Ying-Hua et al. 2006). Together, these differences between rice and Arabidopsis might be the result of evolution and/or domestication to optimize growth depending on their body plan and growth environments, though further investigation into the physiological function of tZ-type CKs in other plant species is necessary to clarify this issue.
Materials and methods
Plant material and growth conditions
Arabidopsis (Arabidopsis thaliana) ecotype Columbia (Col-0) was used as the wild type. The cyp735a1-2 cyp735a2-2 double mutant was characterized previously (Kiba et al. 2013). Arabidopsis plants were grown on half-strength Murashige-Skoog (1/2x MS) agar plates with 1% (w/v) agar and 1% (w/v) sucrose placed vertically or on soil (Supermix A, Sakata, Japan) at 22 °C under fluorescent light (100 µmol m−2 s−1, 16 h light/8 h dark).
Rice (Oryza sativa) cultivar Nipponbare (NB) was used as the wild type. Rice seeds were germinated on moist filter paper at 30 °C for 2 to 3 d in the dark and then grown under hydroponic culture or soil culture conditions. For hydroponic culture, germinated seeds were sown on mesh trays floating on the nutrient solution (Makino et al. 1985) with or without nitrogen source (NH4NO3 was replaced with KCl) and grown in an environment-controlled greenhouse at 28 °C with a 15-h light (500 µmol m−2 s−1)/9-h dark cycle. The nutrient solution was renewed every 3 to 4 d. To analyze CYP735A3 and CYP735A4 expression in response to nitrogen, 12-d-old seedlings grown on the nutrient solution without nitrogen source were incubated with the nutrient solution without nitrogen source supplemented with KCl, NH4Cl, KNO3, or methionine sulfoximine (MSX). For soil culture, germinated seeds were sown on a synthetic soil (SunAgro, No. 3) and grown in an environment-controlled greenhouse at 28 °C with a 15-h light (500 µmol m−2 s−1)/9-h dark cycle. Fifteen-day-old seedlings were transplanted in perforated plastic pots (10 × 12 × 15 cm) filled with nutrient-free soil (Aichi Medel) supplemented with slow-release fertilizer (N, P, and K at 0.3, 0.3, and 0.3 g/kg soil, respectively) and grown in a greenhouse at Nagoya University with supplemental lighting (13-h light/11-h dark cycle).
Generation of transgenic lines overexpressing CYP735A3 or CYP735A4 in cyp735a1 cyp735a2
The CYP735A3 and CYP735A4 cDNA were amplified with the specific primer sets oxCYP735A3-F/R and oxCYP735A4-F/R, respectively (Supplemental Table S4). The amplified fragments were cloned into the Xba I/Sac I site of pBI121 (Clontech) located downstream of the cauliflower mosaic virus (CaMV) 35S promoter and introduced into cyp735a1-2 cyp735a2-2 double mutant by floral-dipping (Clough and Bent 1998).
CRISPR-Cas9 mutagenesis of CYP735A3 and CYP735A4
The mutants of CYP735A3 and CYP735A4 were generated using the transfer RNA-based-multiplex CRISPR-Cas9 vector, pMgPoef4_129-2A-GFP (Toda et al. 2019). Guide sequences for CYP735A3 and CYP735A4 were designed by CHOPCHOP (Labun et al. 2019) and 2 multiplex CRISPR-Cas9 vectors pMg129_A3A4-1 and pMg129_A3A4-2, each harboring 3 guide sequences for CYP735A3 and CYP735A4 sequences, were constructed as described (Toda et al. 2019). pMg129_A3A4-1 and pMg129_A3A4-2 contained guide sequences g3-1, g4-1-1, and g4-1-2 (Supplemental Fig. S1), and g3-2, g4-1-2, and g4-1-2 (Supplemental Fig. S2), respectively. Transgenic rice plants were generated by the Agrobacterium tumefaciens-mediated method (Hirose et al. 2005) using EHA105 strain harboring the vectors. Mutations were identified by DNA sequencing of PCR products amplified with specific primer sets, gCYP735A3-F2/R2 and gCYP735A4-F3R3 (Supplemental Table S4), and genomic DNA prepared from T1 plants. A T1 line transformed using pMg129_A3A4-2 but without any mutations in CYP735A3 and CYP735A was used as the vector control line. For assays, cyp735a3-1, cyp735a4-2, cyp735a3-1 cyp735a4-1, cyp735a3-2 cyp735a4-2, and vector control identified by genotyping progenies of these lines were used.
Quantification of plant hormones
CK levels were determined as described previously (Kojima et al. 2009; Kojima and Sakakibara 2012) using an ultra-performance liquid chromatograph coupled with a tandem quadrupole mass spectrometer (ACQUITY UPLC System/XEVO-TQS; Waters, Milford, MA, USA) with an octadecylsilyl (ODS) column (ACQUITY UPLC HSS T3, 1.8 µm, 2.1 mm × 100 mm, Waters). In the results, iP, iP-riboside, iP-riboside 5'-phosphates, iP-7-N-glucoside, and iP-9-N-glucoside are collectively referred to as “iP-type CK”; tZ, tZ-riboside, tZ-riboside 5'-phosphates, tZ-7-N-glucoside, tZ-9-N-glucoside, tZ-O-glucoside, and tZR-O-glucoside are collectively referred to as “tZ-type CK”; cZ, cZ-riboside, cZ-riboside 5'-phosphates, cZ-O-glucoside, and cZR-O-glucoside are collectively referred to as “cZ-type CK”; dihydrozeatin, dihydrozeatin riboside, dihydrozeatin riboside 5'-phosphates, and dihydrozeatin-9-N-glucoside are collectively referred to as “dihydrozeatin-type CK.”
Promoter-GUS analysis
The CYP735A3 promoter (proCYP735A3; −3636 to +21 bp relative to the inferred initiation codon) and CYP735A4 promoter (proCYP735A4; −3623 to +21 bp) were amplified with PrimeSTAR GXL DNA polymerase (Takara) and the specific primer sets proCYP735A3-F/R and proCYP735A4-F/R, respectively (Supplemental Table S4). The fragments were cloned into pENTR/D-TOPO vector (Thermo Fisher), sequenced, and then integrated into the GATEWAY binary vector pCAMBIA-GW-GUS (Kudo et al. 2012) to generate pCAMBIA1390-proCYP735A3:GUS and pCAMBIA1390-proCYP735A4:GUS vectors. Transgenic rice plants were generated by the Agrobacterium tumefaciens-mediated method (Hirose et al. 2005) using EHA105 strain harboring the vectors. Histochemical analysis of GUS activity was conducted as described previously (Hirose et al. 2005; Kiba et al. 2018). Fresh and GUS-stained samples were fixed and then embedded in Technovit 7100 resin (Heraeus Kulzer) as instructed by the manufacturer. Sections were produced using a Leica RM2165 microtome (Leica) and observed under an Olympus BX51 microscope (Olympus).
Gene expression analysis
Total RNA was extracted from root and shoot samples using the RNeasy Plant Mini kit (QIAGEN) in combination with the RNase-Free DNase set (QIAGEN). Total RNA was used for first strand cDNA synthesis by the ReverTra Ace qPCR RT Master Mix (Toyobo). Reverse transcription quantitative PCR (RT-qPCR) was performed on a Quant Studio 3 Real-Time PCR system (Thermo Fisher) with the KAPA SYBR Fast qPCR kit (KAPA Biosystems) and gene specific primers (Supplemental Table S5). ZIURP1 (LOC_Os03g08010/Os03g0234200) was used as an internal control because this gene has been shown to be one of the most stably expressed genes in rice (Pabuayon et al. 2016). Primer sets are listed in Supplemental Table S4.
Complementation analysis
To test the ability of externally applied CKs to complement the cyp735a3 cyp735a4 phenotype, NB and cyp735a3-1 cyp735a4-1 mutant seeds were germinated on a moist filter paper at 30 °C for 2 to 3 d in the dark. Germinated seeds were then transferred to soil culture conditions where 0.01% (v/v) DMSO (Mock), 20 M iP (iP), or 20 M tZ (tZ) solution containing a spreading agent at 0.003% (v/v) (Laviden3S, Nippon Soda) was sprayed on the shoot for 14 d daily.
Morphological analyses
The rosette diameter, shoot length, root length, leaf blade length, leaf sheath length, leaf width, panicle length, and culm length were measured manually or determined from pictures using ImageJ (https://imagej.nih.gov/ij/). The days leading to the appearance of a panicle in main stem are defined as “days to heading”.
Treatment with phytohormones and nitrogen sources
For phytohormone treatments, 12-d-old Nipponbare seedlings grown in hydroponic culture with the nutrient solution (Makino et al. 1985) containing 1 mM NH4NO3 were used. Phytohormone stock solutions were prepared by dissolving N6-(Δ2-isopentenyl)adenine (iP), trans-zeatin (tZ), indole-3-acetic acid (IAA), and abscisic acid (ABA) in dimethyl sulfoxide (DMSO) at 100 mM. Roots of the seedlings were submerged in the nutrient solution containing 1 µ M iP, 1 µ M tZ, 10 µ M IAA, 50 µ M ABA, 0.001% (v/v) DMSO (mock for iP and tZ treatment), or 0.05% (v/v) DMSO (mock for IAA and ABA treatment) and harvested after 2 h. For the treatment of shoots, 1 µ M iP, 1 µ M tZ, 10 µ M IAA, 50 µ M ABA, 0.001% (v/v) DMSO (mock for iP and tZ treatment), or 0.05% (v/v) DMSO (mock for IAA and ABA treatment) solution was sprayed on the shoot and the shoots were harvested after 2 h.
For nitrogen treatments, Nipponbare seedlings were grown in hydroponic culture with the nitrogen-free nutrient solution (1 mM NH4NO3 was replaced with 1 mM KCl) for 12 d. Roots of the seedlings were incubated with the nitrogen-free nutrient solution supplemented with 5 mM KCl, 5 mM NH4Cl, or 5 mM KNO3 for 2 h or 4 h. In case of MSX and nitrogen co-treatment, roots were pre-treated with the nitrogen-free nutrient solution containing 2 mM MSX for 2 h and then incubated with the MSX containing nitrogen-free nutrient solution supplemented with 5 mM KCl, 5 mM KNO3 or 50 mM glutamine for 2 h. For nitrogen treatment of proCYP735A3:GUS transgenic seedlings, seedlings grown in nitrogen-free nutrient solution for 16 d were incubated with the nutrient solution supplemented with 5 mM ammonium nitrate for 24 h. Crown roots were harvested from each seedling before and 24 h after ammonium nitrate supplementation and were subjected to GUS staining.
Phylogenetic analyses
Full-length amino acid sequences were obtained from Plant P450 database (https://erda.dk/public/vgrid/PlantP450/index.html). A phylogenetic tree was inferred by the neighbor-joining method using MEGAX (https://www.megasoftware.net/). The tree is drawn to scale, with branch lengths in the same units as those of the evolutionary distances used to infer the phylogenetic tree. The evolutionary distances were computed using the JTT matrix-based method and are in the units of the number of amino acid substitutions per site. All positions containing gaps and missing data were eliminated.
Statistical analysis
The experiments were independently performed at least twice. Data are shown as means ± standard deviation (Sd) of 1 representative experiment. In order to examine whether hormone concentration, gene expression, or morphological data were significantly different between treatments, Student's t-test, Tukey's honestly significant difference (HSD) test or Dunnet's test were performed using KaleidaGraph ver. 4.1 software (Synergy Software).
Accession numbers
Sequence data for the genes described in this article can be found in The Arabidopsis Information Resource database (http://www.arabidopsis.org) and Oryzabase (https://shigen.nig.ac.jp/rice/oryzabase/) under the following accession numbers: CYP735A1 (At5g38450), CYP735A2 (At1g67110), ACT8 (AT1G49240), CYP735A3 (LOC_Os08g33300/Os08g0429800), CYP735A4 (LOC_Os09g23820/Os09g0403300), ZIURP1 (LOC_Os03g08010/Os03g0234200), OsRR1 (LOC_Os04g36070/Os04g0442300), OsRR2 (LOC_Os02g35180/Os02g0557800), OsRR4 (LOC_Os01g72330/Os01g0952500), OsRR6 (LOC_Os04g57720/Os04g0673300), OsRR9 (LOC_Os11g04720/Os11g0143300), OsRR10 (LOC_Os12g04500/Os12g0139400), OsRR21 (LOC_Os03g12350/Os03g0224200), OsRR23 (LOC_Os02g55320/Os02g0796500), OsSIPP2C1 (LOC_Os09g15670/Os09g0325700), OsGH3.2 (LOC_Os01g55940/Os01g0764800), OsIPT4 (LOC_Os03g59570/Os03g0810100), and OsNIA1 (LOC_Os08g36480/Os08g0468100).
Author contributions
T.K. and H.S. designed the experiments; T.K., K.M., A.N., Y.T., M.K., T.H., Y.O., K.O., and H.S. performed the experiments, T.K., K.M., and A.N. analyzed the data, and T.K. and H.S. wrote the paper.
Supplemental data
The following materials are available in the online version of this article.
Supplemental Figure S1. Phylogenetic tree of Arabidopsis cytochrome P450s, CYP735A3, and CYP735A4.
Supplemental Figure S2. The cyp735a3-1 cyp735a4-1 mutant generated by the CRISPR/Cas9 system.
Supplemental Figure S3. The cyp735a3-2 cyp735a4-2 mutant generated by CRISPR/Cas9 system.
Supplemental Figure S4. Deduced amino acid sequences of CYP735A3, cyp735a3-1, and cyp735a3-2.
Supplemental Figure S5. Deduced amino acid sequences of CYP735A4, cyp735a4-1, and cyp735a4-2.
Supplemental Figure S6. Effect of disruption of CYP735As on vegetative growth.
Supplemental Figure S7. External application of tZ rescues the growth defect of cyp735a3 cyp735a4 seedlings.
Supplemental Figure S8. A representative image of GUS staining obtained from a proCYP735A3:GUS transgenic seedling before and after ammonium nitrate supplementation.
Supplemental Table S1. Cytokinin concentrations in Col-0, cyp735a1 cyp735a2 mutant, and transgenic plants constitutively expressing CYP735A3 (A3-ox) or CYP735A4 (A4-ox) in cyp735a1 cyp735a2 background.
Supplemental Table S2. Cytokinin concentrations in Nipponbare, vector control, cyp735a3-1 single mutant, and cyp735a3-1 cyp735a4-1 double mutant.
Supplemental Table S3. Cytokinin concentrations in Nipponbare, vector control, cyp735a4-2 single mutant, and cyp735a3-2 cyp735a4-2 double mutant.
Supplemental Table S4. List of primers used for vector construction and genotyping.
Supplemental Table S5. List of primers used for quantitative RT-PCR analysis.
Funding
This work was supported by KAKENHI from Japan Society for the Promotion of Science (No. JP18H04793 and JP20H02888 for T.K., JP17H06473 for H.S.).
References
Author notes
The author responsible for distribution of materials integral to the findings presented in this article in accordance with the policy described in the Instructions for Authors (https://dbpia.nl.go.kr/plphys/pages/General-Instructions) is Takatoshi Kiba ([email protected]).
Conflict of interest statement. None declared.