-
PDF
- Split View
-
Views
-
Cite
Cite
Zhengzheng Cai, Gang Wang, Jieqiong Li, Lan Kong, Weiqi Tang, Xuequn Chen, Xiaojie Qu, Chenchen Lin, Yulin Peng, Yang Liu, Zhanlin Deng, Yanfang Ye, Weiren Wu, Yuanlin Duan, Thermo-Sensitive Spikelet Defects 1 acclimatizes rice spikelet initiation and development to high temperature, Plant Physiology, Volume 191, Issue 3, March 2023, Pages 1684–1701, https://doi.org/10.1093/plphys/kiac576
- Share Icon Share
Abstract
Crop reproductive development is vulnerable to heat stress, and the genetic modulation of thermotolerance during the reproductive phase, especially the early stage, remains poorly understood. We isolated a Poaceae-specific FAR-RED ELONGATED HYPOCOTYLS3 (FHY3)/FAR-RED IMPAIRED RESPONSE1 (FAR1)family transcription factor, Thermo-sensitive Spikelet Defects 1 (TSD1), derived from transposase in rice (Oryza sativa) TSD1 was highly expressed in spikelets, induced by heat, and specifically enhanced the thermotolerance of spikelet morphogenesis. Disrupting TSD1 did not affect vegetative growth but markedly retarded spikelet initiation and development, as well as caused varying degrees of spikelet degeneration, depending on the temperature. Most tsd1 spikelets were normal at low temperature but gradually degenerated as temperature increased, and all disappeared at high temperature, leading to naked branches. TSD1 directly promoted the transcription of YABBY1 and YABBY3 and could physically interact with YABBY1 and three TOB proteins, YABBY5, YABBY4, and YABBY3. These YABBY proteins can form either homodimers or heterodimers and play an important role in spikelet morphogenesis, similar to TSD1. Notably, the knockout mutant yab5-ko and double mutant tsd1 yab5-ko resembled tsd1 in spikelet appearance and response to temperature, indicating that these genes likely participate in spikelet development through the cooperative TSD1–YABBY module. These findings reveal a distinctive function of FHY3/FAR1 family genes and a unique TSD1–YABBY complex to acclimate spikelet development to high temperature in rice, providing insight into the regulating pathway of enhancing thermotolerance in plant reproductive development.
Introduction
Temperature is a key factor governing the growth and development of the plant. High temperature has profound effects on crop production. It is estimated that every 1°C increase in the average daily temperature (ADT) may cause ∼14% decrease in crop yield (Wu et al., 2019). Plants have undergone long-term adaptation to ambient temperature and evolved multiple complex signaling pathways to sense temperature so as to adjust metabolism status and cell functions to alleviate or prevent heat damage (Chao et al., 2017). Upon exposure to high temperatures, plants sense the temperature change and initiate cellular and metabolic responses that enable them to adapt to the new environmental conditions (Li et al., 2018). So far, studies have revealed a number of vital cellular components and processes involved in thermoresponsive growth and acquisition of thermotolerance in plants (Li et al., 2018; Zhang et al., 2019a). Several key sensors/regulators associated with thermal adaptation have been identified in Arabidopsis (Arabidopsis thaliana) (Kumar and Wigge, 2010; Kumar et al., 2012; Jung et al., 2016), and factors for establishing and maintaining inflorescence architecture in response to temperature have been identified in Arabidopsis, maize (Zea mays ssp. maize), and barley (Hordeum vulgare) (Chao et al., 2017; Liu et al., 2019; Xie et al., 2020a; Li et al., 2021). However, none of them is involved in the specification of reproductive organs, which are usually more sensitive to high temperatures than vegetative tissues. Spikelet is the most vital organ in cereal crops, which directly determines grain yield but is vulnerable to heat. Studies have evaluated how rice plants respond to heat stress during the mid-late reproductive phase (Wu and Cui, 2014), but there is no conclusive evidence concerning heat stress on the reproductive development of early stages, especially the spikelet formation. The molecular modulation of thermotolerance in spikelets remains unknown.
YABBY family genes are plant-specific transcription factors, which exhibit diverse and unique roles. In Arabidopsis, YABBY genes are involved in the differentiation and patterning of leaves and the development of floral organs including carpel and ovules (Bowman and Smyth, 1999; Sawa et al., 1999; Villanueva et al., 1999; Sarojam et al., 2010; Zhang et al., 2020). These YABBY proteins can form either homodimers or heterodimers, which are involved in lateral organ formation and floral meristem (FM) termination via transcriptional repression or activation (Stahle et al., 2009). In rice, the YABBY family consists of eight members, five of which play important roles in reproductive development (Zhang et al., 2020). DROPPING LEAF (DL), an ortholog of CRC exhibits an important function in flower meristem determinacy and carpel specification (Yamaguchi et al., 2004; Ohmori et al., 2011; Sugiyama et al., 2019). YABBY1 plays an important role in meristem development and the maintenance of carpels and stamens (Jang et al., 2004; Dai et al., 2007). Three FILAMENTOUS FLOWER (FIL)/YAB3 orthologs of rice, TOB1/YABBY5, TOB2/YABBY4, and TOB3/YABBY3, are expressed in bract and lateral organ primordia. TOB1 is involved in lateral organ development and spikelet meristem activity maintenance. Disruption of TOB1 displays pleiotropic defects in spikelets (Tanaka et al., 2012). RNAi of either TOB2 or TOB3 alone does not result in perceptible abnormality in spikelets but can aggravate spikelet defects in tob1, and RNAi of both TOB2 and TOB3 in tob1 (TOB2-TOB3-RNAi; tob1) even expands the influence from spikelet to bract (Tanaka et al., 2017). Therefore, it is considered that the three TOB genes have redundant functions in floral organ identity determination and meristem activity maintenance.
FHY3/FAR1 family genes are also plant-specific transcription factors, which are derived from transposases (Hudson et al., 2003). Emerging evidence indicates that FHY3/FAR1 family genes regulate diverse physiological and developmental processes in Arabidopsis, such as light signal transduction and photomorphogenesis, shade avoidance response, circadian clock entrainment and flowering time regulation, chloroplast development, chlorophyll biosynthesis, ABA signaling, oxidative stress, and cell death, inositol biosynthesis, branching, plant architecture, and shoot and FM determinacy (Li et al., 2011, 2016; Stirnberg et al., 2012; Tang et al., 2013; Wang and Wang, 2015; Ma et al., 2016; Siddiqui et al., 2016; Wang et al., 2016; Ma et al., 2017; Ritter et al., 2017; Ma and Li, 2018; Zhang et al., 2019b; Liu et al., 2020; Xie et al., 2020b). Although some functions of FHY3/FAR1 have been identified, it is found that FHY3/FAR1 plays more roles than expected; meanwhile, the functions of most FHY3/FAR1 members are still unclear. Phylogenetic analysis shows that FHY3/FAR1 members are widespread in angiosperms, and over 18,000 putative FHY3/FAR1 homologs have been predicted in diverse plant species (Chae et al., 2021), indicating that FHY3/FAR1 members are evolutionarily conserved in angiosperms. However, their functions remain elusive outside Arabidopsis, and hitherto only one homolog has been characterized in rice (Joly-Lopez et al., 2016; Ma and Li, 2018; Chae et al., 2021).
In this study, we characterized a Poaceae-specific transposase-derived transcription factor gene TSD1 in rice. We found that TSD1 played a critical and specific role in maintaining the normal initiation and development of spikelets at high temperatures. Loss of TSD1 function caused thermo-sensitive defects in spikelets. TSD1 directly activated the transcription of YABBY1 and YABBY3 and physically interacted with YABBY1, YABBY3, YABBY4, and YABBY5. In addition, disrupting YABBY5 also resulted in thermo-sensitive spikelet defects closely resembling tsd1. These findings reveal a unique TSD1/YABBY module for rice to adapt to high temperatures during spikelet formation, and also provide an insight into the spikelet thermomorphogenesis in cereals.
Results
Tsd1 exhibits pleiotropic defects in spikelets
We identified a recessive mutant tsd1 from the progeny of indica rice cultivar MH86 mutagenized by irradiation. tsd1 exhibited normal vegetative growth but noticeably defects in spikelets when growing in the paddy field and flowering in late June (ADT being ∼27°C) in Fuzhou, China (Figure 1A). Most tsd1 spikelets showed curved lemma/palea with varying degrees of size reduction (Figure 1B). A wild-type floret was comprised of one lemma/palea, two lodicules, six stamens, and one carpel (Figure 1C). According to the phenotypes of tsd1 spikelets, they could be roughly divided into five classes (the detailed quantitative information is also shown in Figure 8S). (1) Normal organs inside lemma/palea (∼8%); (2) reduced number and maldevelopment of sex organs (∼60%); (3) without organs inside lemma/palea (∼14%); (4) without floret (∼10%); and (5) formation of ectopic organs (∼8%).
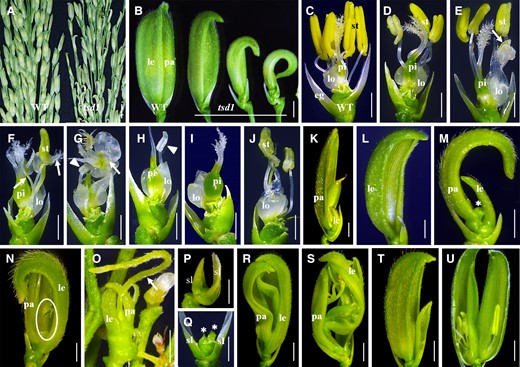
Phenotypes of spikelets in the tsd1 mutant. A, Wild-type and tsd1 panicles. B, Wild-type and tsd1 spikelets. Most tsd1 spikelets showed a reduced size of lemma/palea. C, A wild-type spikelet with lemma/palea removed. D–J, tsd1 spikelets (class II) with lemma and palea removed. Stamen and/or pistil showed reduced numbers and maldevelopment. Arrows in (E–G) indicate an unidentified organ or a chimera tissue; arrowheads in (G, H) indicate a maldeveloped stamen. K, A tsd1 spikelet producing seed. L–O, tsd1 spikelets (class III). The inner organs inside the lemma/palea were almost or thoroughly lost. The asterisk in (M) indicates a short cylinder-like structure; the ellipse in (N) and the arrow in (O) indicate a filamentous structure formed inside the lemma/palea. P, Q, tsd1 spikelets (class IV) without organs inside the sterile lemmas. The asterisks in (Q) indicate the inhibited meristem-like structure. R–U, tsd1 spikelets (class V) lacking the organs of the inner three whorls, replaced with ectopic lemma/palea-like organs or a panicle-like structure (R, S), or containing two florets (T, U). WT, wild-type; eg, extra glume; le, lemma; lo, lodicule; pa, palea; pi, pistil; st, stamen; sl, sterile lemma; bars = 1 cm in (A) and 2 mm in (B–U).
In class II spikelets, sex organs showed partial or complete degeneration (Figure 1, D–J). The number of stamens was variably reduced among spikelets (Figure 1, D–F, J), and even no stamen was formed (Figure 1I). Some spikelets generated chimeric or unidentified organs (Figure 1, E–G) and anther-maldeveloped stamens (Figure 1, G and H). Most class II spikelets produced a pistil in whorl 4, but a part of them was likely to be sterile due to abnormal appearance, such as with multiple stigmas (Figure 1F) or without stigma (Figure 1, G and H). Besides, a few spikelets showed a shortage of a pistil (Figure 1J). Altogether, most of the class II spikelets showed serious sex organ defects and therefore lost developmental function, but a few spikelets still maintained fertility and set seeds with good viability (Figure 1K). In class III spikelets, organs inside lemma/palea were almost or thoroughly lost (Figure 1, L–O). In severe cases, mutant spikelets lacked all inner organs and palea (Figure 1L). Sometimes, a short cylinder-like structure developed in the central zone (Figure 1M), and a filamentous structure formed just inside the lemma/palea in a few spikelets (Figure 1, N and O), which probably directly developed from the meristem. Class IV spikelets did not contain a floret (Figure 1, P and Q). They lost all the floral organs inside the sterile lemma (Figure 1P) except that one or two arrested meristem-like structures formed occasionally instead (Figure 1Q). Class V spikelets lost all the organs inside the lemma/palea, which were replaced with some ectopic glume-like organs and inflorescence-like structures (Figure 1, R and S), or generation two florets within the same spikelet (Figure 1, T and U). The palea was transformed into lemma-like organs, in which the palea membranous margin tissue was replaced with lemma-like tissue (Figure 1, R and S). Most of these twin florets were of agenesis and could not produce seeds (Figure 1, T and U). Occasionally, two unidentified structures instead of two florets formed within a single spikelet.
Taken together, disruption of TSD1 altered the size, shape, number, and location of floral organs, or even made the whole floret lost, suggesting that TSD1 is essential for spikelet specification.
TSD1 is required for FM activity maintenance and lateral primordia elaboration
To investigate the abnormalities of tsd1 spikelets in detail, we examined early-stage spikelets of the mutant by SEM. In the wild-type, lemma/palea developed beside the meristem in synchronized growth and alternate manner, and the dome-shaped primordia of stamen and pistil originated inside the lemma/palea (Figure 2A). As expected, in developing tsd1 spikelets, the floral organ primordium origination was substantially suppressed, leading to a noticeable reduction in both number and size or even loss of floral organs (Figure 2, B–J). As for stamen primordia, the number and the size were both variably reduced, the appearances were obviously irregular (Figure 2, B–E), and sometimes even thoroughly lost (Figure 2, F and G). Similar alterations were observed in the pistil primordium. The FM in the presumptive pistil primordium showed a flatter appearance in tsd1 (Figure 2, D and E), which differed considerably from the dome shape in wild-type (Figure 2A). In some spikelets, the FMs were prematurely terminated without forming primordium after the initiation of lemma/palea primordia (Figure 2, H–J). These observations confirmed that sex organ primordia were substantially suppressed in various degrees or thoroughly blocked in tsd1 spikelet. Functional alternation of FM was observed in tsd1 spikelet as well. Some tsd1 spikelets originated a variable number of protrusions with irregular shapes in the FM inside lemma/palea (Figure 2, K and L), indicating that the FM identity was lost and would develop into several lemma-like organs or spikelet-like organs consisting of multiple lemma-like organs (Figures 1R and 2M). We also found that two discrete meristem-like domes existed within the same tsd1 spikelet (Figure 2N), which were likely to be the primordia of two florets (Figure 2O) and would develop into a pair of twin florets in a spikelet (Figure 1S).
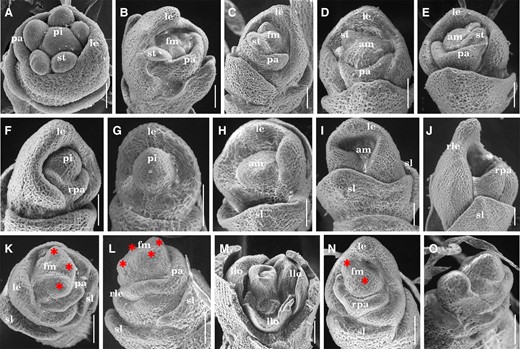
Spikelets at early developmental stages in the wild-type and tsd1 mutant. A, A wild-type spikelet at the stage of stamen and pistil initiation. B–O, tsd1 spikelets. B, C, tsd1 spikelets with several aberrant stamens. D, E, tsd1 spikelets with several aberrant stamens without pistil initiation. F, G, tsd1 spikelets without stamen primordia but a dome in the zone of presumptive pistil primordium. No palea was observed in (G). tsd1 spikelets without organ primordia inside the sterile lemmas (H, I) or lemmas/palea (J). K–L, tsd1 spikelets develop several irregular bumps (indicated by red asterisks) in the putative FM zone. M, tsd1 spikelets develop several lemma-like organs instead of sex organs. N, A tsd1 spikelet with two meristem-like domes (indicated by red asterisks). O, Two floret primordia in the same spikelet with lemma/palea removed. am, arrested meristem; fm, floral meristem; le, lemma; llo, lemma-liked organ; pa, palea; pi, pistil; rle, reduced lemma; rpa, reduced palea; sl, sterile lemma; st, stamen. Bars = 50 µm.
Collectively, SEM observations revealed that tsd1 pleiotropic spikelet phenotypes are due to misregulation of the FM or defects in the elaboration/growth of the lateral primordia that initiates from the FM, indicating that the activity and function of FM were disrupted or altered in tsd1 spikelet.
High temperature aggravates spikelet degeneration in tsd1
We noticed that the severity of defects in tsd1 spikelet changed with seasons in the paddy field in Fuzhou, China. The spikelet defect was moderate when heading in late June (Figure 1), but substantially aggravated in August and markedly relieved in November 2018 and 2019, indicating that the growth conditions had critical impacts on mutant spikelet development. No substantial morphological alteration was observed in tsd1 spikelets under short days and long days with different day-lengths but a constant ADT 27°C (Supplemental Figure 1), suggesting that photoperiod is not the influencing factor.
To assess the impact of ambient temperature on tsd1 spikelet, we cultivated mutant plants in a growth chamber under different temperatures at constant photoperiod (12 h light/12 h dark). As expected, the wild-type spikelets appeared normal at low or high temperatures (Figure 3, A and B, O, P), while tsd1 spikelets showed noticeable changes with the temperature (Figure 3C–N, Q–S; Table 1), .–Most tsd1 spikelets appeared normal at 22°C, with only a few spikelets showing slight defect on lemma/palea size (Figure 3, C and D), and/or generating 1∼2 chimeric organs instead of stamens (Figure 3E). At 27°C, however, over 70% tsd1 spikelets showed curved glumes (Figure 3F) and the organs inside lemma/pale showed defects in most spikelets similar to those growing in the paddy field and heading in June (Figure 1). When the temperature elevated to 31°C, the proportion of severely degenerated spikelets increased dramatically (Figure 3G). Most spikelets consisted of only reduced lemma/palea without inner organs (Figure 3, H–J). Occasionally, a filamentous structure was formed inside the rudimentary glumes (Figure 3K), or a branch-like structure was formed inside the glumes (Figure 3L). In addition, there were some spikelets, of which the development stagnated at the early stage or completely terminated (Figure 3M). As the temperature further increased to 34°C, most spikelets were completely terminated in development or degenerated except for a few severely defective spikelets forming in tsd1 panicle (Figure 3N). All spikelets were terminated to develop at 37°C, making the panicle look like a bundle of sticks or rods (Figure 3Q). A close-up view showed that the apical zones of the branches lacked meristem-like dome structures (Figure 3R), suggesting that the function and the maintenance of the spikelet meristem were profoundly compromised.
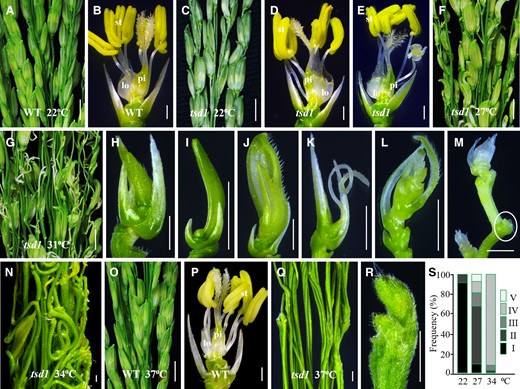
Thermo-sensitive degeneration of tsd1 spikelets. A, B, Wild-type (WT) panicle and spikelet at 22°C. C–E, tsd1 panicle and spikelets at 22°C. F, A tsd1 panicle at 27°C, in which most spikelets showed a curved appearance and defective organs inside glumes. G–M, tsd1 panicle and various severely defective spikelets at 31°C. Spikelets without organs inside defective lemma/palea in (H–J). A filamentous organ formed inside rudimentary glumes (K), and a branch-like structure formed inside glumes (L). Spikelets stagnate at an early stage or are completely terminated (indicated by a white ellipse) in development (M). N, A tsd1 panicle without spikelets except for a few defective ones at 34°C. O, P, Wild-type panicle and spikelet at 37°C. A tsd1 panicle with naked branches without spikelets (Q) and terminated spikelet origination (R) at 37°C. S, The proportions of various spikelet phenotypes in tsd1 at different temperatures. Lemma and palea were removed in (B, D, E, P). lo, lodicule; pi, pistil; st, stamen. Bars = 1 cm in (A, C, F, G, O), 1 mm in (B, D, E, H–M, P), and 200 µm in (N, Q, R).
Proportions of various tsd1 spikelet phenotypes at different temperatures in growth chambers
Type . | Temperature (°C) . | |||||
---|---|---|---|---|---|---|
22 . | 27 . | 31 . | 34 . | 37 . | ||
I | Normal sex organs | 462 (92.40%) | 46 (8.74%) | 14 (2.76%) | 0 | 0 |
II | Abnormal sex organs | 38 (7.60%) | 312 (59.32%) | 87 (17.09%) | 17 (3.31%) | 0 |
III | Loss of inner three whorls | 0 | 70 (13.31%) | 119 (23.37%) | 29 (5.64%) | 0 |
IV | Without floret | 0 | 56 (10.65%) | 159 (31.23%) | 41 (7.98%) | 0 |
Naked branch | 0 | 4 (0.76%) | 123 (24.17%) | 427 (83.07%) | 500 (100%) | |
V | Ectopic glumes | 0 | 17 (3.23%) | 4 (0.79%) | 0 | 0 |
Ectopic inflorescence | 0 | 9 (1.71%) | 3 (0.59%) | 0 | 0 | |
Ectopic florets | 0 | 12 (2.28%) | 0 | 0 | 0 | |
Total number examined | 500 | 526 | 509 | 514 | 500 |
Type . | Temperature (°C) . | |||||
---|---|---|---|---|---|---|
22 . | 27 . | 31 . | 34 . | 37 . | ||
I | Normal sex organs | 462 (92.40%) | 46 (8.74%) | 14 (2.76%) | 0 | 0 |
II | Abnormal sex organs | 38 (7.60%) | 312 (59.32%) | 87 (17.09%) | 17 (3.31%) | 0 |
III | Loss of inner three whorls | 0 | 70 (13.31%) | 119 (23.37%) | 29 (5.64%) | 0 |
IV | Without floret | 0 | 56 (10.65%) | 159 (31.23%) | 41 (7.98%) | 0 |
Naked branch | 0 | 4 (0.76%) | 123 (24.17%) | 427 (83.07%) | 500 (100%) | |
V | Ectopic glumes | 0 | 17 (3.23%) | 4 (0.79%) | 0 | 0 |
Ectopic inflorescence | 0 | 9 (1.71%) | 3 (0.59%) | 0 | 0 | |
Ectopic florets | 0 | 12 (2.28%) | 0 | 0 | 0 | |
Total number examined | 500 | 526 | 509 | 514 | 500 |
Proportions of various tsd1 spikelet phenotypes at different temperatures in growth chambers
Type . | Temperature (°C) . | |||||
---|---|---|---|---|---|---|
22 . | 27 . | 31 . | 34 . | 37 . | ||
I | Normal sex organs | 462 (92.40%) | 46 (8.74%) | 14 (2.76%) | 0 | 0 |
II | Abnormal sex organs | 38 (7.60%) | 312 (59.32%) | 87 (17.09%) | 17 (3.31%) | 0 |
III | Loss of inner three whorls | 0 | 70 (13.31%) | 119 (23.37%) | 29 (5.64%) | 0 |
IV | Without floret | 0 | 56 (10.65%) | 159 (31.23%) | 41 (7.98%) | 0 |
Naked branch | 0 | 4 (0.76%) | 123 (24.17%) | 427 (83.07%) | 500 (100%) | |
V | Ectopic glumes | 0 | 17 (3.23%) | 4 (0.79%) | 0 | 0 |
Ectopic inflorescence | 0 | 9 (1.71%) | 3 (0.59%) | 0 | 0 | |
Ectopic florets | 0 | 12 (2.28%) | 0 | 0 | 0 | |
Total number examined | 500 | 526 | 509 | 514 | 500 |
Type . | Temperature (°C) . | |||||
---|---|---|---|---|---|---|
22 . | 27 . | 31 . | 34 . | 37 . | ||
I | Normal sex organs | 462 (92.40%) | 46 (8.74%) | 14 (2.76%) | 0 | 0 |
II | Abnormal sex organs | 38 (7.60%) | 312 (59.32%) | 87 (17.09%) | 17 (3.31%) | 0 |
III | Loss of inner three whorls | 0 | 70 (13.31%) | 119 (23.37%) | 29 (5.64%) | 0 |
IV | Without floret | 0 | 56 (10.65%) | 159 (31.23%) | 41 (7.98%) | 0 |
Naked branch | 0 | 4 (0.76%) | 123 (24.17%) | 427 (83.07%) | 500 (100%) | |
V | Ectopic glumes | 0 | 17 (3.23%) | 4 (0.79%) | 0 | 0 |
Ectopic inflorescence | 0 | 9 (1.71%) | 3 (0.59%) | 0 | 0 | |
Ectopic florets | 0 | 12 (2.28%) | 0 | 0 | 0 | |
Total number examined | 500 | 526 | 509 | 514 | 500 |
Overall, ambient temperature rather than photoperiod affected tsd1 spikelet development. Most tsd1 spikelets could develop normally at low temperatures but became more and more defective as the temperature increased until no spikelets could be formed. This indicates a dosage-based rather than threshold-based mode of temperature effect on the development of tsd1 spikelets. These findings suggest that TSD1 is necessary to maintain spikelet origination and fate at high temperatures.
TSD1 encodes a transposase-derived transcription factor
Genetic analysis showed that tsd1 was controlled by a single gene. Based on an F2 population and using RM-series rice microsatellite markers, we roughly mapped TSD1 to the region between RM555 and RM424 on chromosome 2 (Figure 4A). To narrow down the interval, more markers were used, but no recombinant was found. To tackle this problem, we performed whole-genome resequencing of a pair of wild-type and mutant DNA pools. Based on the resequencing data, we found three breakpoints at about 4.66, 7.51, and 10.70 Mb, respectively, suggesting that a long segment from 4.66 to 10.70 Mb must have been deleted and broken into two fragments (one from 4.66 to 7.51 Mb and the other from 7.51 to 10.70 Mb) in the mutant. However, PCR analysis showed that the expected deletion did not occur. Subsequent TAIL-PCR (thermal asymmetric interlaced PCR) analysis indicated that the two fragments were reorganized by inversion and translocation (Figure 4A). This break-reorganization event destroyed Os02g0232500 and Os02g0284500 by breaking them into two separate parts, respectively (Figure 4B). No other mutation was detected within the mapped region. Therefore, the phenotype of tsd1 spikelet was likely caused by the function loss of either or both of these two genes. Os02g0232500 encodes a leucine-rich repeat-containing protein kinase and is expressed in developing panicles, but all 24 independent CRISPR/Cas9-induced transgenic lines showed normal spikelets (Supplemental Figure 2 and Table 1), suggesting that this gene is likely not TSD1.
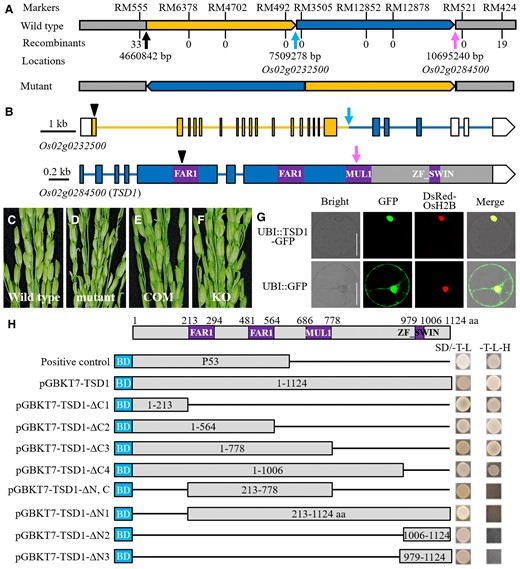
Identification of the TSD1 gene, which encodes a transposase-derived transcription factor. A, Fine mapping and structural analysis of the region between RM555 and RM424 on chromosome 2. The number of recombination events between a marker and TSD1 is shown under the marker. Inversion and translocation were found in the region from 4.66 to 10.70 Mb in the mutant, making Os02g0232500 (indicated by the blue arrow) and Os02g0284500 (indicated by the red arrow) broken into two separate parts, respectively. B, Schematic presentation and the breakpoints of both Os02g0232500 (indicated by the blue arrow) and Os02g0284500 (indicated by the pink arrow). Four conserved functional domains were also shown in Os02g0284500. Boxes indicate exon (white box, untranslated region); solid lines indicate introns. Black arrowheads indicate the editing sites of Cas9-induced knockout. C–F, Genetic complementation test of the tsd1 mutant and Cas9-induced knockout of TSD1 in the wild-type. The wild-type phenotype was recovered in the transgenic plants of the complementation test, while the tsd1 panicle phenotype appeared in all the transgenic plants of TSD1 knockouts. COM, complementation; KO, knockout. G, Subcellular localization of the TSD1-GFP fusion protein in a rice protoplast. The DsRed-OsH2B fusion protein was used as a nuclear localization marker, and the GFP protein alone was used as the control. Bars = 20 μm. H, Transcription activity assay of full-length or truncated TSD1 in yeast. The full-length and a series truncation of TSD1 were fused to the GAL4 BD of the pGBKT7 vector as bait and tested on a selective medium (SD/-Trp/-Leu/-His). The numbers in the boxes indicate the TSD1 amino acid residues used for construction.
Os02g0284500 encodes a transposase-derived protein with 1124 amino acids. Similar to Arabidopsis FRS7/12, the Os02g0284500 protein has four conserved domains (Figure 4B; Ma and Li, 2018). However, phylogenetic analysis indicated that it belonged to a different subgroup from that of FRS7/12 (Supplemental Figure 3). Mutation in tsd1 resulted in an unfunctional truncated protein of Os02g0284500 (Figure 4B), suggesting that it was likely a null allele. When the plasmid carrying a 6583-bp wild-type genomic DNA sequence was introduced into tsd1, the mutant phenotype was rescued in all nine complementary lines (Figure 4, C–E). In contrast, disrupting Os02g0284500 led to spikelet defects as observed in tsd1 in all seventeen independent transgenic lines (Figure 4F; Supplemental Table 2; Supplemental Figure 4). These results confirmed that Os02g0284500 is TSD1. TSD1 is the same gene as PANICLE AND SPIKELET DEGENERATION (PSD), which was mapped previously by Zhang et al. (2015).
Subcellular localization analysis of GFP-TSD1 fusion protein indicated that TSD1 is localized in the nucleus (Figure 4G). This is consistent with the prediction that TSD1 is a transcription factor. We also examined the transcriptional activation of TSD1 protein and showed that the TSD1 and GAL4 DNA-binding domain (BD) in the pGBKT7 vector fusion protein in yeast has transcriptional activity, indicating that TSD1 is a transcription factor. A truncation analysis revealed that the N-terminal 213 aa of TSD1 is required for its transcriptional activation (Figure 4H).
TSD1 has higher expression in reproductive organs and is up-regulated by high temperature
To investigate the spatio-temporal expression of TSD1, we created transgenic plants expressing the GUS gene driven by the promoter of TSD1 and examined GUS expression by histochemical staining. The results indicated that GUS activity was strong in inflorescence (Figure 5A) and young spikelet (Figure 5B). As the panicle developed, TSD1 expression gradually decreased (Figure 5, C–F). In mature spikelets, strong TSD1 expression was detected in all inner three whorls of organs (Figure 5G). In addition, TSD1 was also expressed in all vegetative tissues examined including young root (Figure 5H), culm (Figure 5I), and leaf blade (Figure 5J). To further characterize the tissue-specific expression of TSD1 in spikelets, we performed in situ hybridization experiments. The results indicated that TSD1 expression was initially high in FM (Figure 5K), as well as maintained high expression in the four whorls of floral organ primordia during floral organ origination (Figure 5, L–N). We also carried out RT-qPCR to analyze the transcription of TSD1. The results indicated that TSD1 was expressed in all tissues or organs examined, which is consistent with GUS activity detection. Overall, the TSD1 transcription levels in vegetative tissues were substantially lower than those in reproductive organs. The highest expression of TSD1 was detected in young panicles, and the expression was reduced as the panicle developed (Figure 5O).

Expression pattern of TSD1 in rice. A–J, TSD1 expression was revealed by GUS staining. GUS activity was detected in young panicles (A, B), developing spikelet (C–F), mature spikelet and floral organs (G), young root (H), culm (I), and leaf blade (J). Lemma, palea, and three stamens in (G) were removed. K–N, RNA in situ hybridization detection of TSD1 expression during the differentiation and development of floral organ primordia. Scale bars = 1 mm in (A–J), 50 µm in (K–N). TSD1 expression was detected by RT-qPCR analysis in different organs/tissues (O) and at high and low temperatures treatment in young leaf (P) and young panicle of three different development stages (Q). The RT-qPCR analysis was performed with three biological replicates. The error bars indicate standard deviation. Significant differences (**P < .01) were determined via the t test between the two temperatures. eg, extra glume; fm, floral meristem; le, lemma; lo, lodicule; pa, palea; pi (Pi), pistil; st, stamen; Gl, glume; L, leaf blade; R, root; S, stem; Sh, sheath; P1, P2, P3, P5 and P7, young panicles of 1, 2, 3, 5, and 7 cm in length. Bars = 50 µm.
To investigate the relationship of TSD1 expression with ambient temperature, we performed RT-qPCR to analyze TSD1 expression in young leaves and panicles from wild-type plants cultivated in a growth chamber at 22°C (low temperature) and 34°C (high temperature), respectively, with a photoperiod of 12 h light/dark alternation. The results of eight-hour successive monitoring indicated that TSD1 expression in young leaves was relatively stable at low temperatures, but substantially increased in two hours and maintained a stable high expression at high temperatures (Figure 5P). The TSD1 expression in young panicles of three different development stages displayed the same trend as that in young leaves (Figure 5Q). These results indicate that TSD1 expression is up-regulated by heat. This appears to be consistent with the temperature-dependent phenotype of tsd1 spikelet.
TSD1 directly regulates the transcription of YABBY1 and YABBY3
It is known that YABBY1 and the three TOB-type YABBY genes play an important role in FM maintenance and lateral organ development in rice. In particular, the abnormal spikelets of tob1 and triple mutant (TOB2-TOB3-RNAi; tob1) resemble those of tsd1 (Figure 1; Tanaka et al., 2012, 2017), indicating that these YABBY genes have a similar function to TSD1 in spikelet formation. We presumed that TSD1 might either regulate the expression or be involved in the same regulation pathway of these YABBY genes.
To investigate whether these YABBY genes are regulated by TSD1, we first used RT-qPCR to analyze their transcription in young panicles from tsd1 and wild-type plants. The result showed that TSD1 promoted the expression of YABBY1 and YABBY3, but had no significant effect on YABBY4 and YABBY5 (Figure 6A). We examined their genomic sequences and found that there are specific FHY3 binding sites (FBS, with motif CACGCGC; Li et al., 2016) in the promoters of OsYABBY1 and OsYABBY3 (Supplemental Figure 5), but not in those of YABBY4 and YABBY5. The ChIP-qPCR assay was performed on TSD1-GFP with an antibody to GFP. The results showed that each gene was significantly enriched in the promoter fragment containing the FBS site (Figure 6B). To validate the binding, we performed a yeast one-hybrid (Y1H) assay, which confirmed that TSD1 could bind to the promoter of both YABBY1 and YABBY3 containing FBS sites (Figure 6C). We also performed a transient expression assay in rice protoplasts to examine the effect of TSD1 on the transcription of YABBY1 and YABBY3. The promoter sequences of YABBY1 and YABBY3 were inserted into pGreenII 0800-LUC, and the coding sequence of TSD1 was inserted into pGreenII 62-SK. Compared with that of the control vector, the luciferase activity was significantly elevated when TSD1 cotransfected with the promoter of YABBY1 and YABBY3 (Figure 6D). Together, these observations indicated that TSD1 could directly bind to the promoters of YABBY1 and YABBY3 and positively regulate the expression of the two genes.
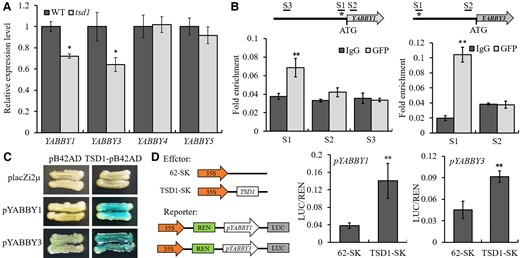
TSD1 binds to the promoters of YABBY1 and YABBY3 to promote their expression. A, Expression of four YABBY genes in wild-type and tsd1 young panicles. The RT-qPCR analysis was performed with three biological replicates. The error bars indicate standard deviation. The difference in relative expression level of each gene between the WT (set to be 1) and mutant was examined with a t test. *P < .05. B, ChIP assay of YABBY1 and YABBY3 in 10-day-old tsd1 seedlings grown under Normal white light conditions. Asterisk indicates the FBS. C, Y1H assay showing the binding of TSD1 to the promoters of YABBY1 and YABBY3. Weak self-activation was shown in YABBY3. D, LUC assay of luciferase activity showed that TSD1 directly enhanced the expression of YABBY1 and YABBY3 in rice protoplasts. The values given in (B, D) are mean ± Sd (n = 3). **P < .01 by t test.
TSD1 physically interacts with YABBY1, 3, 4, and 5, and these YABBYs form homo- and heterodimers
YABBY proteins may directly regulate the transcription of target genes or be involved in protein–protein interactions that modulate the functions of other proteins (Palermo and Dornelas, 2021). To investigate whether TSD1 interacts with the above four YABBY proteins, we performed a Y2H assay. The Y2H assay indicated that TSD1 interacted with the four YABBY proteins (Figure 7A). The (yeast two-hybrid) Y2H results were further confirmed by BiFC assay, in which TSD1 was fused to the N-terminal fragment (YN) of YFP, and four YABBY genes were fused to the C-terminal fragment (YC) of YFP, respectively. EYFP fluorescence was observed in the nuclei of rice protoplasts in the combined expression of TSD1-YN and four YABBY-YC with different YABBY genes, respectively (Figure 7B). However, no fluorescence was observed in the combined expression of negative controls (Supplemental Figure 6). The same results were obtained by luciferase complementation imaging (LCI) assays in leaves of Nicotiana benthamiana. The strong fluorescence signals were observed in the coexpression of TSD1-nLUC and four YABBY-cLUC with different YABBY genes, respectively, but not in the leaves of all negative controls (Figure 7C). These interactions were also confirmed by in vivo co-immunoprecipitation (Co-IP) assay (Figure 7D). These results strongly indicated that TSD1 physically interacts with the four YABBY proteins, respectively. To investigate whether these TSD1–YABBY complexes still maintained the transcriptional activity, we performed a transient expression assay in rice protoplasts to examine the effect of TSD1 + YABBY3 and TSD1 + YABBY3 on the transcription of YABBY1. The results showed that the two complexes maintained the similar transcriptional activity to TSD1 (Supplemental Figure 7).
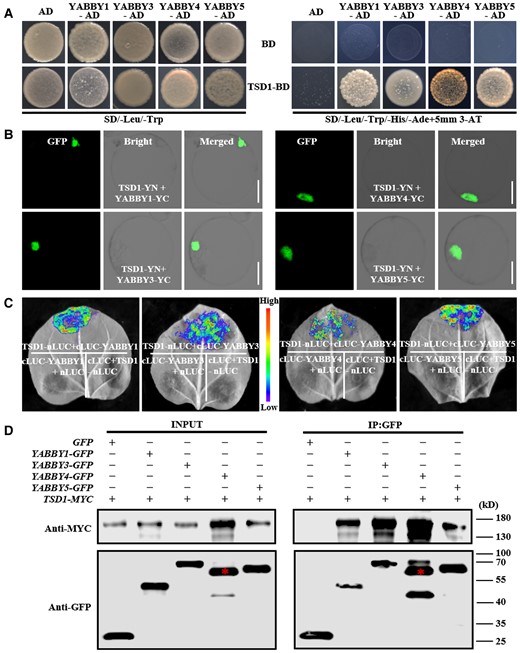
Identification of the interactions between TSD1 and four YABBY proteins. A, Y2H assay of interactions between TSD1 and the four YABBY proteins. TSD1 and four YABBY proteins showed no toxicity in SD selective medium (-Leu/-Trp) and could grow normally. The selection medium (-Trp/-Leu/-His/-Ade) contained 5 mM 3-AT (3-aminotriazole). B, BiFC analysis of interactions between TSD1 and the four YABBY proteins in rice protoplasts. C, LCI assay of interactions between TSD1 and the four YABBY proteins in N. benthamiana leaves. TSD1 was fused to nLUC and four YABBY genes were fused to cLUC. TSD1-nLUC and four YABBY-cLUC from different YABBY genes were used as control. D, Co-IP analysis of interactions between TSD1 and the four YABBY proteins; red asterisks indicate the target bands of YABBY4. Ad, pGADT7; BD, pGBKT7. Scale bars = 10 μm.
It is known that transcription factors with an HMG box or a zinc finger domain can form both homo- and heterodimers (Sanchez-Giraldo et al., 2015). YABBY proteins have both domains. Indeed, Arabidopsis YABBY proteins are able to form either homodimers or heterodimers with each other (Stahle et al., 2009). To investigate whether this is also the case for YABBY1, 3, 4, and 5, we performed the BiFC and LCI assay. The results confirmed that these four YABBY proteins can form homodimers and heterodimers (Supplemental Figures 6 and 8).
TSD1 and YABBY5 may regulate spikelet morphogenesis through the same genetic network
The YABBY proteins are plant-specific TFs, which exhibit diverse and unique roles in floral formation (Zhang et al., 2020; Palermo and Dornelas, 2021). In rice, the function of three TOB genes in spikelet formation has been characterized, in which TOB1/YABBY5 takes a more important role than the other two TOB genes. In particular, the abnormal spikelets of tob1 and their seasonal phenotypes were similar to those of tsd1 (Tanaka et al., 2017). To verify the roles of YABBY genes in the regulatory mechanism of TSD1, we employed CRISPR/Cas9 to create knockout plants of YABBY5 from MH86, the same genetic background as tsd1. A total of 37 nonfunctional transgenic lines were obtained, which can be divided into four alleles (Supplemental Table 3). These mutant alleles did not show substantial phenotypic differences among them when growing in the paddy field. So, we named them all yab5-ko.
To investigate the effect of temperature on the phenotypes of yab5-ko spikelets, we cultivated mutant plants in a growth chamber under three different temperatures at 12 h light/dark alternation. As expected, almost all spikelet defects in tsd1 were also observed in yab5-ko at 27°C, such as reduction of palea and/or lemma (Figure 8, A and B), formation of an ectopic floret (Figure 8C) or glumes (Figure 8D), alteration the size, shape, number, and location of floral organs inside lemma/palea, and generation maldeveloped or chimeric tissues, unidentified organs, or even complete loss of floret (Figure 8, E–L). The only exception was that yab5-ko produced a small number of cone-shaped glumes that are not observed in tsd1 (Figure 8M). Most yab5-ko spikelets showed a normal appearance and the floral organs inside lemma/palea were largely normal as well at 22°C (Figure 8, N and O). In contrast, when the temperature increased to 34°C, the development of most yab5-ko spikelets was terminated, giving rise to a few seriously degenerating spikelets (Figure 8, P–R). These results indicate that yab5-ko has the characteristics of thermo-sensitive spikelet defects and its spikelet degeneration can be aggravated by high temperature, similar to tsd1. Overall, yab5-ko was very similar to tsd1 in spikelet appearance and response to temperature, but it produced fewer defective spikelets with less severe mutant phenotypes than tsd1 at high temperatures (Figures 3S and 8S), probably due to the redundant functions of other, –ABBY genes in FM maintenance and floral organ identification, which has been confirmed in the three TOB genes (Tanaka et al., 2017).
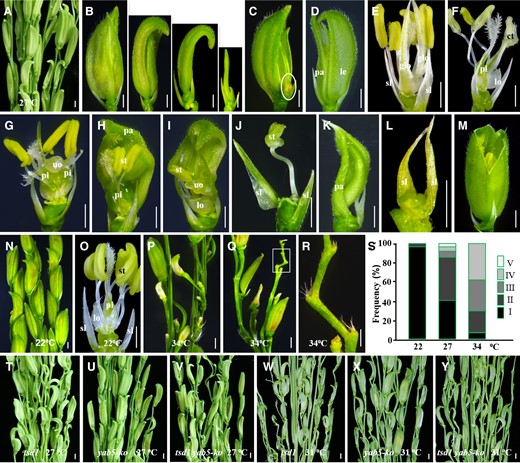
yab5-ko and tsd1 yab5-ko resembled tsd1 in spikelet appearance and response to temperature change. A, Part of a mutant panicle. B–M, Mutant spikelets at 27°C. B, Several spikelets with smaller palea/lemma. C, A spikelet with an additional degenerated-spikelet organ (indicated by an ellipse). D, A spikelet with opened and deformed glumes without the special marginal tissue in palea. E, A spikelet with glume-like organs transformed from lodicules. F, A chimeric tissue transformed from stamens. A spikelet with reduced stamen number but increased pistil number (G) or pistil size (H). I, A spikelet with one stamen and an unidentified organ but without a pistil. J, A spikelet with an abnormal stamen inside lemma/palea. K, A spikelet with a deformed palea without other floral organs. (L) A spikelet without floret. M, A cone-shaped spikelet with completely fused lemma–palea. Part of mutant panicle (N) and a spikelet (O) growing at 22°C. P, Q, Part of mutant panicles growing at 34°C. R, Close-up view of the degenerated spikelets in (Q; indicated by a rectangle). The lemma and/or palea were removed from the mutant spikelets in (E–J, O). S, The proportions of various spikelet phenotypes in yab5-ko at different temperatures. (I) Normal inner three whorls of organs; (II) reduced number and abnormal morphology of stamen and/or pistil; (III) loss of organs inside lemma/palea; (IV) loss of floret; and (V) formation ectopic organs. T–Y, tsd1 yab5-ko showed similar spikelet phenotypes to tsd1 under different temperatures. Increasing temperature aggravates spikelet degeneration in tsd1, yab5-ko, and tsd1 yab5-ko, but without significant morphological alteration was observed between tsd1 and tsd1 yab5-ko spikelets. ct, chimeric tissue; glo, lemma/palea-like organ; le, lemma; lo, lodicule; pa, palea; pi, pistil; sl, sterile lemma; st, stamen; uo, unidentified organ; bars = 1 mm.
To confirm the molecular and genetic relationship between TSD1 and YABBYs in spikelet development, we created the tsd1 yab5-ko double mutant and examined the spikelet phenotypes. The double mutant displayed similar mutant phenotypes to tsd1 without producing additional or more severe defects at both 27°C and 31°C (Figure 8, T and U), indicating that TSD1 and YABBY5 are likely to function in the same genetic network to modulate spikelet development.
Discussion
Two major classes of transposase-derived transcription factors, FHY3/FAR1 family and MUSTANG (MUG; Cowan et al., 2005; Joly-Lopez et al., 2012) family, have been identified in plants. These plant-specific transcription factors make important contributions to the adaptation and evolution of their host genomes and are increasingly recognized as important sources of beneficial agricultural traits and adaptive functions in plants in response to changing environments (Chae et al., 2021; Nicolau et al., 2021). FHY3/FAR1 family genes are widespread in angiosperms. Their functions have been well characterized in Arabidopsis, but the knowledge about the homologous genes in other species is still very limited (Ma and Li, 2018; Chae et al., 2021). In this study, we characterized an FHY3/FAR1 family gene TSD1 in rice. Our results indicated that TSD1 was highly expressed in spikelets depending on ambient temperature and specifically played a critical role in the thermomorphogenesis of spikelets. Loss of TSD1 function resulted in thermo-sensitive spikelet degeneration, producing severely defective or even completely degenerated spikelets under high temperatures (Figures 1–3). Interestingly, FHY3 also plays a role in seed reproduction and FM determinacy in Arabidopsis (Li et al., 2016), in addition to its well-known multifaceted role in plant growth and development during the vegetative stage (Ma and Li, 2018). Mutation of FHY3 can reduce SAM size, result in small petals, sterile anthers, and very short bulged siliques, and enhance the indeterminacy of weak allele ag-10 (Li et al., 2016). Therefore, FHY3 is another FHY3/FAR1 family gene that has been found to control plant reproductive development. However, the effect of FHY3 on reproductive development is markedly distinct from that of TSD1. In particular, it is unclear whether the effect of FHY3 is dependent on ambient temperature or not.
Phylogenetic analysis shows that FHY3/FAR1 family genes in angiosperms can be divided into 28 subgroups, denoted as G1 to G28 (Chae et al., 2021). TSD1 is located in a Poaceae-specific subgroup G25, which is quite distant from the subgroups G6 of FRS7/12 and G7 of FHY3/FAR1, although the Arabidopsis FRS7/12 also contains the same conserved domains as in TSD1 (Figure 4B; Ma and Li, 2018; Chae et al., 2021). These results suggest that TSD1 is a Poaceae-specific gene, which has greatly diverged from the homologs of dicotyledonous species in both structure and function during evolution. Nevertheless, whether the function of TSD1 identified in this study is conserved in the genes of other Poaceae species in G25 remains to be further investigated.
Reproductive organs are the most sensitive and vulnerable to high temperatures in plants. In rice, high temperature attenuates the differentiation of secondary branches and spikelets and promotes their degradation in sensitive varieties (Wu et al., 2016). In this study, we demonstrated that TSD1 is essential to maintaining spikelet origination and fate at high temperatures. Several YABBY genes have also been found to have similar functions in rice. TOB1/YABBY5 is confirmed to be involved in spikelet formation (Tanaka et al., 2012), whereas TOB2/YABBY4 and TOB3/YABBY3 probably play a redundant role with TOB1 in the maintenance and fate of spikelet because RNAi of either/both of these two genes can exacerbate the mutant phenotype of tob1 (Tanaka et al., 2017). Moreover, it is found that the effects of the three TOB genes are influenced by environments because the spikelet phenotypes of tob1 and TOB2-TOB3-RNAi; tob1 change with the season (Tanaka et al., 2012, 2017). However, it is unclear which environmental factors affect the mutant phenotypes of the three TOB genes. By creating the knockout mutant yab5-ko with the same genetic background as that of tsd1, we verified that yab5-ko as well as tsd1 yab5-ko double mutant closely resembled tsd1 in spikelet appearance and response to temperature change (Figure 8), indicating that TSD1 and YABBY5 are likely involved in the same genetic network to modulate spikelet development. Particularly, the phenotype of branch development termination without forming spikelets in tsd1 (Figure 3) was also observed in yab5-ko (Figure 8) as well as in TOB2-TOB3-RNAi; tob1 (Tanaka et al., 2017). Although TSD1 and TOB1/YABBY5 displayed similar functions in spikelet thermomorphogenesis, they could not compensate for each other's function loss. This suggests that they interact with each other in the mode of complementary gene action or duplicate recessive epistasis. However, the mutant phenotype of yab5-ko (Figure 8) was not as severe as that of tsd1 (Figure 3), suggesting that TSD1 has a larger effect than TOB1/YABBY5 on spikelet thermomorphogenesis. We found that TSD1 could directly promote the transcription of TOB3 and YABBY1 (Figure 6) and physically interact with the three TOB proteins and YABBY1 (Figure 7). In Arabidopsis, YABBY proteins can form either homodimers or heterodimers, which involved in lateral organ formation and FM termination via transcriptional repression or activation (Stahle et al., 2009). We also found that these four YABBY proteins can form either homodimers or heterodimers, similar to the Arabidopsis orthologous proteins (Supplemental Figure 8). The above results suggest that TSD1 and the three TOB genes play a similar role in rice spikelet thermomorphogenesis, and they (probably also including YABBY1) are involved in the same genetic network. Additional genetic evidence would further support the conclusion.
High temperature can alter phytohormone production and signaling, and induce transcriptomic reprogramming and metabolomic changes in plants, which in turn helps plants adapt to the high temperature (Li et al., 2018; Jha et al., 2022). Phytohormones play critical roles in preventing spikelet degeneration under heat stress in rice (Wu et al., 2016; Wang et al., 2018). Recent studies reveal that transcription factors are pivotal molecular players in mediating inflorescence thermomorphogenesis through hormonal regulation. Arabidopsis SPL1 and SPL12 contribute greatly to inflorescence thermotolerance through PYL-mediated ABA signaling (Chao et al., 2017); whereas barley HvMADS1 maintains unbranched spikes under high ambient temperature by modulating cytokinin (CK) homeostasis (Li et al., 2021). YABBYs are plant-specific transcription factors, which recognize cis-acting elements of phytohormone and stress response (Zhang et al., 2020), but whether and how YABBY genes regulate stress response are still unclear. In rice, YABBY1 and YABBY4 both participate the modulation of growth and development through the gibberellin (GA) signaling pathway. YABBY1 directly binds to a GA-responsive element in the GA3ox2 promoter to influence the feedback regulation of GA biosynthesis (Dai et al., 2007). YABBY4 serves as a DNA-binding intermediate protein for SLR1(the sole DELLA protein negatively controlling GA responses in rice), which negatively controls GA responses (Yang et al., 2016). In Arabidopsis, DELLA proteins have genetically separable roles in controlling stem growth and the size of the inflorescence meristem, where flowers initiate (Serrano-Mislata et al., 2017). GA is an important phytohormone regulating thermomorphogenesis in plants such as rice and Arabidopsis (Koini et al., 2009; Wu et al., 2016, 2019; Ding et al., 2020). In rice, GA contributes greatly to preventing spikelet degeneration under heat stress and other abiotic stresses (Yokoyama et al., 2002; Wu et al., 2016, 2019). The application of GA3 can suppress rice spikelet degeneration under the stress of saline flooding (Yokoyama et al., 2002). Heat stress suppresses GA biosynthesis and promotes GA deactivation in young panicles and disrupts phytohormone homeostasis, so as to attenuate the differentiation and promote the degradation of secondary branches and attached florets, especially in heat-sensitive rice varieties (Wu et al., 2016). Therefore, it is possible that YABBY1 and YABBY4 enhance thermotolerance to maintain spikelet fate under high temperatures through GA modulation.
Collectively, TSD1 and four YABBY genes (YABBY1 and three TOB genes) are all essential for spikelet morphogenesis in rice. Particularly, YABBY5 acts complementarily to TSD1 for spikelet thermotolerance, making spikelet development acclimate to local high temperatures (Figures 1–3 and 8). TSD1 directly regulates YABBY1 and YABBY3 transcription (Figure 6) and physically interacts with the four YABBY proteins (Figure 7). Besides, these four YABBYs can form either homodimers or heterodimers (Supplemental Figure 8). They all are known to play important roles in FM activity maintenance and lateral organ development, similar to that of TSD1. Moreover, both yab5-ko mutant and tsd1 yab5-ko double mutant resembled tsd1 in spikelet appearance and response to temperature. The above findings confirm that TSD1 and the four YABBYs are all related, and probably involved in the same genetic network. Based on these findings, we can establish a primary model: a TSD1–YABBY module enhances spikelet thermotolerance and maintains spikelet origination and fate under high temperatures (Figure 9). This provides insight into the molecular mechanism of thermotolerance in plant reproductive development. Nevertheless, it remains unknown how the temperature signal is sensed and conducted to TSD1, and whether and how YABBYs mediate GA modulation to alleviate or prevent heat damage of spikelets due to temperature increase. These questions deserve further investigation.
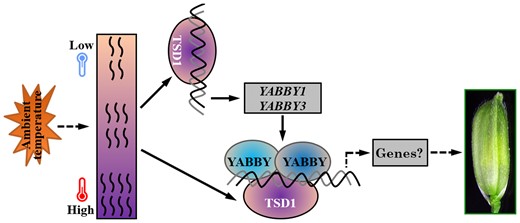
Proposed composition of the TSD1–YABBY complex and its role in acclimatizing spikelet initiation and development to high ambient temperature in rice. TSD1 is highly expressed in spikelets, induced by heat, and specifically enhances the thermotolerance during spikelet morphogenesis. TSD1 could directly bind to the promoters of YABBY1 and YABBY3, and physically interact with YABBY1, 3, 4, and 5. These four YABBY proteins can form homodimers and heterodimers. Given the redundant role of YABBY genes and the broadly similar phenotypes of tsd1, yab5-ko, tsd1 yab5-ko, and triple mutant TOB2-TOB3-RNAi; tob1 (Tanaka et al., 2017), it is reasonable to speculate that these genes likely participate in spikelet development through the cooperative TSD1–YABBY pathway. The arrows represent a direct relationship or positive regulation; the dotted arrows indicate the possible regulation or relationship that has not been directly demonstrated in the specific process.
Materials and methods
Plant materials and growth conditions
Rice cultivars Minghui-86 (MH86, indica cv.), DZ60 (tropical japonica), Zhonghua-11 (ZH11, japonica), and four mutants from MH86, namely tsd1, tsd1-ko, yab5-ko, and Os02g0232500-ko were used in the study. tsd1 was obtained by 60Co gamma-ray radiation mutagenesis, whereas the other three mutants were generated by CRISPR/Cas9 editing system. All four mutants could set a small number of seeds under mild ambient temperature.
To assess the impact of photoperiod, plants were cultivated in a growth chamber at 30°C/24°C (day/night), and with 10 h/14 h and 14 h/10 h (day/night) photoperiod, respectively. To assess the impact of temperature, plants were cultivated in a growth chamber with a photoperiod of 12 h day/12 h night at different day/night temperature combinations, namely, 24°C/20°C, 29°C/25°C, 33°C/29°C, 34°C/30°C, 37°C/33°C, and 37°C/37°C.
Scanning electron microscopy
Young panicles (1–10 mm) were observed by scanning electron microscopy (SEM). Panicles were fixed in 2.5% (v/v) glutaric dialdehyde and washed with a sodium phosphate buffer (0.1 M, pH7.0); further fixed in 1% osmic acid (w/v) for 1–2 h and again washed with the sodium phosphate buffer; dehydrated with an ethanol series, incubated in ethanol-tert butanol and then in tert butanol; and finally observed with a TM3030 Plus scanning electronic microscope (Hitachi, Japan).
Identification of TSD1 locus
To isolate the TSD1 gene, we adopted a map-based approach and the next-generation sequencing technique. An F2 population was developed from the cross between tsd1 and DZ60. A total of 478 F2 plants displaying the tsd1 mutant phenotype were selected for gene mapping. To identify the candidate gene, we performed a whole-genome resequencing wild-type and mutant DNA pools, which were made from a mixture of equal-amount fresh leaves of 30 homozygous wild-type lines and from that of 30 mutant lines randomly selected from the F2:3 population, respectively. The two DNA pools were sequenced using the Illumina PE Genome Analyzer. Based on the sequence information of the two pools, the TSD1 locus was identified. The primers used for mapping TSD1 are listed in Supplemental Table 4.
Vector construction and plant transformation
For the genetic complementation test, a 6583-bp genomic DNA sequence of TSD1 was amplified from MH86, and fused into the binary vector pCAMBIA1300. The plasmid was introduced into tsd1 embryonic calli. To create mutants of Os02g0232500-ko, tsd1-ko, and yab5-ko, CRISPR/Cas9 targets of the three genes were selected as described (Miao et al., 2013), and vector construction was performed according to the manufacturer's instruction of regent kit (VIEWSOLID Biotech, China). The vectors were transferred into MH86. To determine the expression patterns of TSD1, a 2287 bp promoter upstream of the coding region of TSD1 was amplified from MH86 and fused into the GUS reporter gene in pCAMBIA1391Z. The plasmid was introduced into ZH11. The primers used for vector construction are listed in Supplemental Table 4.
All constructs were introduced into Agrobacterium tumefaciens strain EHA105 and transferred into rice. The genomic regions surrounding the CRISPR target sites of TSD1, YABBY5, and Os02g0232500 were amplified and sequenced to screen for mutants. Histochemical assay for GUS activity in transgenic plants was performed as described (Jefferson et al., 1987).
RNA isolation and Rt-qPCR analysis
RT-qPCR analysis was performed according to Duan et al. (2019). Total RNA was isolated using a Trizol reagent kit (Omega R6934, USA). Reverse transcription was performed using the PrimeScript RT reagent kit (Takara RR047A, China). RT-qPCR analysis was performed with three biological replicates using the SYBR Premix Ex Taq II (Takara, China) in an ABI QuantStudio 3 detect system. Amplification of Actin was used as an internal control to normalize all data. Primers used for RT-qPCR analysis are listed in Supplemental Table 4.
mRNA in situ hybridization
Tissue fixation, embedding, and sectioning were done according to Duan et al. (2019). Digoxygenin-labeled sense or antisense RNA probes were prepared following the manufacturer's recommendation. The tissues were sliced into 8-mm sections with a rotary microtome and attached to microscope slides. Primers used for mRNA in situ hybridization were 5′–cgaacccacagaggaagagg–3′ and 5′–gaggatttcgcctgcgtttg–3′.
Subcellular localization of TSD1
The full-length cDNA of TSD1 was amplified and inserted into pRTVcGFP (He et al., 2018). The pRTVcGFP-TSD1 plasmid was transiently transformed into rice protoplasts from ten-day-old seedlings by the polyethylene glycol/calcium-mediated transformation method. DsRed-OsH2B was cotransfected for nuclear localization control (Chen et al., 2019). The samples were observed with a confocal laser scanning microscope (LEICA-SP8). Primer pairs for amplifying TSD1 cDNA are listed in Supplemental Table 4.
Yeast two-hybrid
Y2H analysis was performed according to the manufacturer's instruction of Matchmaker Gold Yeast Two-Hybrid System (Clontech, Cat. No.630489). The coding region of YABBY1, YABBY3, YABBY4, and YABBY5 were amplified and inserted into pGADT7 as prey. Different construct combinations of bait and prey were cotransformed into the yeast strain Y2H Gold and culture on SD/-Leu-Trp medium plates at 30°C. The interactions were examined on the selective medium SD-Leu/-Trp/-His/-Ade supplemented with 5 mm 3-AT. The transformants containing empty plasmids pGADT7 and pGBKT7 served as a negative control. Primers for Y2H are listed in Supplemental Table 4.
Yeast one-hybrid
The coding sequence of TSD1 was amplified and fused into the pB42AD vector. The promoter regions of YABBY1 and YABBY3 including FBS cis-elements were amplified and inserted into the pLacZi2μ vector. Plasmids were cotransformed into the yeast strain EGY48 and cultured on a synthetic medium DDO (-Ura/-Trp) plus 5-Bromo-4-chloro-3-indolyl-β-D-galactopyranoside (X-β-gal) at 30°C. The yeast transformation was performed according to the manufacturer's instructions. Primer pairs for Y1H are listed in Supplemental Table 4.
Bimolecular fluorescence complementation (BiFC)
The full-length CDS of TSD1, YABBY1, YABBY3, YABBY4, and YABBY5 were amplified and inserted into the N-terminal fragment (YN) and the C-terminal fragment (YC) of the GFP vector pCAMBIA1300S, respectively. The fusion constructs were cotransformed into rice protoplast from ten-day-old seedlings. The vector combinations of YN + YC, TSD1-YN + YC, four YN + YABBY-YC, and four YN-YABBY + YC from different YABBY genes were used as negative controls to verify the specificity of the interactions. Fluorescence in transformed protoplast was examined at 16 h after cotransformation and imaged using LEICA-SP8. The primers used for vector construction are listed in Supplemental Table 4.
Dual-luciferase reporter (LUC)
The promoter sequences of YABBY1 and YABBY3 containing FBS cis-elements were amplified and inserted into the pGreenII 0800-LUC vector as a reporter. The coding sequence of TSD1 was inserted into the pGreenII 62-SK vector with a 35S promoter as effector and the pGreenII 62-SK empty vector as an internal control. About 10 mg of effector and reporter plasmids were cotransfected into rice protoplasts and incubated for 16 h at 28°C in the dark. The luciferase activity was measured using the Luciferase Reporter Assay System (Promega) according to the manufacturer's instructions. The primers for the LUC assay are listed in Supplemental Table 4.
Firefly LCI
The full CDS of OsTSD1 was cloned into pCAMBIA1300-nLUC, and the full CDS of four YABBY genes were cloned into pCAMBIA1300-cLUC and pCAMBIA1300-nLUC, respectively. Different construct combinations of plasmid were transformed into A. tumefaciens strain GV3101 and N. benthamiana leaves were infiltrated with Agrobacterium suspensions. After incubated for 72 h, the leaves were infiltrated with 1 mM precooled luciferin (Promega; E1603). The luciferase intensity was imaged using an imaging system equipped with a cold CCD (NightSHADE LB985). Primer pairs for LCI assay are listed in Supplemental Table 4.
ChIP-qPCR
The ChIP assays were performed as described with minor modifications (Lee et al., 2017). Briefly, the pRTVcGFP-TSD1 was transiently transformed into rice protoplasts from ten-day-old tsd1 seedlings. The transformed protoplasts were crosslinked with 1% formaldehyde, and then were sheared into 200–500 bp size by sonication and incubated with GFP polyclonal antibody (Invitrogen; A11122) and IgG (MBL; PM035) for 2 h at 4°C, respectively. Then, 20 μL Magna ChIP Protein A + G Magnetic Beads (Sigma-Aldrich; 16–663) were added and incubated for 2 h at 4°C. The immunocomplexes were purified using the kit (Qiagen; 28104). The purified DNA was analyzed by quantitative PCR. Primer pairs for ChIP-qPCR assay are listed in Supplemental Table 4.
Co-immunoprecipitation
The ORF of four YABBY genes were amplified and inserted into pRTVcGFP (He et al., 2018) to generate expression vectors pRTVcGFP-YABBY, respectively. Full-length cDNA of TSD1 was amplified and cloned into the psuper1300-MYC. The Co-IP assay was performed as described (Kong et al., 2019). Briefly, the plasmids were used to transiently cotransform rice protoplasts from 10-day-old etiolated seedlings. After incubation for 16 h, total proteins were extracted from the protoplasts and incubated with GFP-Nanoab-Agarose (Lablead, GNA-20-400) for 1 h. The GFP-Nanoab-Agarose was washed four times with lysis buffer and then boiled in the 2XSDS-PAGE buffer. The immunoprecipitation products were detected by SDS-PAGE and western blot using anti-Myc (MBL, M047-3) or anti-GFP (MBL, 598) antibody. The primers used for vector construction are listed in Supplemental Table 4.
Accession numbers
Sequence data for genes described in this article can be found in the GenBank/EMBL/Gramene data libraries or Web site under accession numbers: TSD1 (LOC_Os02g18370), YABBY1 (LOC_Os07g06620), YABBY3 (LOC_Os10g36420), YABBY4 (LOC_Os02g42950), and YABBY5 (LOC_Os04g45330).
Supplemental data
The following materials are available in the online version of this article.
Supplemental Figure S1. Phenotypes of tsd1 panicles under short days and long days
Supplemental Figure S2. Phenotype of Cas9-induced knockouts of Os02g0232500 in the wild-type.
Supplemental Figure S3. Phylogenetic analysis of FH3/FAR1 family proteins of rice and other plants.
Supplemental Figure S4. Phenotypes of Cas9-induced knockouts of TSD1 in the wild-type.
Supplemental Figure S5. Promoter regions of YABBY1 and YABBY3 with the FBS motifs.
Supplemental Figure S6. Negative controls of BiFC analyses showing the interaction between TSD1 and YABBY proteins in rice protoplasts.
Supplemental Figure S7. LUC assay of luciferase activity showed that the TSD1–YABBY complex maintained the transcriptional activity of TSD1.
Supplemental Figure S8. The homo- and heterodimer forms in YABBY1, 3, 4 and 5.
Supplemental Table S1. Primers used in this study.
Supplemental Table S2. Results of Os02g0232500 sequence editing by Cas9-induced knockout.
Supplemental Table S3. Results of TSD1 sequence editing by Cas9-induced knockout.
Supplemental Table S4. Results of YABBY5 sequence editing by Cas9-induced knockout.
Acknowledgments
The authors are grateful to Mathew M.S. Evans (Department of Plant Biology, Carnegie Institution for Science, USA) and the anonymous editors, and reviewers for offering valuable suggestions and help to improve the manuscript. They thank Ms. Ling LU for technical assistance in performing the LCI assay and Dr. Shengping Li for providing the vectors of LCI assay and EMSA assay and Dr. Songbiao Chen for providing the DsRed-OsH2B vector. They also thank Dr. Zhiwei Chen, Dr. Huazhong Guan, and Ms. Damei Mao of Fujian Agriculture and Forestry University for their help in the experiment.
Funding
This project was supported by grants from the National Natural Science Foundation of China (31871600), the Natural Science Foundation of Fujian Province (2021J01074), and the Chinese Scholarship Council (201908350032).
References
Author notes
Y.D. and W.W. conceived and designed the research plans, Y.D. participated and supervised throughout the project, Z.C. performed most of the experiments, G.W. identified and characterized TSD1, J. L. made some of the constructs and performed the LCI and Y2H assay. L.K. created and identified the yab5-ko mutants, W.T. and X.C. performed whole-genome resequencing analysis, X.Q., C.L. Y. P., Y.L., Z.D., and Y.Y. completed partial experiments. Y.D. and W.W. analyzed the data, Y.D. wrote the manuscript and W.W. revised the manuscript.
The authors responsible for the distribution of materials integral to the findings presented in this article in accordance with the policy described in the Instructions for Authors (https://dbpia.nl.go.kr/plphys/pages/General-Instructions) are Yuanlin Duan ([email protected]) and Weiren Wu ([email protected]).
Conflict of interest statement. None declared.