-
PDF
- Split View
-
Views
-
Cite
Cite
Haiyan Cao, Yali Liang, Liping Zhang, Zhiqiang Liu, Danmei Liu, Xiaofeng Cao, Xian Deng, Zhuping Jin, Yanxi Pei, AtPRMT5-mediated AtLCD methylation improves Cd2+ tolerance via increased H2S production in Arabidopsis, Plant Physiology, Volume 190, Issue 4, December 2022, Pages 2637–2650, https://doi.org/10.1093/plphys/kiac376
- Share Icon Share
Abstract
Arabidopsis (Arabidopsis thaliana) PROTEIN ARGININE METHYLTRANSFERASE5 (PRMT5), a highly conserved arginine (Arg) methyltransferase protein, regulates multiple aspects of the growth, development, and environmental stress responses by methylating Arg in histones and some mRNA splicing-related proteins in plants. Hydrogen sulfide (H2S) is a recently characterized gasotransmitter that also regulates various important physiological processes. l-cysteine desulfhydrase (LCD) is a key enzyme of endogenous H2S production. However, our understanding of the upstream regulatory mechanisms of endogenous H2S production is limited in plant cells. Here, we confirmed that AtPRMT5 increases the enzymatic activity of AtLCD through methylation modifications during stress responses. Both atprmt5 and atlcd mutants were sensitive to cadmium (Cd2+), whereas the overexpression (OE) of AtPRMT5 or AtLCD enhanced the Cd2+ tolerance of plants. AtPRMT5 methylated AtLCD at Arg-83, leading to a significant increase in AtLCD enzymatic activity. The Cd2+ sensitivity of atprmt5-2 atlcd double mutants was consistent with that of atlcd plants. When AtPRMT5 was overexpressed in the atlcd mutant, the Cd2+ tolerance of plants was significantly lower than that of AtPRMT5-OE plants in the wild-type background. These results were confirmed in pharmacological experiments. Thus, AtPRMT5 methylation of AtLCD increases its enzymatic activity, thereby strengthening the endogenous H2S signal and ultimately improving plant tolerance to Cd2+ stress. These findings provide further insights into the substrates of AtPRMT5 and increase our understanding of the regulatory mechanism upstream of H2S signals.
Introduction
Hydrogen sulfide (H2S) has been identified as a gasotransmitter, together with nitric oxide and carbon monoxide, and it participates in a wide range of physiological processes (Wang, 2002). In plants, H2S is involved in seed germination, root morphogenesis, stomatal movement, and photosynthesis (Zhang et al., 2009, 2020a; Zhou et al., 2018; Chen et al., 2020; Shen et al., 2020). Moreover, H2S participates in response to many abiotic stresses, including drought, salt, low temperature, and osmotic stresses, resulting in research interest (Jin et al., 2013; Corpas, 2019; Liu et al., 2019; Jiang et al., 2020).
Cadmium (Cd) is a kind of environmental pollutant that has serious adverse impacts on plants. Through crops, Cd easily infiltrates the food chain, and it is seriously harmful to human and animal health (Buchet et al., 1990). In plants, Cd stress causes a series of morphological and physiological changes, such as leaf chlorosis, leaf curl, inhibited root elongation, biomass reduction, stomatal closure, imbalanced water absorption, and inhibited photosynthesis (Clemens, 2006; Clemens and Ma, 2016).
H2S can effectively enhance the tolerance to Cd stress in various plants. For example, under Cd2+-stress conditions, an exogenous H2S pretreatment can affect the relative absorptions of K+ and Ca2+ and increase photosynthesis and antioxidant enzymatic activities to alleviate damage in Nicotiana benthamiana seedlings (Wang et al., 2021). H2S can stimulate the activities of AsA-GSH cycle enzymes in tomato (Solanum lycopersicum L.) (Alamri et al., 2020) or participate in salicylic acid production in Arabidopsis (Qiao et al., 2015) to improve its Cd2+ tolerance. In addition, H2S can reduce ROS accumulation and inhibit Cd2+-induced root tip cell death in cucumber (Cucumis sativus L) and Chinese cabbage (Brassica rapa L. ssp. pekinensis) by activating the antioxidant system (Zhang et al., 2015; Luo et al., 2020).
In plants, l-cysteine desulfhydrase (LCD) and d-cysteine desulfhydrase (DCD) are important H2S-generating enzymes that can degrade cysteine (Cys) into H2S, NH3, and pyruvate (Riemenschneider et al., 2005; Papenbrock et al., 2007). The transcriptional expression of LCD and DCD is up-regulated by Cd2+ stress (Cui et al., 2014; Zhang et al., 2015; Lv et al., 2017). Under Cd2+-stress conditions, an atlcd mutant shows greater Cd2+ sensitivity, whereas the phenotype of AtLCD-overexpressing (OE) Arabidopsis plants is the opposite (Qiao et al., 2015). Some factors regulating the expression of H2S generating genes have been reported. The TGA3 factor binds to the AtLCD promoter region and activates its expression, thereby increasing the production of H2S (Fang et al., 2017). Similarly, the trans-factor WRKY13 binds to the promoter of AtDCD and upregulates its expression (Zhang et al., 2020b). In general, our understanding of the upstream regulatory mechanisms of H2S production is still very limited. In particular, whether the LCD activity can be regulated at the post-translational level is still not known.
Protein Arg methylation is an important post-translational modification. It is involved in different key biological processes, including RNA processing, DNA repair, and signal transduction, and it is considered an epigenetic regulator of transcription (Bedford and Richard, 2005; Bedford and Clarke, 2009). This modification is catalyzed by a group of protein Arg methyltransferases (PRMTs) that are divided into types I and II on the basis of biochemical activity (Blanc and Richard, 2017). PROTEIN ARGININE METHYLTRANSFERASE5 (PRMT5) (At4g31120), also known as SKB1, mediates the symmetric Arg dimethylation and is the most critical member of this family (Wang et al., 2007).
PRMT5 is involved in the regulation of plant growth and development, including the growth rate, flowering time, root development, circadian rhythm, and bud regeneration (Pei et al., 2007; Wang et al., 2007; Schmitz et al., 2008; Hong et al., 2010; Sanchez et al., 2010; Li et al., 2016; Liu et al., 2016a). PRMT5 also plays critical roles in the perception of environmental cues by plants, including abiotic stresses and vernalization. An atprmt5 mutant shows a high salt sensitivity. Under salt-stress conditions, AtPRMT5 was dissociated from the chromatin. Thus, the level of H4R3sme2 decreased, which induced stress response gene expression. In addition, the methylation level of the small nuclear ribonucleoprotein Sm-like4 (LSM4) increased, which then affected the splicing of stress–response genes (Zhang et al., 2011). In Arabidopsis, AtPRMT5-mediated histone H4R3 dimethylation negatively regulates iron homeostasis. The buds of atprmt5 mutants accumulate more iron and show an insensitivity to iron deficiency (Fan et al., 2014). The gasotransmitter nitric oxide increases the methyltransferase activity of AtPRMT5 by increasing its S-nitrosylation level, which improves the plant’s abiotic stress tolerance (Hu et al., 2017). Further, AtPRMT5 mediates the methylation of AtSmD1, D3, U small nuclear ribonucleoproteins, and AtLSM4 proteins, leading to changes in gene alternative splicing. To date, these are the main known functional modes of PRMT5. It still remains to be determined whether there are enzymatic proteins that are direct substrates of PRMT5.
The biological regulatory functions of PRMT5 overlap with those of H2S, especially in abiotic stress responses. This implied an interaction between them. In this study, we detail a pathway in which AtPRMT5 regulates endogenous H2S production and improves heavy metal tolerance through the direct methylation of AtLCD. This is an important discovery that AtPRMT5 directly regulates enzymatic activity through methylation. In addition, we show a regulatory pathway for endogenous H2S emission in plants.
Results
Either AtLCD or AtPRMT5 can improve Cd2+ tolerance in Arabidopsis
LCD enhances plant tolerance when exposed to various abiotic stresses, including drought, salt, high temperature, and heavy metals (Li et al., 2013; Scuffi et al., 2014; Chen et al., 2015; Zhao et al., 2018). To confirm the role of AtLCD in the regulation of Cd2+ tolerance, the AtLCD transcript level was determined in wild type (WT) under Cd2+-stress conditions. AtLCD was induced after 6 h, but did not peak until 12 h (Figure 1A). Consequently, we obtained and identified 35S::AtLCD transgenic plants and the T-DNA insertion mutant atlcd (Supplemental Figures S1 and S2). WT, atlcd, AtLCD-OE1, and AtLCD-OE2 seedlings were cultured on 1/2 Murashige and Skoog (MS) medium with or without Cd2+ for 14 days, and the half-inhibition concentration of Cd2+ was used for screening, as shown in Supplemental Figure S3. The root lengths of seedlings were also analyzed after 14 days. There was no substantial phenotypic difference among different plant lines under normal growth conditions. In Cd2+-containing medium, the AtLCD-OE1 and AtLCD-OE2 plants exhibited a higher tolerance to Cd2+, whereas atlcd plants had a Cd2+-sensitive phenotype compared with WT (Figure 1B). The root lengths of AtLCD-OE1 and AtLCD-OE2 plants were greater compared with that of the WT (Figure 1C). There was a substantially positive association between root length phenotype and AtLCD gene expression (Figure 1B, Heat map).
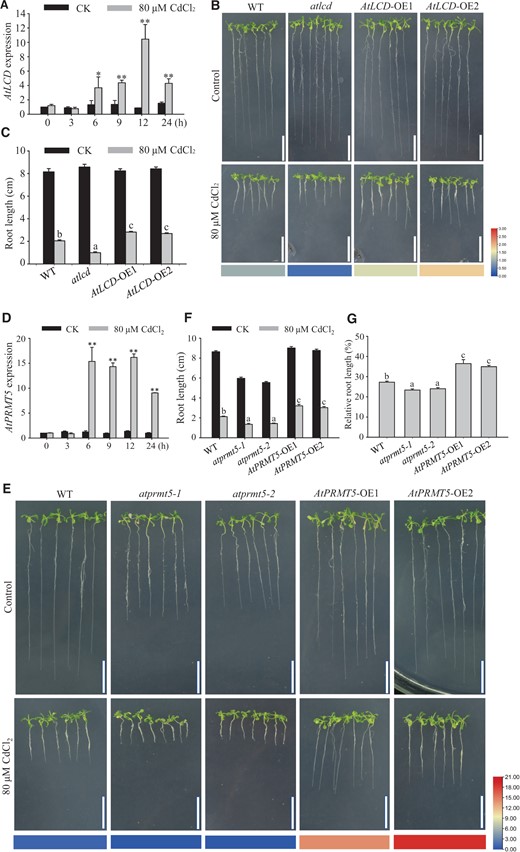
Phenotypic analysis of different plant materials exposed to Cd2+ stress. A, RT-qPCR analysis of AtLCD in WT with (gray column) or without (black column) Cd2+ stress. RNA was extracted from 10-day-old plants exposed to 80 μM CdCl2 for 0, 3, 6, 9, 12, and 24 h. AtACT2 was used as the internal control. Error bars represent SEs, n = 3. B and C, Analysis of the Cd2+ tolerance of WT, atlcd, AtLCD-OE1, and AtLCD-OE2 seedlings grown on 1/2 MS medium. B, Phenotype of seedlings at 14 days without (top panels) and with (bottom panels) the 80 μM CdCl2 treatment; scale bar, 2 cm. C, Root lengths of seedlings at 14 days. Error bars represent SEs, n ≥ 10. The heat map displays AtLCD expression in WT, atlcd, AtLCD-OE1, and AtLCD-OE2 seedlings, and is based on the RT-qPCR data shown in Supplemental Figures S1 and S2. D, RT-qPCR analysis of the AtPRMT5 gene in WT with (gray column) or without (black column) Cd2+ stress. Error bars represent SEs, n = 3. E and F, Analysis of the Cd2+ tolerance of WT, atprmt5-1, atprmt5-2, AtPRMT5-OE1, and AtPRMT5-OE2 seedlings grown on 1/2 MS medium. E, Phenotypes of seedlings grown on medium without (top panels) or with (bottom panels) 80 μM CdCl2 for 14 days. The heat map displays the AtPRMT5 expression in WT, atprmt5-1, atprmt5-2, AtPRMT5-OE1, and AtPRMT5-OE2 seedlings based on the RT-qPCR data shown in Supplemental Figures S1 and S2. Scale bar, 2 cm. F, Root lengths of seedlings at 14 days. Error bars represent SEs, n ≥ 10. G, Root lengths relative to controls of seedlings grown on 1/2 MS medium for 14 days. Error bars represent SEs, n = 3. All the data are means ± se. Experiments were performed three times with similar results. Different letters indicate significant differences (one-way ANOVA test, P < 0.05). * and ** represent significant difference (P < 0.05) and extremely significant difference (P < 0.01), respectively.
Mutations in AtPRMT5 may cause developmental defects and increase sensitivity in response to abiotic stress in plants (Pei et al., 2007; Zhang et al., 2011; Hu et al., 2017). Thus, we investigated whether AtPRMT5 is involved in Cd2+-stress responses. Similarly, the expression of AtPRMT5 started to increase after treating with Cd2+ for 6 h and continued until 24 h (Figure 1D). Subsequently, to further confirm the function of AtPRMT5 in Cd2+-stress responses, we constructed 35S::AtPRMT5 transgenic plants and obtained atprmt5-1 and atprmt5-2 homozygous mutant plants (Pei et al., 2007; Supplemental Figures S1 and S2). After WT, atprmt5-1, atprmt5-2, AtPRMT5-OE1, and AtPRMT5-OE2 seedlings were grown on 1/2 MS medium with or without Cd2+ for 14 days, the root lengths of the seedlings were analyzed. As shown in Figure 1E, the root lengths of atprmt5-1 and atprmt5-2 were inhibited under Cd2+-stress conditions. By contrast, the phenotype of 35S::AtPRMT5 plants has been restored (Figure 1, E and F). The expression of AtPRMT5 (Figure 1E, Heat map) was substantially positive association with root length. Given that root development was affected in the atprmt5 mutants without Cd2+ stress, we analyzed the proportion of root lengths of seedlings treated with Cd2+ compared with those that were not treated with Cd2+ (Figure 1G). The root lengths of WT plants grown on medium containing 80 μM CdCl2 were 20%–30% of the plants grown on normal medium, while the root lengths of AtPRMT5-OE plants were 30%–40% of the plants grown on medium without Cd2+, and atprmt5 mutants showed higher Cd2+ sensitivity than WT plants. The result was consistent with the above phenotypic observation. Thus, AtLCD and AtPRMT5 play vital roles in response to Cd2+ stress in Arabidopsis.
AtPRMT5-mediated methylation of AtLCD at Arg-83 increases the AtLCD activity and H2S production
Based on the above results and our research on the functions of these two genes over the years (Pei et al., 2007; Chen et al., 2015; Fang et al., 2017; Zhao et al., 2018), we hypothesized that there was an interaction between AtPRMT5 and H2S signals in the Cd2+-stress response. Consequently, H2S production was examined in WT, AtPRMT5-OE plants and atprmt5 mutants using the H2S probe 7-azido-4-methylcoumarin (AzMC) (Figure 2, A and B) and methylene blue (Figure 2C). The H2S contents of atprmt5-1 and atprmt5-2 mutants exhibited obvious decreases, whereas the H2S contents of AtPRMT5-OE plants significantly increased compared with the WT.
![AtPRMT5 methylates AtLCD at Arg-83 and increases its activity. A–C, Analysis of the H2S contents in WT, atprmt5-1, atprmt5-2, AtPRMT5-OE1, and AtPRMT5-OE2 seedlings. A, Analysis of the H2S contents in 14-day-old seedlings using the AzMC probe. Scale bar, 100 μm. B, Analysis of fluorescence density using ImageJ2x. C, Analysis of the H2S contents in 14-day-old seedlings using methylene blue methods. D and E, The transcriptional levels of AtLCD in WT, atprmt5-1, and atprmt5-2 plants as assessed by RT-qPCR (D) and RT-PCR (E) using AtACT2 as the internal control. F, Immunoblot analysis of AtLCD in 14-day-old seedlings. AtACT2 was used as the internal control. G, Methylation analysis of His-AtLCD catalyzed by GST-AtPRMT5. Recombinant proteins GST-AtPRMT5 and His-AtLCD were incubated in the presence of the methyl donor S-adenosyl-l-[methyl-3H] at 30°C for 2 h. Coomassie bright blue-stained gel is shown in the top panel and the autoradiograph of the gel is shown in the bottom panel. H, Activity analysis of methylated His-AtLCD catalyzed by GST-AtPRMT5. I, Activity analysis of AtLCD in WT, atprmt5-1, atprmt5-2, AtPRMT5-OE1, and AtPRMT5-OE2 plants grown on 1/2 MS medium for 14 days. J, Activity analysis of methylated His-AtLCD and His-AtLCDArg83Ala proteins catalyzed by His-TF-AtPRMT5. All the data are means ± se (n = 3). Experiments were performed three times with similar results. Different letters indicate significant differences (one-way ANOVA test, P < 0.05). * and ** represent significant difference (P < 0.05) and extremely significant difference (P < 0.01), respectively.](https://oup.silverchair-cdn.com/oup/backfile/Content_public/Journal/plphys/190/4/10.1093_plphys_kiac376/1/m_kiac376f2.jpeg?Expires=1750426244&Signature=LszZjCVHqw6O4gJFrxgLngaLWl49O4FXvK2uSsxMXh0iu3uIQjWSwKhkexIrJ3vpN3vOrK-RqCt0ykbBYflZWGIzHTUpgVcCngmb2OsrNTO6-XyPyVjJPDx1iGO859Yaw2UCkeOrbEoooVibfEU2oTAl33~EyOTY3CTE6U9RItWbLZPWsbsoNsPcqBmR7c1Fn9gavzasm6pXbDnAuapwAs18ooxKl1oQzZeUOuik3EUHTiWCTVAMoCIFaTk0ypkOH1idQ3BY7SmpMgv6Y4kiJF2wHoSxfvyr82Lem437dUWMerH8glo6ELKJHakOXTE2Im9mdOrKQChWOdS4CkaSnw__&Key-Pair-Id=APKAIE5G5CRDK6RD3PGA)
AtPRMT5 methylates AtLCD at Arg-83 and increases its activity. A–C, Analysis of the H2S contents in WT, atprmt5-1, atprmt5-2, AtPRMT5-OE1, and AtPRMT5-OE2 seedlings. A, Analysis of the H2S contents in 14-day-old seedlings using the AzMC probe. Scale bar, 100 μm. B, Analysis of fluorescence density using ImageJ2x. C, Analysis of the H2S contents in 14-day-old seedlings using methylene blue methods. D and E, The transcriptional levels of AtLCD in WT, atprmt5-1, and atprmt5-2 plants as assessed by RT-qPCR (D) and RT-PCR (E) using AtACT2 as the internal control. F, Immunoblot analysis of AtLCD in 14-day-old seedlings. AtACT2 was used as the internal control. G, Methylation analysis of His-AtLCD catalyzed by GST-AtPRMT5. Recombinant proteins GST-AtPRMT5 and His-AtLCD were incubated in the presence of the methyl donor S-adenosyl-l-[methyl-3H] at 30°C for 2 h. Coomassie bright blue-stained gel is shown in the top panel and the autoradiograph of the gel is shown in the bottom panel. H, Activity analysis of methylated His-AtLCD catalyzed by GST-AtPRMT5. I, Activity analysis of AtLCD in WT, atprmt5-1, atprmt5-2, AtPRMT5-OE1, and AtPRMT5-OE2 plants grown on 1/2 MS medium for 14 days. J, Activity analysis of methylated His-AtLCD and His-AtLCDArg83Ala proteins catalyzed by His-TF-AtPRMT5. All the data are means ± se (n = 3). Experiments were performed three times with similar results. Different letters indicate significant differences (one-way ANOVA test, P < 0.05). * and ** represent significant difference (P < 0.05) and extremely significant difference (P < 0.01), respectively.
To further explore the reason for AtPRMT5 having an effect on the H2S content, the transcript level of AtLCD and its protein expression was determined in WT and atprmt5 mutant plants. The AtLCD expression level and AtLCD protein level did not substantially change in atprmt5 plants (Figure 2, D–F). PRMT5 can methylate histone H4, H2A, and RNA processing factors. Is AtLCD a substrate of AtPRMT5? To test this hypothesis, recombinant protein AtPRMT5 was incubated with AtLCD in the presence of the methyl donor S-adenosyl-l-[methyl-3H] Met for 2 h (Supplemental Figure S4). SM-like protein LSM4, which had been identified as a methyltransferase substrate previously (Zhang et al., 2011), served as a positive control. The autoradiography results showed that the methylation of AtLCD was mediated by AtPRMT5 (Figure 2G). Thus, AtLCD is indeed a substrate of AtPRMT5. Next, we determined whether the activity of AtLCD was affected by AtPRMT5-mediated methylation. To answer this question, the Cys desulfhydrase activity of methylated-AtLCD via AtPRMT5 was determined. The methylation mediated by AtPRMT5 increased AtLCD activity significantly (Figure 2H). We further examined AtLCD activity in atprmt5 mutants and AtPRMT5-OE transgenic plants. Consistent with the results in vitro, AtLCD activities were lower in atprmt5-1 and atprmt5-2 mutants, but higher in AtPRMT5-OE plants compared with in WT (Figure 2I). Thus, the reduced H2S content in atprmt5 plants may be due to a reduced AtLCD activity caused by weakened AtPRMT5-mediated methylation in Arabidopsis.
Theoretically, 30 Arg residues in the AtLCD protein are putative target sites of methylation mediated by AtPRMT5 (Supplemental Figure S5A). A mass spectrometric analysis of recombinant protein His-AtLCD treated with the His-TF-AtPRMT5 protein in the presence of S-adenosyl-l-methionine (SAM) was carried out, and Arg-83 was identified as a very probable methylated residue (Supplemental Figure S5B). Thus, to further verify the methylation of AtLCD at Arg 83 and determine whether the Arg-83 residue was required for increasing the AtLCD activity mediated by AtPRMT5, we determined the activity of AtLCD mutated at Arg-83 to form Ala. AtLCDArg83Ala showed an obvious lower activity level compared with that of AtLCD after being treated with AtPRMT5 (Figure 2J).
AtPRMT5 interacts with AtLCD in vitro and in vivo
PRMT5 can methylate different substrates, including histones and non-histones (Bedford and Clarke, 2009; Liu et al., 2010). As a binding partner, PRMT5 mediates SREBP1a methylation and promotes transcriptional activity during the process of metabolic reprogramming in cancer cells (Liu et al., 2016b). PRMT5 interacts directly and mediates the methylation of DUSP14, which is involved in T-cell receptor signaling (Yang et al., 2018). To investigate the relationship between AtPRMT5 and AtLCD, subcellular localization assays with AtPRMT5 and AtLCD were performed in N. benthamiana epidermal cells. The merged yellow fluorescence showed that both AtPRMT5 and AtLCD localized to the nucleus (Figure 3A). Then, we performed bimolecular fluorescence complementation (BiFC) assays to determine the interaction between AtPRMT5 and AtLCD. Protein interaction (green fluorescence) was observed when cotransforming nYFP-AtLCD and cCFP-AtPRMT5 or cCFP-AtLCD and nYFP-AtPRMT5 constructs, whereas no green fluorescent signal was detected in N. benthamiana cells cotransformed with AtLCD and cCFP-GUS or AtPRMT5 and cCFP-GUS (Figure 3B). In addition, the merged yellow fluorescence indicated that the interaction occurs in the nucleus. The co-immunoprecipitation (Co-IP) assay was used for determining interactions between AtLCD and AtPRMT5 in vivo (Figure 3C). Protein extracts from WT plants were precipitated using an anti-AtLCD antibody, then the precipitate was examined using anti-AtPRMT5 and anti-AtLCD antibodies. The results confirmed that AtLCD interacted with AtPRMT5 in vivo. Furthermore, we purified the recombinant protein His-TF-AtPRMT5 and incubated it with protein extracts of WT seedlings to examine their interaction in vitro. Again, the pull-down results provide evidence of an interaction between AtPRMT5 and AtLCD (Figure 3D). Thus, AtPRMT5 appears to physically interact with AtLCD.
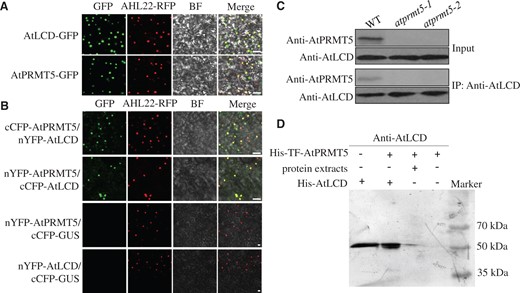
AtPRMT5 interacts with AtLCD. A, Subcellular localizations of the AtLCD and AtPRMT5 proteins. Confocal images of N. benthamiana leaf cells harboring the pMDC43-AtLCD or pMDC43-AtPRMT5 constructs. AHL22 was used as an indicator of nuclei; BF, bright field. Scale bar, 10 μm. B, The interaction between AtLCD and AtPRMT5 in N. benthamiana leaves. Pairwise expression of nYFP-AtPRMT5, cCFP-AtPRMT5, nYFP-AtLCD, cCFP-AtLCD, and cCFP-GUS constructs in N. benthamiana. AHL22 was used as an indicator of nuclei. BF, bright field. cCFP-GUS was used as a negative control. Scale bar, 10 μm. C, The combination of AtLCD and AtPRMT5 in 14-day-old Arabidopsis. Protein extracts of WT were immunoprecipitated by anti-AtLCD antibody, and then, the precipitates were exposed to anti-AtPRMT5 or anti-AtLCD antibodies. D, Pull-down assays of AtLCD and AtPRMT5. Recombinant protein His-TF-AtPRMT5 binds to Ni-NTA agarose matrix, then incubated with protein extracts from WT plants, the precipitates were immunoblotted with anti-AtLCD antibody.
AtLCD acts downstream of AtPRMT5 in response to Cd2+ stress
To further investigate the genetic interaction relationship of AtPRMT5 and AtLCD in response to Cd2+ stress, the atprmt5-2 atlcd homozygous double-mutant was generated by crossing atprmt5-2 with atlcd (Supplemental Figure S6). Then WT, atlcd, atprmt5-2, and atprmt5-2 atlcd seedlings were grown on 1/2 MS medium with or without Cd2+ for 14 days. The growth of atlcd, atprmt5-2, and atprmt5-2 atlcd was inhibited compared with that of the WT under Cd2+-stress conditions (Figure 4A). After 14 days, root lengths were also measured. The results indicated that the root length of WT subjected to Cd2+ stress was 20%–30% of WT plants not subjected to Cd2+ stress. By contrast, the root lengths of atlcd and atprmt5-2 atlcd plants were reduced to 10%–20% of those grown in medium without Cd2+ stress, whereas the atprmt5-2 mutant exhibited Cd2+ sensitivity to a small extent (Figure 4B). The Cd2+ sensitivity of the atprmt5-2 atlcd double mutant was much like that of the atlcd single mutant in response to Cd2+ stress, which suggests that AtLCD acts downstream of AtPRMT5.
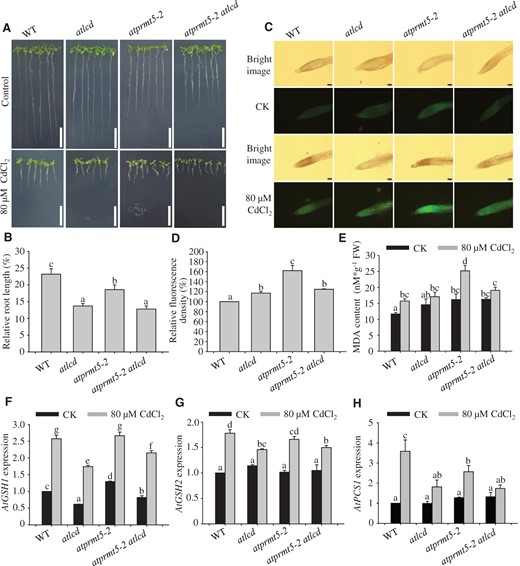
AtLCD acts downstream of AtPRMT5 after exposure to Cd2+. A and B, An analysis of the Cd2+ tolerance of WT, atlcd, atprmt5-2, and atprmt5-2 atlcd seedlings. A, Phenotypes of seedlings grown on 1/2 MS medium with (bottom panels) or without (top panels) 80 μM CdCl2 for 14 days. Scale bar, 2 cm. B, Root lengths relative to controls of 14-day-old seedlings. Error bars represent SEs, n = 3. C and D, Roots of WT, atlcd, atprmt5-2, and atprmt5-2 atlcd plants grown on 1/2 MS medium assessed using Leadamium Green AM dye. Roots from 10-day-old seedlings were transferred to 1/2 MS medium with (bottom panels) or without (top panels) 80 μM CdCl2 for 5 days, and they were then loaded with Leadamium Green AM dye for 90 min. The images were taken at 10× magnification. Scale bar, 100 μm. Green fluorescence signals indicated that Cd2+ and the dye bound. The fluorescence density was analyzed using ImageJ2x software. E, An analysis of the MDA content. The MDA contents were determined at 5 days after 10-day-old seedlings were transferred to the medium supplemented with 80 μM CdCl2. F–H, Expression analysis of Cd2+ stress-related genes in WT, atlcd, atprmt5-2, and atprmt5-2 atlcd plants. The template RNA was isolated from 10-day-old seedlings treated with 80 μM CdCl2 for 12 h. AtACT2 was used as the internal control. All the data are means ± se (n = 3). Experiments were performed three times with similar results. Different letters indicate significant differences (one-way ANOVA test, P < 0.05).
The amount of Cd2+ accumulated in cells is an important factor in plants that display the Cd2+ sensitivity phenotype. To determine the Cd2+ contents in roots of different seedlings, a Cd2+ probe (LeadmiumTM Green AM dye) was used to detect Cd2+ in 10-day-old seedlings transferred to medium with or without 80 μM CdCl2 for 5 days. A weak fluorescence signal was observed in WT, whereas stronger and bright green fluorescence signals were observed in the roots of atprmt5-2 mutant plants exposed to Cd2+ stress. The fluorescence intensity in atprmt5-2 atlcd plants resembles that of the atlcd mutant. The mutants atprmt5-2 atlcd and atlcd showed weaker fluorescence signals than in atprmt5-2 but stronger fluorescence signals than in WT (Figure 4, C and D). To further examine the degree of membrane damage in these plants under Cd2+-stress conditions, the malondialdehyde (MDA) content was determined. In general, the higher the MDA content, the higher the membrane damage degree. Similar to the fluorescent probe assay results, we found that Cd2+-stress treatments resulted in a significantly increased MDA level in atprmt5-2, as well as slightly increased MDA levels in atlcd and atprmt5-2 atlcd plants in comparison with WT. The MDA content in atprmt5-2 atlcd plants resembled that in the atlcd mutant (Figure 4E).
We then examined the expression levels of genes related to Cd2+ detoxification in WT, atlcd, atprmt5-2, and atprmt5-2 atlcd plants. Glutathione (GSH) was synthesized by G-glutamylcysteine synthetase and GSH synthetase (Seth et al., 2012). GSH was used as a substrate, and it is catalyzed by phytochelatin (PC) synthase (PCS) to produce PCs (Grill et al., 1989; Thangavel and Long, 2007). PCs bind with cytoplasmic Cd2+ to form PC–Cd complexes, which play critical roles in Cd2+ detoxification. Thus, Cd2+ stress upregulated the expression of AtGSH1, AtGSH2, and AtPCS1. However, the up-regulated gene expression trend was weak in atlcd, atprmt5-2, and atprmt5-2 atlcd mutants compared with that in WT under Cd2+-stress conditions. The induced expression patterns of these genes in atprmt5-2 atlcd were more similar to those in atlcd than in atprmt5-2 plants (Figure 4, F–H).
AtPRMT5 regulation of Cd2+ tolerance partially depends on H2S
Does AtPRMT5 improve Cd2+ tolerance depending on H2S? To answer this question, we overexpressed AtPRMT5 in the atlcd background, which is defective in H2S production (Figure 5A). WT, atlcd, AtPRMT5-OE, AtPRMT5-OE1/atlcd, and AtPRMT5-OE2/atlcd plants were cultured on 1/2 MS medium with or without 80 μM CdCl2 for 14 days to assess Cd2+ tolerance. The root lengths of AtPRMT5-OE1/atlcd and AtPRMT5-OE2/atlcd seedlings in medium containing 80 μM CdCl2 were inhibited significantly compared with roots of AtPRMT5-OE plants, but they were still longer than those of atlcd plants (Figure 5, B and C). Thus, we speculated that AtPRMT5 ability to enhance Cd2+ tolerance in plants was partly dependent on AtLCD.

AtPRMT5’s regulation of Cd2+ tolerance in plants partly depends on H2S. A, Expression analyses of AtPRMT5 in WT, AtPRMT5-OE1/atlcd, and AtPRMT5-OE2/atlcd plants as assessed by RT-qPCR. Error bars represent SEs, n = 3. B and C, An analysis of the Cd2+ tolerance of AtPRMT5-OE plants in an atlcd background (AtPRMT5-OE/atlcd). B, Phenotypes of WT, atlcd, AtPRMT5-OE, AtPRMT5-OE1/atlcd, and AtPRMT5-OE2/atlcd seedlings grown on 1/2 MS medium with (bottom panels) or without (top panels) 80 μM CdCl2 for 14 days. Scale bar, 2 cm. C, Root lengths of seedlings at 14 days. Error bars represent SEs, n ≥ 12. D–G, Analysis of the Cd2+ tolerance of AtPRMT5-OE seedlings in the presence of AOA or HT. D and E, Phenotypes of AtPRMT5-OE1 seedlings grown on 1/2 MS medium containing 80 μM CdCl2 in the absence (left panels) or presence (right panels) of 50 μM AOA (D) or 100 μM HT (E) for 14 days. Scale bar, 1 cm. F and G, Root lengths of seedlings at 14 days. Error bars represent SEs, n ≥ 10. H and I, The alleviation effects of H2S on WT, atprmt5-1, and atprmt5-2 plants under Cd2+-stress conditions. Here, 7-day-old seedlings grown on 1/2 MS medium were fumigated with 5 μM NaHS or not fumigated. The seedlings were then transferred to medium with or without 80 μM CdCl2 for 72 h. Scale bar, 0.5 cm. The hook lengths of the roots were measured after 72 h. Error bars represent SEs, n ≥ 10. All the data are means ± se. Experiments were performed three times with similar results. Different letters indicate significant differences (one-way ANOVA test, P < 0.05). * and ** represent significant difference (P < 0.05) and extremely significant difference (P < 0.01), respectively.
To further provide evidence that AtPRMT5-mediated AtLCD methylation elevates H2S production to bolster the Cd2+ tolerance in Arabidopsis, root lengths of AtPRMT5-OE1 and AtPRMT5-OE2 seedlings grown on Cd2+ stress medium containing either hypotaurine (HT) or aminooxyacetic acid (AOA) were determined. The half-inhibition concentrations of AOA and HT were screened for in preliminary experiments (Supplemental Figures S7 and S8). The root lengths of AtPRMT5-OE1 and AtPRMT5-OE2 seedlings were significantly inhibited when grown in medium supplemented with either HT or AOA (Figure 5, D–G and Supplemental Figure S9). Thus, the reduction in H2S significantly weakened the promotive role of AtPRMT5 in increasing the Cd2+ tolerance of plants.
Furthermore, we performed root-tip bending experiments. We used 5 μM NaHS solution (H2S donor, Zhao et al., 2001) to fumigate WT and atprmt5 plants and then transferred the seedlings to medium containing 80 μM CdCl2 for 72 h. Afterward, the hooks of the roots were observed and measured. H2S significantly reduced the inhibitory effects of Cd2+ on seedling root tips in WT and the atprmt5 mutants (Figure 5, H and I). These pharmacological experiments suggested that H2S played a critical role in the process of AtPRMT5-mediated Cd2+ tolerance.
Discussion
In this study, we propose a mechanism by which H2S signaling is enhanced by the protein methylation of AtLCD at Arg-83 mediated by AtPRMT5 during Cd2+ stress responses in Arabidopsis (Figure 6).
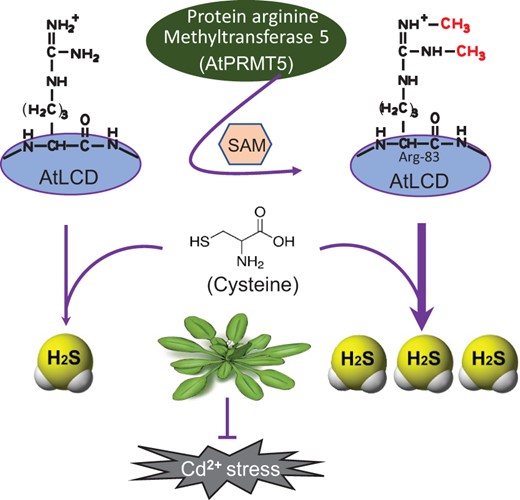
A model of AtPRMT5 regulation of AtLCD activity that results in H2S production after exposure to Cd2+ in Arabidopsis. AtPRMT5-mediated methylation of AtLCD at Arg-83 increases the AtLCD activity and H2S production, which strengthens Cd2+ tolerance.
PRMT5 was involved in the regulation of plant growth and development, multiple stress responses, and the regulation of gene expression mainly by affecting histone methylation. Based on our previous research and other reports, the level of H4R3sme2 in the promoter of AtFLC decreases in the atprmt5 mutant, which in turn increases AtFLC expression and regulates flowering in Arabidopsis (Pei et al., 2007; Wang et al., 2007; Schmitz et al., 2008). The repressed shoot regeneration phenotype of the atprmt5 mutant is caused by the decrease in H4R3sme2 in the AtKRP promotor region, which results in upregulated AtKRP expression (Liu et al., 2016a). Similar AtPRMT5 roles have been found in normal shoot apical meristem growth maintenance (Yue et al., 2013), proline accumulation (Fu et al., 2018), Ca2+ signaling responses (Fu et al., 2013), and root stem cell maintenance (Li et al., 2016). Furthermore, PRMT5-mediated methylation of the small nuclear ribonucleoprotein components of Sm protein and spliceosome complex LSM4 regulates mRNA alternative splicing, which affects growth-, development-, and stress-responsive genes in plants (Meister et al., 2001; Deng et al., 2010). This strongly implied that histone methylation modifications mediated by AtPRMT5 have evolutionarily conserved functions in regulating different biological processes. According to the literature, PRMT5 regulates diverse biological processes mainly through histone and pre-mRNA splicing-related protein (Sanchez et al., 2010; Zhang et al., 2011; Liu et al., 2016a). To date, there have been no reports that PRMT5 uses enzymatic proteins as direct substrates to regulate its activity.
In this study, an interaction between AtLCD and AtPRMT5 in response to Cd2+ stress was identified (Figure 1). The H2S content of atprmt5 mutant plants decreased (Figure 2, A–C), but the transcriptional and translational levels of AtLCD did not substantially change (Figure 2, D–F). Therefore, we have speculated that AtPRMT5 uses AtLCD as a substrate and regulates its activity through methylation modifications. The result showed that AtPRMT5 has the ability to methylate enzymatic proteins and regulate their activities, in addition to methylating histone and spliceosome-related protein. This is a previously uncharacterized pathway through which AtPRMT5 can regulate plant physiological processes and environmental responses.
H2S participates in the regulation of Cd2+ tolerance in plants. Exogenous H2S enhances the tolerance to Cd2+ by affecting antioxidant enzymes, ROS, and the absorption and transport of heavy metal ions (Sun et al., 2013; Ali et al., 2014; Mostofa et al., 2015). However, the regulatory mechanisms involved in endogenous H2S generation are still poorly understood. Cd2+ stress induces the expression of AtLCD and the production of H2S (Zhang et al., 2015; Qiao et al., 2016). However, the regulatory mechanism is still unknown. Because LCD is crucial for H2S generation, the regulation of its expression and activity has attracted great interest. Here, we showed that the AtPRMT5-mediated methylation of AtLCD increased its activity and H2S production, thus bolstering Cd2+ tolerance in Arabidopsis. This represents an important pathway for the regulation of endogenous H2S generation.
l- and d-Cys can be used as substrates and are catalyzed by LCD and DCD, respectively, to generate H2S in plants. The l-type amino acids are the main forms in plants, whereas the d-type free amino acids represent only 0.5%–3% of the former. The concentration of l-Cys is 10 μM in plant cells, whereas the concentration of d-Cys is too low to be accurately determined (Riemenschneider et al., 2005). Purified protein BL21 (LCD) degrades l-Cys to generate H2S, with a H2S-production rate that is 1.07 times that of the control, whereas purified protein BL21 (DCD) degrades d-Cys into H2S, with a H2S-production rate this is 7.2 times that of the control. Thus, DCD exhibits a higher catalytic activity compared with LCD (Shen et al., 2012). Because of the lower d-Cys content, whether DCD could decompose d-Cys to H2S in plant cells has been ignored. The posttranslational modifications of DCD will be explored in a future study.
Here, we demonstrated that AtLCD acts downstream of AtPRMT5 in response to Cd2+ stress in Arabidopsis. The Cd2+ sensitivity of the atprmt5-2 atlcd double-mutant plants was similar to that of the atlcd single mutant (Figure 4, A and B). In addition, the lower Cd2+ tolerance of AtPRMT5-OE/atlcd plants compared with that of AtPRMT5-OE plants further confirmed the conclusion (Figure 5, B and C). However, the Cd2+ sensitivity of AtPRMT5-OE/atlcd plants was comparable with that of WT and was lower than that of the atlcd mutant. Consequently, we speculated that AtPRMT5 partly depends on the H2S pathway to increase Cd2+ tolerance in plants.
AtPRMT5 and AtLCD affected the expression levels of genes involved in Cd2+ detoxification. Under Cd2+-stress conditions, the expression levels of AtGSH1/2, key genes of GSH synthesis, were significantly lower in the atlcd and atprmt5 atlcd mutant plants than in WT. The same trend was found for AtPCS1 expression (Figure 4, F–H). These data supported that AtPRMT5 acts upstream of AtLCD in the regulation of PC biosynthesis. Moreover, we further examined the Cd2+ contents in the mutant lines. The atlcd and atprmt5-2 atlcd mutant plants had higher Cd2+ contents than the WT, but they had lower Cd2+ contents than the atprmt5 mutant (Figure 4, C and D). In addition, the atprmt5 mutant exhibited a stronger Cd2+ tolerance than atlcd and atprmt5 atlcd mutant plants (Figure 4, A and B). We speculated that cytoplasmic Cd2+ in the atprmt5 mutant might be transported to the vacuole by metal ion transporters, thereby enhancing Cd2+ tolerance in plants. These data also supported a role for H2S signaling downstream of AtPRMT5.
AtPRMT5 is an activation regulator of AtLCD in Arabidopsis. The AtPRMT5-mediated methylation of AtLCD increases its activity and enhances H2S production, thereby improving Cd2+ tolerance. Our study provides further insights into the biological functions of AtPRMT5 in plants and the study of Arg methylation. In addition, we present a mechanism for endogenous H2S production and establish a link between methylation modifications and the regulation of Cd2+ tolerance in plants.
Materials and methods
Plant materials and growth conditions
The genetic background of Arabidopsis (Arabidopsis thaliana) used in this study was Colombia-0. The T-DNA insert mutants atprmt5-1 (SALK_065814) and atprmt5-2 (SALK_095085) were used. Seeds of atlcd (SALK_082099) were ordered from the Arabidopsis Biological Resource Center (http://www.arabidopsis.org/abrc/) and had been characterized previously (Fang et al., 2017). 35S::AtLCD transgenic plants were constructed in our laboratory. We identified and obtained atprmt5-2 atlcd homozygous double-mutants through crosses. Further details can be found in the Supplemental Data.
Seeds were disinfected in 75% (v/v) ethanol for 30 s, followed by 6% (v/v) NaClO for 10 min. Finally, seeds were washed five times with sterile water, for 5 min each time. They were sown in 1/2 MS medium with or without 80 μM CdCl2 (1% [w/v] sucrose and 1% [w/v] agarose, pH 5.8), then vernalization occurred at 4°C over 3 days under dark conditions. Root lengths of seedlings grown in a greenhouse (at 23°C and 60% relative humidity, with 16-h/8-h light/dark and 160 μE·m−2·s−1) were measured after 14 days.
To evaluate the effect of sodium hydrosulfide (NaHS, a H2S donor, Zhao et al., 2001), HT (a H2S scavenger, Ortega et al., 2008), and AOA (a Cys desulfhydrase inhibitor, Bianca et al., 2009) on Cd2+ sensitivity, 14-day-old seedlings were sown on 1/2 MS medium containing Cd2+ in the absence or presence of HT or AOA. Seedlings root lengths were recorded after 14 days. For the root tip bending experiment, 7-day-old seedlings were transferred to medium containing 5 μM NaHS for 24 h, and then, they were exposed to 80 μM CdCl2 for 72 h. Seedling root lengths were measured.
Plant expression vector construction and generation of transgenic plants
The coding DNA sequence of AtPRMT5 was amplified from Arabidopsis cDNA. The obtained cDNA fragment was ligated into the BamHI and EcoRI sites of XF348, which was provided by Prof. Xiaofeng Cao, to generate the XF348-AtPRMT5 expression vector. Agrobacterium strain GV3101, carrying XF348-AtPRMT5, was introduced into WT and atlcd plants by floral dipping (Clough and Bent, 1998). The T3 generation of homozygous plants obtained was used for further experiments. All the primers used in this study are listed in Supplemental Table S1.
Expression and purification of recombinant proteins
The coding region of AtLCD was amplified and ligated into pET28a vector (Novagen, Malaysia). The coding region of AtPRMT5 was amplified and inserted into pCold-TF (TaKaRa, Japan) and pGEX-4T-1 vector (GE Healthcare, USA), respectively. For site-mutated AtLCD, primers with corresponding mutated sites were designed. Recombinant plasmid pET28a-LCD was used as the template for PCR amplification and primers used are listed in Supplemental Table S1. The above PCR products were used for bridge PCR reaction to obtain AtLCDArg83Ala. Then AtLCDArg83Ala gene was ligated into pET28a vector. Constructed plasmids were successfully transformed into BL21 (DE3) to express fusion proteins. The proteins were purified using Ni-NTA Seflnose Resin (Sangon, China) or GST-Sefinose Resin in accordance with the manufacturer’s instructions.
BiFC and subcellular localization assay
BiFC methods have been described previously in detail (Walter et al., 2004). Briefly, to perform subcellular localization and BiFC assays, coding sequences of AtLCD and AtPRMT5 were amplified and cloned independently into the pMDC43 Gateway vector, provided by Liyu Huang (School of Agriculture, Yunnan University), as well as the pnYFP and pcCFP Gateway vectors, provided by Yongfu Fu (Institute of Crop Science, Chinese Academy of Agricultural Sciences). The constructed plasmids were transformed into EHA105 and co-transformed into the leaves of 3-week-old Nicotiana benthamiana (N. benthamiana) leaves were cultured under the conditions of 16-h/8-h light/dark for 72 h in a greenhouse after infiltration. Fluorescence signals were detected and photographed using confocal laser scanning microscopy (ZEISS, Germany). Excitation and emission wavelengths of GFP are 488/493 to 598 and those of RFP are 561/595 to 670. AHL22-RFP showed nuclear localization (Xiao et al. 2009). The N. benthamiana leaves were transformed with nYFP-AtPRMT5/cCFP-GUS and nYFP-AtLCD/cCFP-GUS as negative controls.
Pull-down assay
The 14-day-old Arabidopsis seedlings were ground in liquid nitrogen and suspended in 1 mL extraction buffer (containing 50 mM PBS pH 7.0, 150 mM NaCl, 0.1% Triton X-100, 1 mM PMSF, and 1× proteinase inhibitor cocktail). Protein extracts of WT plants were centrifuged at 12,000 g at 4°C for 30 min for later experiments. The expression and purification of the His-TF-AtPRMT5 protein were performed with Ni-NTA agarose beads (Sangon, China) in a 10-mM HEPES buffer using an ultrafiltration device. After ultrafiltration, the His-TF-AtPRMT5 recombinant protein was bound with pre-treated Ni-NTA agarose beads for 1 h. The unbound proteins were removed by washing three times with solution A (20 mM Tris–Cl, pH 7.5), and then, protein extracts of WT were incubated with Ni-NTA agarose beads at 4°C for 20 min. The beads were collected and washed three times with washing buffer (20 mM Tris–Cl, pH 7.0, 0.5 mol/L NaCl) to remove unbound proteins. The resuspended immunoprecipitates were separated using 12% SDS-PAGE and exposed to an anti-AtLCD antibody (2,000×).
Co-IP assay
The Co-IP assay was performed in accordance with previous methods (Shang et al., 2010). The 14-day-old Arabidopsis seedlings were ground in liquid nitrogen and suspended with 1 mL extraction buffer (containing 50 mM PBS pH 7.0, 150 mM NaCl, 0.1% Triton X-100, 1 mM PMSF, and 1× protease inhibitor cocktail). The protein extracts of WT were centrifuged at 12,000 g at 4°C for 30 min. After pre-incubation with protein G, an anti-AtLCD antibody was added and the samples were rotated gently at 4°C overnight. Then, protein G was added at 4°C for 3 h. The beads were collected and washed three times with washing buffer (PBS pH 7.0, 150 mM NaCl, and 0.1% Triton X-100). The precipitates were separated using 12% SDS-PAGE and exposed to an anti-AtLCD antibody (2,000×) or anti-AtPRMT5 antibody (5,000×).
Determination of H2S contents
The H2S contents of 14-day-old Arabidopsis seedlings grown in 1/2 MS medium were assayed in accordance with previous methods (Fang et al., 2017). In addition, the H2S fluorescence probe (Sigma-Aldrich) was used to determine the H2S contents of 14-day-old seedlings. Seedlings were incubated in 20 mM HEPES-NaOH buffer (pH 7.5) containing 10 μM AzMc probes for 20 min. Then, seedlings were washed three times with 20 mM HEPES for 15 min each time. Fluorescence signals were observed using a fluorescence microscope, and fluorescence intensities were analyzed using ImageJ2x (Rawak Software Inc., Stuttgart, Germany). Samples were excited at 360–370 nm and emission was recorded at 420 nm.
Determination of AtLCD activity
The AtLCD activity levels were determined in accordance with previously reported methods (Fang et al., 2017).
Analysis of methyltransferase activity in vitro
The determination of methyltransferase activity was performed as described previously (Pei et al., 2007). Recombinant plasmid GST-AtPRMT5 was transformed into BL21 (DE3) to express the fusion protein. Then, purified GST-AtPRMT5 protein and His-AtLCD protein were incubated with methyl donor S-adenosyl-l-[methyl-3H] Met (Amersham Biosciences, USA) in HMT buffer (20 mM Tris–HCl, pH 8.0, 4 mM EDTA, 1 mM PMSF, and 0.5 mM DTT) at 30°C for 1–3 h. The proteins were separated using 12% SDS-PAGE and Coomassie bright blue staining for visualization. Gels were dried and exposed to Kodak Biomax MS film.
The purified proteins His-TF-AtPRMT5 and His-AtLCD were treated with methyl donor SAM (Solarbio, China) in HMT buffer at 30°C for 2 h. The reaction mixtures were used for the determination of AtLCD activity.
Liquid chromatograph/mass spectrometry/mass spectrometry analysis
Purified His-AtLCD protein was treated with His-TF-AtPRMT5 protein at 30°C for 2 h in the presence of the methyl donor SAM. After the addition of six volumes of 10% trichloroacetic acid/acetone overnight, the proteins were precipitated by centrifugation at 4°C and 14,000 g for 10 min. The precipitates were washed with 100% acetone and resuspended in 8 M urea in 100 mM Trizma base buffer (pH 8.0). Then, the proteins were reduced, alkylated, and digested with trypsin using the FASP method (Wiśniewski et al., 2009). Tryptic peptides were redissolved in 0.1% formic acid and then, an approximately 200-µg sample was injected into an ACQUITY UPLC M-class high-performance liquid chromatography system (Waters, USA) that was online with an Orbitrap Fusion Lumos (Thermo Fisher Scientific, USA). The sample was loaded directly onto the analytical column (nanoEase M/Z HSS C18, 75 μm × 25 cm, Waters) using a mobile phase buffer that consisted of 0.1% formic acid in water (buffer A) and an eluting buffer of 0.1% formic acid in 100% acetonitrile (v/v, buffer B). The gradient was set as 4%–7% buffer B for 3 min, 7%–20% for 20 min, and 20%–30% for 11 min at 600 nL min−1. The peptides were ionized when the spray voltage was 2.2 kV and then, they entered the mass spectrometry system. The mass spectrometry was in a data-dependent acquisition mode and switched automatically between mass spectrometry and mass spectrometry/mass spectrometry acquisition on a 3-s cycle.
All the raw data were analyzed using Proteome Discoverer 2.2 (Thermo Fisher Scientific) with the protein sequences of AtLCD and AtPRMT5. The false discovery rates of proteins and peptides were set to 0.01, and the minimum and maximum lengths of peptides were 6 and 144, respectively. The methylation modification of Cys was designated as the fixation modification, whereas the methionine oxidation and Arg methylation were considered variable modifications. A decoy database search was employed to generate high (P < 0.01) and medium (P < 0.05) confidence peptide lists.
Measurement of MDA
The MDA content was assayed in accordance with previously published methods (Zhang et al., 2015). Arabidopsis seedlings were homogenized with 5% trichloroacetic acid. The mixtures were centrifuged at 8,000 g for 10 min. The supernatants were mixed with 0.67% thiobarbituric acid. The mixtures were heated at 100°C for 30 min. After centrifugation at 8,000 g for 5 min, the absorbance of the supernatant was measured at 450, 532, and 600 nm.
Analysis of gene expression
The total RNAs from seedlings were extracted using TRIzol (TakaRa, Japan). Reverse transcription reactions were carried out using 5× All-In-One RT MasterMix (abm, China) in accordance with the instruction manual. Reverse transcription quantitative PCR (RT-qPCR) analysis was performed using an EvaGreen Kit (Biotium, USA). The relative expression levels of target genes were calculated using the 2−ΔΔCt method (Livak and Schmittgen, 2001). AtACT2 (AT3G18780) was the internal reference for RT-PCR and RT-qPCR. For each experiment, at least three independent biological and technical repeats were performed.
Analysis of Cd2+ content using Cd2+-specific fluorescent probes
At 10 days after germination, Arabidopsis seedlings of different genotypes were transferred to 1/2 MS medium with or without 80 μM CdCl2 for 5 days. Seedlings were incubated with 0.5% LeadmiumTM Green AM dye (Molecular Probes, Invitrogen, Carlsbad, California, USA) for 1 h at 37°C. Seedlings were washed with washing buffer (0.85% NaCl) three times for 5 min each time. Fluorescence signals were observed under a fluorescence microscope equipped with a specific filter set (excitation at 488 nm and emission at 500–517 nm). The mean fluorescence intensity was quantified using ImageJ2x (Rawak Software Inc.). The experiments were repeated twice.
Statistical analysis
A one-way ANOVA was used for determining statistical significance. Data shown in experiments are mean averages ± standard errors (SEs). * and ** represent significant differences (P < 0.05) and extremely significant differences (P < 0.01), respectively. All the data were analyzed using SPSS (version 17, IBM SPSS, USA).
Accession numbers
Sequence information used in this study was downloaded from the Arabidopsis Information Resource Centre (https://www.arabidopsis.org/). The accession numbers are as follows: AtLCD (AT3G62130), AtPRMT5 (AT4G31120), AtPCS1 (AT5G44070), AtGSH1 (AT4G23100), and AtGSH2 (AT5G27380).
Supplemental data
The following materials are available in the online version of this article.
Supplemental Figure S1. The identification of atlcd, atprmt5-1, and atprmt5-2 mutants.
Supplemental Figure S2. The analysis of DNA identification and gene expression in AtLCD-OE, AtPRMT5-OE plants.
Supplemental Figure S3. The effect of Cd2+ on seedlings grown on 1/2 MS medium.
Supplemental Figure S4. The purification and separation of recombinant proteins.
Supplemental Figure S5. Amino acid sequence and mass spectrometry analysis of AtLCD protein.
Supplemental Figure S6. DNA identification of atprmt5 atlcd double mutant.
Supplemental Figure S7. The effect of AOA on seedlings grown on 1/2 MS medium containing 80 μM CdCl2.
Supplemental Figure S8. The effect of HT on seedlings grown on 1/2 MS medium with 80 μM CdCl2.
Supplemental Figure S9. The analysis of Cd2+ tolerance of AtPRMT5-OE2 seedlings in the presence of AOA or HT.
Supplemental Table S1. Primers used in this study.
Acknowledgments
We are grateful to Dr Yongfu Fu (Institute of Crop Science, Chinese Academy of Agricultural Sciences) for providing the BiFC vectors pnYFP-X and pcCFP-X, Dr Liyu Huang (School of Agriculture, Yunnan University) for providing the pMDC43 Gateway vector, and Dr Liang Yang (Westlake Omics [Hangzhou] Biotechnology, Zhejiang Province, China) for mass spectrometric analysis.
Funding
This work was supported by the National Natural Science Foundation of China (31972428 to Yanxi Pei, 32172550 to Zhuping Jin).
Conflict of interest statement. The authors declare that they have no conflict of interest.
Y.P., Z.J., and X.D. conceived and directed the project. H.C., L.Z., Y. L., and Z.L. performed the experiments. H.C., Y.L., and D.L. analyzed the data. Y.P., Z.J., X.D., and H.C. wrote the manuscript. X.C. supervised the project and revised the manuscript. All authors read and approved the final manuscript.
The author responsible for distribution of materials integral to the findings presented in this article in accordance with the policy described in the Instructions for Authors (https://dbpia.nl.go.kr/plphys/pages/general-instructions) is: Yanxi Pei ([email protected]).