-
PDF
- Split View
-
Views
-
Cite
Cite
Ren-Jie Tang, Yang Yang, Yu-Wei Yan, Dan-Dan Mao, Hong-Mei Yuan, Chao Wang, Fu-Geng Zhao, Sheng Luan, Two transporters mobilize magnesium from vacuolar stores to enable plant acclimation to magnesium deficiency, Plant Physiology, Volume 190, Issue 2, October 2022, Pages 1307–1320, https://doi.org/10.1093/plphys/kiac330
- Share Icon Share
Abstract
Magnesium (Mg) is an essential metal for chlorophyll biosynthesis and other metabolic processes in plant cells. Mg is largely stored in the vacuole of various cell types and remobilized to meet cytoplasmic demand. However, the transport proteins responsible for mobilizing vacuolar Mg2+ remain unknown. Here, we identified two Arabidopsis (Arabidopsis thaliana) Mg2+ transporters (MAGNESIUM TRANSPORTER 1 and 2; MGT1 and MGT2) that facilitate Mg2+ mobilization from the vacuole, especially when external Mg supply is limited. In addition to a high degree of sequence similarity, MGT1 and MGT2 exhibited overlapping expression patterns in Arabidopsis tissues, implying functional redundancy. Indeed, the mgt1 mgt2 double mutant, but not mgt1 and mgt2 single mutants, showed exaggerated growth defects as compared to the wild type under low-Mg conditions, in accord with higher expression levels of Mg-starvation gene markers in the double mutant. However, overall Mg level was also higher in mgt1 mgt2, suggesting a defect in Mg2+ remobilization in response to Mg deficiency. Consistently, MGT1 and MGT2 localized to the tonoplast and rescued the yeast (Saccharomyces cerevisiae) mnr2Δ (manganese resistance 2) mutant strain lacking the vacuolar Mg2+ efflux transporter. In addition, disruption of MGT1 and MGT2 suppressed high-Mg sensitivity of calcineurin B-like 2 and 3 (cbl2 cbl3), a mutant defective in vacuolar Mg2+ sequestration, suggesting that vacuolar Mg2+ influx and efflux processes are antagonistic in a physiological context. We further crossed mgt1 mgt2 with mgt6, which lacks a plasma membrane MGT member involved in Mg2+ uptake, and found that the triple mutant was more sensitive to low-Mg conditions than either mgt1 mgt2 or mgt6. Hence, Mg2+ uptake (via MGT6) and vacuolar remobilization (through MGT1 and MGT2) work synergistically to achieve Mg2+ homeostasis in plants, especially under low-Mg supply in the environment.
Introduction
Magnesium (Mg2+) serves as a counter cation for nucleotides such as ATP and an essential cofactor for many enzymes in all living cells (Shaul, 2002; Hermans et al., 2013). Being positively charged, Mg2+ cannot diffuse freely across membranes and requires specific transport proteins to enter cells and further distribute into various organelles. Mg2+ transport is best understood in prokaryotes that contain several different classes of transporters (Snavely et al., 1989). Among them, COBALT RESISTANCE A (CorA) proteins represent a major Mg2+ transport system in bacteria (Smith and Maguire, 1998). The crystal structure of a CorA protein reveals a pentameric cone-shaped channel (Eshaghi et al., 2006; Lunin et al., 2006). CorA channel activity is regulated by a negative feedback loop, in which low Mg2+ concentrations promote the transition of channel to an open state while Mg2+ binding at the inter-subunit interfaces leads to channel closure (Pfoh et al., 2012; Dalmas et al., 2014).
Homologs of CorA-type Mg2+ transporters are ubiquitously found in eukaryotes including fungi, animals as well as plants. In yeast (Saccharomyces cerevisiae), two CorA-like transporters, ALR1 and ALUMINUM RESISTANCE 1 and 2 (ALR2), are localized to the plasma membrane and function in Mg2+ uptake (Graschopf et al., 2001). Another CorA-type protein in yeast, MITOCHONDRIAL RNA SPLICING 2 (MRS2), targeted to the inner membrane of mitochondria, facilitates Mg2+ influx to the mitochondrial matrix and is necessary for proper splicing of Group II introns, thus enabling yeast to grow under nonfermentable conditions with glycerol as the main carbon source (Kolisek et al., 2003). The fourth CorA homolog, MANGANESE RESISTANCE 2 (MNR2), resides in the vacuolar membrane of yeast cells and plays a critical role in supporting yeast growth through Mg2+ remobilization (Pisat et al., 2009). A family of CorA-type proteins function as Mg2+ transporters (MGTs) in plants such as Arabidopsis (A. thaliana) (Li et al., 2001). Based on the functional complementation of the yeast mrs2Δ mutant, the same family of transporters is also termed as “MRS2s” (Schock et al., 2000). Several members of plant MGT/MRS2s family have been shown to exhibit Mg2+ transport activity in bacteria or yeast cells, although some MGT members may transport other cations in addition to Mg2+, which is exemplified by MGT1/MRS2-10 that delivers Ni2+ and Al3+ into the proteoliposome in vitro (Ishijima et al., 2018). Genetic studies have further unraveled some of their physiological roles in plants. For example, Arabidopsis MGT4, MGT5, and MGT9 are preferentially expressed in mature anthers and accordingly they function in male gametophyte development (Li et al., 2008; Chen et al., 2009; Li et al., 2015; Xu et al., 2015). Among all the MGT members in Arabidopsis, MGT6 appears to be a central player that functions in Mg2+ acquisition and homeostasis, as mgt6 single mutants display dramatic growth defects under both low and high external Mg2+ concentrations (Oda et al., 2016; Yan et al., 2018). It has been established that MGT6 mediates high-affinity Mg2+ uptake in the root when soil Mg2+ levels fall in the sub-millimolar range (Mao et al., 2014). As a root-specific member, MGT7 functions redundantly with MGT6 in sustaining plant growth and survival during Mg2+ starvation (Gebert et al., 2009; Oda et al., 2016; Yan et al., 2018). Importantly, MGT6 and MGT7 are also required for plant tolerance to high-Mg conditions, highlighting their essential roles in maintaining Mg2+ homeostasis at the whole-plant level (Yan et al., 2018). Intriguingly, Mg2+ uptake by MGT-type transporters across the plasma membrane plays a positive role in plant adaptation to other abiotic stresses. For instance, OsMGT1 in rice (Oryza sativa) is critical for tolerance to Al3+ stress, presumably because efficient Mg2+ acquisition facilitated by OsMGT1 is beneficial to alleviate Al3+ toxicity (Chen et al., 2012). This transporter is also shown to participate in rice salt tolerance through enhancing the activity of a sodium (Na+) transporter, OsHKT1;5 (HIGH-AFFINITY K+ TRANSPORTER 1;5), which requires Mg2+ as a co-factor for full activation (Chen et al., 2017). Localized to the chloroplast envelope, Arabidopsis MGT10 is required for chloroplast development and photosynthesis (Liang et al., 2017; Sun et al., 2017). A recent study further showed that OsMGT3 in the rice chloroplast exhibits a rhythmic expression pattern and modulates Rubisco activity through Mg2+ loading into the stroma, thereby fine-tuning photosynthesis on a diel basis (Li et al., 2020; Tang and Luan, 2020).
Many plant cells contain a large central vacuole that generates turgor pressure and stores the majority of water and minerals including Mg2+ (Marty, 1999). As the most abundant free divalent cation, the bulk of Mg2+ in plant cells is believed to be compartmentalized into the vacuole, especially when environmental Mg2+ is in excess. The vacuolar sequestration and buffering maintain a relatively stable cytosolic Mg2+ concentration at around 0.2–0.4 mM (Conn and Gilliham, 2010), whereas vacuoles can accumulate large amount of Mg2+ commonly exceeding 25 mM in the mesophyll cells and up to 80 mM in Arabidopsis leaves fed with high-Mg xylem sap solutions (Conn et al., 2011). When plants encounter Mg shortage in the environment, Mg2+ stored in the vacuole must be efficiently retrieved to satisfy the metabolic demands in the cytoplasm (Martinoia et al., 2012). In addition, high-affinity transporters in the plasma membrane of plant cells are activated as a response to nutrient deficiency to acquire more nutrients (Ogura et al., 2018). Thus, uptake transporters in the plasma membrane work together with vacuolar store retrievers for plants to survive the nutrient starvation (Tang et al., 2020). It has been well established that MGT6, residing in the plasma membrane, is responsible for Mg2+ uptake during Mg2+ deficiency (Mao et al., 2014). However, transport proteins in charge of vacuolar Mg2+ efflux remain poorly understood. Here we demonstrate that two tonoplast transporters MGT1 and MGT2 function in vacuolar Mg2+ remobilization. In synergy with plasma-membrane localized MGT6 transporter, they are required to support plant growth under low-Mg conditions.
Results
MGT1 and MGT2 exhibit an overlapping expression pattern in Arabidopsis
Among the Arabidopsis MGT family transporters, MGT1 and MGT2 share about 90% identity in amino acid sequence and their genes may originate from a gene duplication event (Lenz et al., 2013). As an initial step to dissect their function, we analyzed the expression pattern of MGT1 and MGT2 using transgenic plants that expressed β-glucuronidase (GUS) reporter gene driven by their respective promoters. The results revealed a largely overlapping pattern of expression between MGT1 and MGT2 (Figure 1). For example, MGT1 and MGT2 displayed a strong expression in the radicle upon seed germination, and in the cotyledons of 3-d and 5-d old seedlings (Figure 1A). Both genes were ubiquitously expressed in adult organs including roots, leaves, stems, flowers, and siliques, and expression was especially strong in the vacuolar tissues (Figure 1A). Cross-sections of the inflorescence stem indicated that MGT1 and MGT2 were preferentially expressed in the phloem of the vascular bundle (Figure 1A). Notably, the promoter of MGT2 showed stronger activity than that of MGT1 in most cases. RT-qPCR analysis confirmed that the transcript level of MGT2 was consistently higher than that of MGT1 in all organs examined (Figure 1B). We further analyzed MGT1 and MGT2 transcripts in plants grown under different Mg2+ levels, but found no substantial changes, suggesting that these two Mg2+ transporters are not responsive to external Mg2+ at the mRNA level (Supplemental Figure S1). In conclusion, MGT1 and MGT2 are broadly expressed in plants and display an overlapping expression pattern in various tissues and cell types of Arabidopsis, which may suggest functional redundancy of the two transporters.
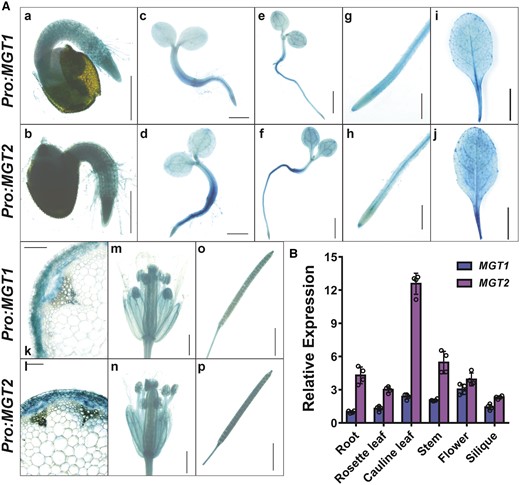
Expression patterns of MGT1 and MGT2 in Arabidopsis. A, GUS expression driven by MGT1 promoter (Pro:MGT1) or MGT2 promoter (Pro:MGT2) in transgenic Arabidopsis plants. Histochemical GUS staining was performed in the seedlings and various adult tissues. (a and b), One-day-old germinating seed, scale bar = 0.5 mm; (c and d) 3-d-old seedling, scale bar = 1 mm; (e and f) 5-d-old seedling, scale bar = 2 mm; (g and h) primary root from 10-d old seedlings, scale bar = 1 mm; (i and j) rosette leaves of 28-d-old plants, scale bar = 10 mm; (k and l) cross-section of an inflorescence stem, scale bar = 0.05 mm; (m and n) flower, scale bar = 1 mm; (o and p) silique, scale bar = 1 mm. B, RT-qPCR analysis of transcript levels of MGT1 and MGT2 in different organs of Arabidopsis plants. The relative expression was double normalized against ACTIN2 (AT3G18780) and the expression level in the root. Data represent means ± SD (n = 4) with four independent experimental results shown as dots and error bars denoting SD.
MGT1 and MGT2 localize to the tonoplast and are functionally equivalent to the vacuolar transporter MNR2 in yeast
We examined the subcellular localization of MGT1 and MGT2 by expressing fluorescence protein (VENUS)-tagged fusions in the mesophyll protoplast followed by confocal imaging. In contrast to an earlier report that localized MGT1 to the plasma membrane (Li et al., 2001), we found that fluorescent signals represented by both MGT1-VENUS and MGT2-VENUS were associated with the membrane of a central vacuole indented by chloroplasts, as well as some smaller compartments near the vacuole (Figure 2A), a pattern of typical tonoplast localization (Tang et al., 2012). The vacuole-associated signals of MGT1-VENUS and MGT2-VENUS were clearly different from those of MGT6-VENUS that were detected in the plasma membrane (Mao et al., 2014; Figure 2A). After isolation of intact vacuoles from protoplasts, the fluorescent signals produced by MGT1 and MGT2 fusions remained associated with the membrane of the central vacuole (Figure 2A). In contrast, no fluorescence signals were detected in the vacuoles isolated from protoplasts expressing either free VENUS or MGT6-VENUS (Figure 2A). These results support the conclusion that MGT1 and MGT2 are localized to the tonoplast.
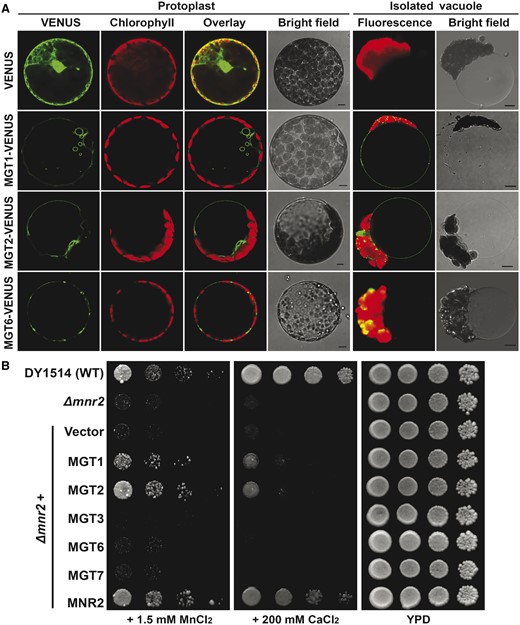
Subcellular localization of MGT1 and MGT2 in the plant cell and functional characterization of MGT1 and MGT2 in the yeast mnr2Δ mutant strain. A, Confocal laser scanning microscopy images of a typical mesophyll protoplast and isolated vacuole transiently expressing fluorescent proteins indicated on the left. In each panel, the VENUS signal, chloroplast fluorescence, overlay, or bright field image from the same cell is shown. Scale bars = 5 μm. B, Complementation test of the yeast mnr2Δ mutant by different MGT transporters. Yeast cells of wild type (DY1514), mnr2Δ, and mnr2Δ transformed with various genes in the p416GPD vector were spotted in YPD (yeast extract, peptone, and dextrose) medium or YPD medium supplemented with 1.5 mM MnCl2 or 200 mM CaCl2 in serial decimal dilutions from left to right. The starting concentration of the first sample on the left is OD600 = 1.0, and therefore from left to right, the concentrations of the four samples represent OD600 = 1.0, 0.1, 0.01, 0.001. Plate cultures were incubated at 28°C and photographed after 3 d. Each experiment was repeated using four independent transformants with similar results.
Previous studies demonstrated that MGT1 and MGT2 exhibit Mg2+ transport activity when expressed in bacteria or targeted to yeast mitochondria (Li et al., 2001; Drummond et al., 2006; Gebert et al., 2009). To address the functional specificity of MGT1 and MGT2 in the tonoplast, we took advantage of the yeast (S. cerevisiae) mutant strain mnr2Δ (Pisat et al., 2009) which lacks the sole CorA homolog in the yeast vacuolar membrane. Because the MNR2 transporter is responsible for vacuolar Mg2+ mobilization, its loss-of-function in the mnr2Δ mutant leads to hypersensitivity of the yeast strain to high levels of Mn2+ and Ca2+ (Pisat et al., 2009), two major antagonistic divalent cations against Mg2+ in cell functions. Given the fact that MGT1 and MGT2 share the same subcellular localization and similar structure as MNR2, we hypothesized that these two plant MGTs may play an equivalent part in vacuolar Mg2+ remobilization as MNR2 in the yeast cell. As a functional assay, we expressed MGT1 and MGT2, individually, in mnr2Δ mutant and found that its sensitivity to high-Mn or high-Ca was effectively suppressed, albeit to a less extent than the complementation by yeast MNR2 (Figure 2B). Meanwhile, the expression of several other MGT members including MGT3, MGT6, and MGT7 failed to rescue the growth defect of mnr2Δ under the same conditions (Figure 2B), although they were able to facilitate Mg2+ translocation into the mitochondrial matrix of the mrs2Δ yeast strain as revealed by complementation of the respiratory deficiency (Supplemental Figure S2; Gebert et al., 2009). Together, these results supported the conclusion that Arabidopsis MGT1 and MGT2, like the yeast MNR2, mediate Mg2+ efflux from the vacuolar store.
Disruption of MGT1 and MGT2 leads to hypersensitivity to low-Mg conditions
To elucidate the physiological function of MGT1 and MGT2 in plant cells, we identified two Arabidopsis T-DNA insertion lines lacking a detectable level of full-length transcripts of MGT1 and MGT2, respectively (Supplemental Figure S3, A and B). Compared with wild-type plants (Col-0), mgt1 or mgt2 single mutants displayed no obvious phenotypic difference during the life cycle or when grown under low-Mg conditions (Supplemental Figure S3, C, D, and E), suggesting that these two transporters might be genetically redundant. The functional redundancy is consistent with their high sequence similarity, transport activity, and overlapping expression pattern in all plant tissues. In a parallel analysis, mgt1 mgt2 double mutants showed a much more pronounced growth defect when Mg2+ was deficient in the culture medium (Figure 3; Supplemental Figure S3). In the presence of 0.01 or 0.05 mM Mg2+, mgt1 mgt2 mutants became more stunted and chlorotic than wild-type plants under the same conditions (Figure 3A), as evidenced by a reduced fresh weight (Figure 3B) and chlorophyll content (Figure 3C). The mgt1 mgt2 seedlings grew better and became comparable to wild type when a higher concentration of Mg2+ (0.1, 0.25, or 1.5 mM) was included in the medium (Figure 3A). Furthermore, under 6 mM Mg2+ (a high-Mg condition), mgt1 mgt2 mutant showed a comparable growth to the wild type (Figure 3A), indicating that MGT1 and MGT2 are not involved in Mg2+ homeostasis under sufficient- or high-Mg conditions.
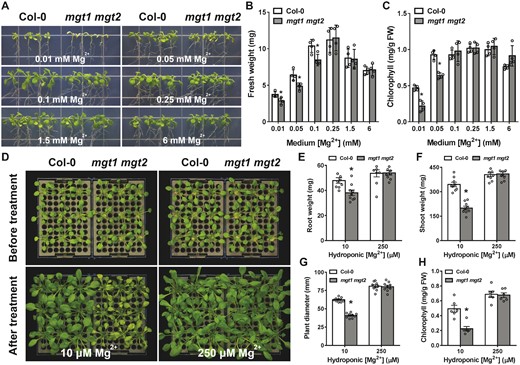
Phenotypic analysis of mgt1 mgt2 double mutant under different external Mg2+ concentrations. A, Growth phenotype of wild type (Col-0) and mgt1 mgt2 on agarose-solidified medium containing different concentrations of Mg2+. Four-day-old seedlings of Col-0 and mgt1 mgt2 germinated on MS medium were transferred onto one-sixth strength MS medium containing indicated concentrations of Mg2+ in each panel. Photographs were taken on the 10th day after the transfer. B and C, Fresh weight (B) and leaf chlorophyll (C) of 14-d-old seedlings as shown in (A). Data represent means ± SE (n = 4). Asterisks indicate statistically significant difference between the Col-0 and mgt1 mgt2 (*P < 0.05 by Student’s t test). D, Phenotype of wild-type (Col-0) and mgt1 mgt2 mutant plants during Mg2+-starvation treatment in the hydroponic assay. Wild-type and mutant plants were grown under Mg-replete conditions (250 µM Mg2+) for 2 weeks and then transferred to new hydroponic solutions containing either low Mg2+ (10 µM) or sufficient Mg2+ (250 µM) for another 14 d. Photographs were taken before the transfer and at the end of 14-d treatment, respectively. E–H, Measurement of root (E) and shoot (F) biomass as well as rosette diameter (G) and leaf chlorophyll (H) of Col-0 and mgt1 mgt2 plants on the 14th day after transferring plants to the new hydroponic solutions. Data represent means ± SE (for E, F, and G, n = 9; for H, n = 6). Asterisks indicate statistically significant difference between the Col-0 and mgt1 mgt2 (*P < 0.05 by Student’s t test).
We next examined whether mgt1 mgt2 plants were affected by elevated concentrations of several other cations in the growth medium. While the mutant and wild-type plants displayed similar growth under most of the conditions, excess of Ca2+ (15 mM) or Sr2+ (8 mM) led to a more severe inhibitory effect on the growth of mgt1 mgt2 (Supplemental Figure S4). Such difference may result from the more closely related chemical properties among Ca2+, Sr2+, and Mg2+, thereby exacerbating Mg2+ deficiency when levels of Ca2+, Sr2+ were elevated in the medium.
To further assess the role of MGT1 and MGT2 in plant adaptation to low-Mg conditions, we transferred adult plants grown in hydroponics from Mg2+-sufficient to Mg2+-deficient conditions. A short period of Mg2+ starvation inhibited growth of mgt1 mgt2 mutants and caused severe leaf chlorosis, a typical symptom for Mg2+ deficiency in plants (Figure 3D). Quantification of the root and shoot biomass as well as chlorophyll content confirmed more severe growth defects of mgt1 mgt2 as compared to the wild type after a 2-week treatment (Figure 3E–H). These results suggested that vacuolar Mg2+ remobilization by MGT1 and MGT2 is critical for plant adaptation to short-term Mg2+ deficiency. Transfer of a genomic fragment containing either the full-length MGT1 or MGT2 gene into mgt1 mgt2 background fully restored plant growth under low-Mg conditions (Supplemental Figure S5), validating that the low-Mg sensitive phenotype of the mutant was attributable to the double knockout of MGT1 and MGT2 and confirming the functional redundancy of MGT1 and MGT2 in Arabidopsis.
The mgt1 mgt2 mutant plants retain high Mg level but exhibit hypersensitive low-Mg response
In order to understand how plant Mg2+ homeostasis is affected by loss-of-function of MGT1 and MGT2, we compared Mg concentrations in different tissues of the wild-type and mgt1 mgt2 plants under varied external Mg2+ conditions. To our surprise, mgt1 mgt2 mutant plants contained a significantly higher Mg concentration than the wild type, particularly in the root tissue under low external Mg2+. When grown on medium containing 0.01 mM Mg2+, mgt1 mgt2 roots accumulated twice as much Mg as wild-type seedlings (Figure 4A). In the medium with 0.25 mM Mg2+ that supported a comparable growth of both the wild type and mutant plants, mgt1 mgt2 also retained a higher Mg concentration in the root than the wild type, albeit a smaller difference was observed than in those seedlings grown on the low-Mg medium (Figure 4A).
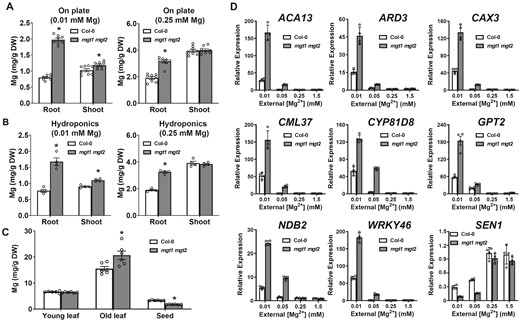
Mg concentration and Mg-starvation marker gene expression in the wild type and mgt1 mgt2 mutant plants. A, Mg concentration in the root and shoot tissues of 2-week-old wild-type (Col-0) and mgt1 mgt2 seedlings grown on the plates containing 0.01 mM (left panel) or 0.25 mM (right panel) Mg2+. Data represent means ± SE (n = 8). B, Mg concentration in the root and shoot tissues of Col-0 and mgt1 mgt2 at the end of the Mg2+-starvation treatment in the hydroponic assay. Data represent means ± SE (n = 4). C, Mg concentration in different tissues of soil-grown Col-0 and mgt1 mgt2 adult plants. Rosette leaves from 3-week-old plants were sampled as a collection of “young leaf” while rosette leaves from 14-week-old plants were sampled as a collection of “old leaf.” Data represent means ± SE (n = 6). From A to C, asterisks indicate statistically significant difference between the Col-0 and mgt1 mgt2 (*P < 0.05 by Student’s t test). D, RT-qPCR analysis of ACA13 (AT3G22910), ARD3 (AT2G26400), CAX3 (AT3G51860), CML37 (AT5G42380), CYP81D8 (AT4G37370), GPT2 (AT1G61800), NDB2 (AT4G05020) WRKY46 (AT2G46400), and SEN1(AT4G35770) in the wild-type (Col-0) and mgt1 mgt2 seedlings grown under different concentrations of external Mg2+. The relative expression of each gene was double normalized against the housekeeping gene ACTIN2 (AT3G18780) and the control expression value measured in Col-0 grown under 1.5 mM Mg2+. Data represent means ± SD (n = 4).
When measuring Mg levels in adult plants grown under hydroponic conditions, we found that lowering external Mg2+ from 0.25 mM to 0.01 mM considerably reduced Mg accumulation in both root and shoot tissues (Figure 4B). Similar to the finding with seedlings on the plate, hydroponic mgt1 mgt2 plants contained higher Mg in the root than the wild type under either low-Mg (0.01 mM) or normal-Mg (0.25 mM) condition, although shoot Mg concentration in the mutant was only elevated during Mg starvation (Figure 4B). Under the same treatments, concentrations of other cationic nutrients such as Ca were not significantly different between mgt1 mgt2 mutants and the wild type (Supplemental Figure S6).
We further analyzed Mg distribution in different tissues of soil-grown plants. While wild-type and mgt1 mgt2 mutant plants retained a similar level of Mg in young leaves, Mg concentration in the old leaves was significantly higher in the mgt1 mgt2 mutant. In parallel, Mg concentration in the seeds was lower in the mutant compared to wild type (Figure 4C). This result implicated a critical role of MGT1 and MGT2 in Mg2+ distribution within the plant grown in the soil. Again, differences in several other mineral nutrients such as Ca, K, and Mn were marginal between the wild-type and mutant plants under the same conditions (Supplemental Figure S7), suggesting that MGT1 and MGT2 function specifically in Mg2+ homeostasis in plants.
The higher Mg concentration in mgt1 mgt2 suggested that, unlike the plasma membrane Mg2+ transporter MGT6, MGT1, and MGT2 are not required for Mg2+ uptake from the external environment. Considering their tonoplast localization, we hypothesized that these two MGT transporters may mediate Mg2+ retrieval from the vacuolar store, consistent with their functional complementation of yeast mnr2Δ mutant impaired in vacuolar Mg2+ efflux. In this scenario, although the mutant plants accumulated more Mg in plant cells (mostly stored in the vacuole), the cytosolic Mg2+ may remain limited as a result of defective Mg2+ retrieval from the vacuolar store. To test this hypothesis, we monitored the expression of Mg-responsive marker genes in the wild-type and mutant seedlings as a proxy for cytoplasmic Mg2+ status. These Mg-starvation markers include genes coding for Ca2+ transporters (CATION EXCHANGER 3, CAX3; AUTOINHIBITED CA2+-ATPASE, ACA13), cell signaling components (CALMODULIN LIKE 37, CML37; WRKY DNA-BINDING PROTEIN 46, WRKY46), and metabolic enzymes (ACIREDUCTONE DIOXYGENASE 3, ARD3; CYTOCHROME P450 FAMILY 81 SUBFAMILY D, CYP81D8; GLUCOSE-6-PHOSPHATE/PHOSPHATE TRANSLOCATOR 2, GPT2; NADH DEHYDROGENASE B2, NDB2), which were previously identified by a genomic approach in Arabidopsis (Kamiya et al., 2012). Under low-Mg conditions, all of the eight representative Mg-starvation gene markers were induced at much higher levels in mgt1 mgt2 than in the wild type, whereas the level of a high-Mg-induced gene SENESCENCE 1 (SEN1) was much lower in the mutant (Figure 4D), confirming that mgt1 mgt2 mutant plants were in fact physiologically more starved for Mg2+ nutrient despite an increased accumulation. Taken together, these results corroborated the physiological role of MGT1 and MGT2 as tonoplast transporters that mediate Mg2+ efflux from the vacuole to the cytoplasm to meet the demand of cytoplasmic Mg2+ in plant cells especially under limited external Mg2+ supply.
MGT1 and MGT2 functionally antagonize vacuolar Mg2+ sequestration
At the cellular level, Mg2+ sequestration into the vacuolar store is an opposite transport process to vacuolar Mg2+ remobilization mediated by MGT1 and MGT2. We previously demonstrated that two tonoplast-associated CALCINEURIN B-LIKE (CBL) proteins and their interacting protein kinases (CBL-INTERACTING PROTEIN KINASES; CIPKs) serve as critical regulators of vacuolar Mg2+ sequestration that are required for detoxification of excessive Mg2+ in plant cells (Tang et al., 2015). Accordingly, the cbl2 cbl3 double mutant is compromised in high-Mg tolerance and displays a hypersensitive phenotype to high-Mg conditions (Tang et al., 2015). Now that we have identified components required for both sequestration and remobilization processes, we tested the genetic interaction of the two by crossing mgt1 mgt2 and cbl2 cbl3 double mutants to produce a quadruple mutant (Figure 5A). When grown in regular soil that contained 50 mg/kg Mg (approximately 2 mM), cbl2 cbl3 mutants were typically stunted during vegetative growth with leaf-tip necrosis as a result of hypersensitive response to high Mg2+, whereas both wild-type and mgt1 mgt2 plants grew well under the same condition (Figure 5B). Notably, the quadruple mutant showed a considerably improved growth than cbl2 cbl3 mutants in the soil (Figure 5B), with less leaf necrosis, larger rosette size, and more biomass (Figure 5, C and D). To demonstrate that the phenotypic suppression of cbl2 cbl3 mutant by mutation of MGT1 and MGT2 resulted from an Mg2+-specific effect, we grew seedlings on agarose plates containing high levels of Mg2+. Indeed, the growth of cbl2 cbl3 mutant plants was greatly inhibited by 4 mM Mg2+ in the medium, but cbl2 cbl3 mgt1 mgt2 quadruple mutants were less stressed under the same condition (Figure 5E). Quantitative analyses of plant fresh weight (Figure 5F) and leaf chlorophyll (Figure 5G) confirmed that the high-Mg sensitive phenotype of cbl2 cbl3 mutants was suppressed by mutations in MGT1 and MGT2. These results further supported that MGT1 and MGT2 mediate Mg2+ efflux from the vacuolar lumen, an antagonistic transport process to Mg2+ sequestration into the vacuole.
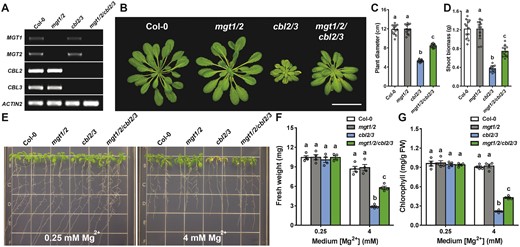
Suppression of the phenotype of cbl2 cbl3 mutant by additional mutations in MGT1 and MGT2. A, RT-PCR analysis of MGT1, MGT2 as well as CBL2 and CBL3 gene expression in wild-type (Col-0), double mutant (mgt1 mgt2, cbl2 cbl3), and quadruple mutant (mgt1/2/cbl2/3) plants. ACTIN2 was analyzed as an internal control. B, Growth phenotype of 4-week-old plants of different genotypes in the soil. Scale bar = 5 cm. C and D, Rosette diameter (C) and shoot biomass (D) of different genotypes as shown in (B). Data represent means ± SD (n = 12). Statistical analyses between different genotypes were performed by one-way ANOVA followed by a Turkey’s multiple comparison test. Different letters indicate significant difference at P < 0.05. E, Growth phenotype of 2-week-old young seedlings of different genotypes on the medium containing 0.25 mM or 4 mM Mg2+. F and G, Seedling fresh weight (F) and leaf chlorophyll (G) of different genotypes as shown in (E). Data represent means ± SE (n = 4). Columns with different letters indicate statistically significant differences performed by one-way ANOVA followed by a Turkey’s multiple comparison test (P < 0.05).
Tonoplast and plasma membrane MGT-type transporters synergistically regulate plant adaptation to low-Mg conditions
Acquisition of external Mg2+ from the environment and Mg2+ retrieval from the internal vacuolar pools are two major routes to meet the cytoplasmic demand for Mg2+ in plant cells. In Arabidopsis, root Mg2+ uptake, particularly under low-Mg conditions, is mediated by plasma membrane-localized MGT6 (Mao et al., 2014). To examine the functional relationship of MGT-type transporters in the plasma membrane and vacuolar membrane for the maintenance of cellular Mg2+ homeostasis, we generated a triple mutant lacking MGT1, MGT2, and MGT6 transcripts (Figure 6A). Under the same growth condition in the soil, the mgt1 mgt2 double mutant was comparable to the wild type, but mgt6 was evidently smaller. The triple mutant mgt1 mgt2 mgt6 was more severely stunted than the mgt6 single mutant (Figure 6, B and C), indicating a functional synergy among the three MGT transporters. We further examined these mutants on the agarose medium supplemented with different concentrations of external Mg2+. Under low-Mg conditions (0.01–0.1 mM), mgt1 mgt2 mgt6 triple mutant indeed displayed more severe growth defects as compared to either mgt6 single or mgt1 mgt2 double mutants (Figure 6D). When medium Mg2+ concentrations reached 0.25–1.5 mM, consistent with earlier results, growth of mgt1 mgt2 became indistinguishable from that of wild type, and mgt6 showed a mild phenotype (Figure 6D). However, under these “optimal” Mg2+ conditions, mgt1 mgt2 mgt6 triple mutant was still visibly stunted (Figure 6D), with shorter primary roots, less fresh weight, and reduced leaf chlorophyll (Figure 6E–G). Furthermore, compared to either mgt1 mgt2 or mgt6, mgt1 mgt2 mgt6 exhibited a significant elevation in the expression level of several Mg2+-starvation marker genes when plants were grown under low-Mg conditions, suggesting an additive defect of the triple mutant in maintaining sufficient cytosolic Mg2+ levels (Figure 6H). These data strongly supported the idea that plasma membrane-localized MGT6 and tonoplast-localized MGT1-MGT2 work synergistically to provide an optimal cellular Mg2+ level required for plant growth.
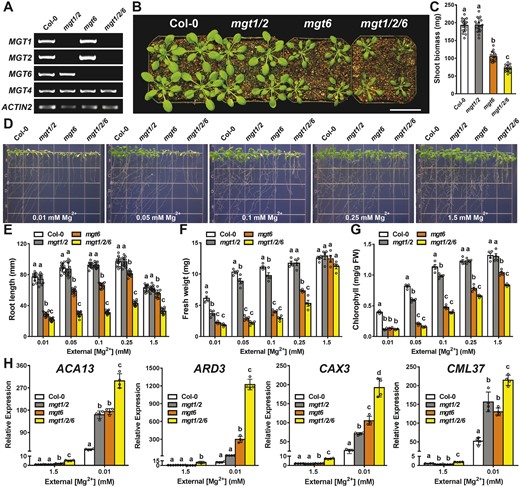
Genetic analysis of the functional synergy of MGT1, MGT2, and MGT6 transporters in Arabidopsis. A, RT-PCR analysis of MGT1, MGT2, and MGT6 gene expression in the wild type (Col-0), mgt1 mgt2, mgt6, and mgt1 mgt2 mgt6 triple mutant. MGT4 (AT3G19640) and ACTIN2 were analyzed as internal controls. B, Growth phenotype of 4-week-old plants of different genotypes in the soil. Scale bar = 5 cm. C, Shoot biomass of different genotypes grown in the soil as shown in (B). Data represent means ± SD (n = 16). Statistical analyses between different genotypes were performed by one-way ANOVA followed by a Turkey’s multiple comparison test. Different letters indicate significant difference at P < 0.05. D, Growth phenotype of 2-week-old young seedlings of different genotypes on the medium containing various concentrations of Mg2+ as indicated. E–G, Measurement of primary root length (E) and seedling fresh weight (F) and leaf chlorophyll (G) of Col-0, mgt1 mgt2, mgt6, and mgt1 mgt2 mgt6 seedlings as shown in (D). For E, data represent means ± SD (n = 12); for (F) and (G), data represents means ± SE of four replicate experiments (n = 4). H, RT-qPCR analysis of ACA13, ARD3, CAX3, and CML37 in Col-0, mgt1 mgt2, mgt6, and mgt1 mgt2 mgt6 seedlings grown on the medium containing 1.5 mM or 0.01 mM Mg2+. Data represent means ± SD (n = 4). For C, E, F, G, and H, statistical analyses between different genotypes were performed by one-way ANOVA followed by a Turkey’s multiple comparison test. Different letters indicate significant difference at P < 0.05.
Discussion
Mg2+ levels in the soil vary, which can impose either toxicity (e.g. the serpentine soil) or deficiency to plants. Therefore, plants have evolved sophisticated transport systems to efficiently acquire, utilize, store, and redistribute Mg2+ (Tang and Luan, 2017). As a highly conserved family of Mg2+ transporters across kingdoms, plant MGTs exhibit Mg2+ transport activity similar to CorA-type homologs in bacteria and yeast. However, the physiological roles of individual MGT members are not fully understood in plants. In this study, we established that MGT1 and MGT2 are associated with the vacuolar membrane and function redundantly in Mg2+ retrieval from the vacuole to the cytoplasm thereby providing a mechanism for plant adaptation to low-Mg conditions. Several lines of evidence supported this conclusion. First, both MGT1 and MGT2 are localized in the tonoplast and functionally complement yeast mutant mnr2Δ that lacks a CorA-type transporter responsible for vacuolar remobilization. Second, Arabidopsis mgt1 mgt2 mutants showed growth retardation, yet retained more Mg under low-Mg conditions than wild-type plants, suggesting that majority of Mg in the vacuolar store is not available for plant metabolism. Third, Mg-starvation marker genes displayed augmented expression levels in mgt1 mgt2, confirming a more severe Mg2+ deficiency status due to the defect in vacuolar remobilization process despite high Mg content stored in the mutant plants.
MGT1 and MGT2 function redundantly in vacuolar Mg2+ retrieval, a housekeeping function that becomes particularly important under Mg-starvation. Such a function is consistent with our finding that both genes were ubiquitously expressed in various tissues and cell types that contain large vacuoles. An earlier study (Gebert et al., 2009) showed that MGT1/MRS2-10 was expressed exclusively in the radicle of young seedlings, but not in cotyledons. This discrepancy may result from different promoter regions employed in the two studies, which is a common concern in analyzing promoter-GUS reporter activity in transgenic plants. Growth conditions and developmental stages of plants are also factors that can cause inconsistent results. For instance, expression of MGT6/MRS2-4 was not detected in the roots (Gebert et al., 2009), but the same gene was found to be strongly expressed when promoter-driven GUS plants were grown under Mg-deficient conditions (Mao et al., 2014). Consistent with this expression pattern, MGT6 resides in the plasma membrane and functions in Mg2+ uptake to cope with low-Mg environments (Mao et al., 2014). Based on the strong expression in mesophyll cells and the subcellular localization in the tonoplast, MGT2 and MGT3 were proposed to function in Mg2+ partitioning into mesophyll vacuoles (Conn et al., 2011), a critical mechanism for detoxifying excessive cellular Mg2+. However, the genetic analysis failed to support such a role for MGT2 and MGT3 in plant tolerance to high-Mg stress (Tang et al., 2015). On the contrary, another study reported that knocking out MGT1, MGT2, and MGT3 reduced plant growth under low-Mg conditions, suggesting that these three MGT members overlap functionally to support plant adaption to low-Mg environments, although the mechanism remained unclear (Lenz et al., 2013). In line with a role in low-Mg adaptation, overexpression of AtMGT1 in Nicotiana benthamiana confers tolerance to Mg2+ deficiency on transgenic plants (Deng et al., 2006). By providing a mechanistic process for MGT1 and MGT2 function, our study here has reconciled differences among previous studies and revealed the molecular basis and physiological importance of vacuolar Mg2+ remobilization in plants.
It is noteworthy that vacuolar remobilization of Mg2+ may be particularly critical for plants to cope with a temporary deprivation of Mg2+, as an external supply must become necessary under long-term Mg2+ deficiency when the vacuolar Mg store is depleted. This idea is consistent with our data showing that mgt6 mutant defective in Mg2+ uptake displayed a more severe growth inhibition than mgt1 mgt2 under Mg2+ deficiency (Figure 6). Furthermore, besides lacking Mg2+ supply, plants can also encounter Mg2+ deficiency when other competitive cations such as Ca2+ and Sr2+ are present in excess because these divalent analogs could antagonize intracellular Mg2+. The finding that mgt1 mgt2 mutant grew poorly under Ca2+ and Sr2+ excess (Supplemental Figure S4) implicates Mg2+ retrieval from the vacuolar pool as a critical mechanism in maintaining an optimal Mg2+ balance under such an ionic stress. Apart from efficient retrieval in response to Mg2+ starvation as a direct compensation mechanism for inadequate uptake, vacuolar Mg2+ store is also important for adjusting the distribution of Mg2+ throughout the whole plant. This is supported by the observation that mgt1 mgt2 mutant accumulated more Mg in the root tissue as compared to the wild type. It is generally assumed that a transient deposition of Mg2+ into the vacuoles would occur prior to the xylem loading for whole plant distribution as soon as Mg2+ is acquired by root cells. When external Mg2+ is sufficient, excess Mg2+ in root cells may directly move from cell to cell through symplastic transport and eventually be exported to the xylem. In addition, Mg2+ temporarily stored in the vacuole would be mobilized by tonoplast Mg2+ efflux transporters (e.g. MGT1 and MGT2) and join the symplastic flow. When external Mg2+ is deficient, much of the symplastic flow is supported by Mg2+ from vacuolar remobilization through MGT1 and MGT2 in the wild-type plants, explaining why the mgt1 mgt2 mutant retained more Mg2+ in the roots, especially under the low-Mg conditions.
Mg2+ redistribution from the old to young tissues represents another long-distance transport process that may entail the function of vacuolar Mg2+ efflux transporters. During nutrient remobilization, excess minerals stored in leaf mesophyll cells are retrieved from the vacuolar store and subsequently excreted across the plasma membrane to operate apoplastic loading into the phloem sap (Maillard et al., 2015). Previous studies showed that Mg2+ can be remobilized from old to young tissues during the life cycle, which is beneficial to the overall growth and development of the plant (Maillard et al., 2015). In this context, it is critical for the vacuolar Mg2+ pool to be efficiently remobilized by tonoplast-localized Mg2+ transporters. Compared with the wild-type plants, we found that mgt1 mgt2 retained a significantly higher Mg concentration in old leaves and reduced Mg content in the seeds, suggesting that Mg2+ efflux across the vacuolar membrane mediated by MGT1 and MGT2 serves as a rate-limiting step in Mg2+ remobilization from old leaves to young tissues such as developing seeds.
Plants fine-tune nutrient uptake and utilization by coordinating different ion transport systems to maximize their growth in response to environmental changes. Genetic analyses in this study have provided support for this notion in the context of Mg2+ homeostasis. Additive effects on plant phenotypes under low-Mg conditions were observed in the mgt1 mgt2 mgt6 triple mutant, suggesting that plant response to Mg2+ deficiency relies on both Mg2+ uptakes across the plasma membrane (MGT6) and Mg2+ remobilization (MGT1 and MGT2) from the vacuolar stores. These two transport processes also coordinate housekeeping maintenance of Mg2+ homeostasis, as evidenced by the finding that the triple mutant showed notable growth defects even when external Mg2+ is sufficient or in excess. The fact that mgt1 mgt2 mgt6 triple mutant was still viable under extremely low-Mg conditions (0.01 mM) may suggest the involvement of other types of unidentified transporters that function under Mg2+ deficiency. Alternatively, other compensation mechanisms may exist to bypass Mg2+ transport processes. For instance, degradation of Mg2+-containing compounds such as chlorophyll would provide a source of free Mg2+ ions for plants to cope with Mg2+ starvation (Peng et al., 2019).
In response to fluctuating Mg2+ levels in the environment, multiple transporters and regulatory processes may be responsible for plant adaptation. These include another important family of Mg2+ transporters termed as MGRs (for “Magnesium Release”) that have been recently identified in plants (Tang et al., 2022). They serve as active Mg2+ transporters exemplified by bacterial CorB protein (Chen et al., 2021). Although these MGRs, like MGTs, are also localized in multiple membranes including tonoplast (Tang et al., 2022), plasma membrane (Meng et al., 2022), and chloroplast envelope, they appear to mediate Mg2+ transport in the opposite direction in vivo as opposed to MGTs. MGTs mainly facilitate Mg2+ uptake into the cytoplasm while MGRs primarily function in Mg2+ exclusion away from the cytoplasm. Most relevant to this study is the tonoplast MGR1 responsible for Mg2+ sequestration into the vacuole (Tang et al., 2022). Therefore, MGR1 delivers Mg2+ into the vacuole (away from cytoplasm) while MGT1 and MGT2 mobilize the vacuolar store to retrieve Mg2+ into the cytoplasm, which may likely serve as a critical mechanism to coordinate vacuole-cytoplasm Mg2+ exchanges depending on the Mg status in the environment. Future studies will be directed to examine functional interactions among different members of these two families of Mg2+ transporters (and possibly other types), which modulate plant Mg2+ homeostasis at subcellular, cellular, and whole-plant levels. The conclusions drawn from our studies on plant Mg2+ transport may also be generally applicable to the understanding of other nutrient transport systems as well, which entails synergistic or antagonistic effects among different transport components to achieve optimal nutrient status for cell growth.
Materials and methods
Plant materials and growth conditions
Arabidopsis (A. thaliana) ecotype Col-0 was used in this study. T-DNA insertional mutant lines were obtained from the Arabidopsis Biological Resource Center. The seed stock IDs are as follows: SALK_100361 (mgt1) and SALK_006797 (mgt2). The double mutant mgt1 mgt2 was generated by crossing mgt1 with mgt2, and progeny of F2 generation was screened for double homozygous mutations in MGT1 and MGT2 using a PCR-based genotyping approach. The cbl2 cbl3 mutant was described in a previous study (Tang et al., 2012) and was crossed to mgt1 mgt2 to generate the quadruple mutant mgt1/2/cbl2/3. The triple mutant mgt1 mgt2 mgt6 was produced through a genetic cross between mgt1 mgt2 and the mgt6 single mutant (Yan et al., 2018).
Wild-type and mutant plants were grown in the soil at 22°C under a long-day (16-h-light/8-h-dark) or a short-day (8-h-light/16-h-dark) condition in the greenhouse, depending on the purpose of the experiment. Soils used in this study were Sunshine Mix #4 Professional Growing Mix (Sun Gro Horticulture; Agawam, MA, USA) that contained about 50–100 mg/kg Mg. Hydroponically grown plants were generally kept in solutions containing one-sixth strength MS salts under the short-day (8-h-light/16-h-dark) condition. Fresh liquid solutions were replaced twice a week.
Phenotypic assays
For all the phenotypic analyses on the plate, a post-germination assay was applied. Arabidopsis seeds of different genotypes were sterilized with 10% (v/v) bleach for 5 min and washed in sterilized water 3 times. Seeds were initially sown on the agar-solidified MS plates. After germination, 4-d old seedlings were transferred onto one-sixth strength Mg2+-free MS medium (containing 1% sucrose, pH = 5.8, solidified with 0.8% agarose, MgSO4 was replaced by K2SO4 to provide sulfate source) supplemented with various concentrations of Mg2+, in which MgCl2 were added as the Mg2+ source (Yan et al., 2018). Seedling phenotypes and growth parameters were recorded after the transfer as indicated in the legend of each figure.
For the Mg-starvation assay in the hydroponics, seedlings were first grown in the Mg-replete (0.25 mM Mg2+) solution containing one-sixth strength MS salts for 14 d and then transferred to a fresh hydroponic solution containing only 0.01 mM Mg2+ for starvation for 14 d. Hydroponic plants were kept at 22°C under a short-day (8-h-light/16-h-dark) condition in the greenhouse.
Functional complementation of mgt1 mgt2 mutant
For complementation of the mgt1 mgt2 mutant, a genomic fragment containing MGT1 or MGT2 coding region was amplified by PCR from Arabidopsis genomic DNA using primers listed in Supplemental Table S1. The PCR products were cloned into the Sma I site of the binary vector pCAMBIA1300. After sequencing, the constructs were transformed into Agrobacterium tumefaciens strain GV3101 and introduced into mgt1 mgt2 mutant plants by the floral dip method (Clough and Bent, 1998). Transgenic seeds were screened on MS medium supplemented with 25 mg/mL hygromycin. Resistant seedlings were transplanted into soil and grown in the greenhouse for seed propagation. T3 homozygous transgenic plants were subject to gene expression analysis and phenotypic assays together with wild-type plants and mgt1 mgt2 mutants.
Promoter-GUS fusion analysis and histochemical assay of GUS activity
For the construction of MGT1 or MGT2 promoter-driven GUS transgenic lines, an approximate 2.0 kb promoter region directly upstream of ATG start codon of MGT1 gene or MGT2, respectively, was amplified from Col-0 genomic DNA by PCR using the primers listed in Supplemental Table S1. PCR fragments were cloned into the binary vector pCAMBIA-1381Z and the constructs were introduced into the A. tumefaciens GV3101 strain, which was thereafter used to transform A. thaliana by the floral dipping method (Clough and Bent, 1998). At least 10 independent transgenic lines were selected and seedlings of T2 generation were subjected to GUS assays. For histochemical analysis to detect GUS expression, plant materials were incubated at 37°C for 4 h in the staining buffer containing 100 mM sodium phosphate, pH 7.0, 10 mM EDTA, 0.5 mM K3[Fe(CN)6], 0.5 mM K4[Fe(CN)6], 0.1% Triton X-100 and supplemented with 0.5 mM 5-bromo-4-chloro-3-indolyl-β-D-glucuronide (X-Gluc). To clear chlorophyll from the green tissues, the stained materials were incubated in 75% ethanol overnight and then kept in 95% ethanol.
RNA isolation and gene expression analysis
Arabidopsis seedlings grown on the plates were harvested and ground to fine powder in liquid nitrogen. Total RNA was extracted using the Trizol reagent (Invitrogen; Carlsbad, CA, USA). After being digested by DNase I (Invitrogen; Carlsbad, CA, USA) to decontaminate DNA, cDNAs were produced from RNA samples at 42°C using SuperScript II reverse transcriptase (Invitrogen; Carlsbad, CA, USA). The resultant cDNA samples were used for PCR amplification or RT-qPCR analysis with the gene-specific primers listed in Supplemental Table S1. RT-qPCR was performed on the DNA Engine Opticon System (MJ Research; Hercule, CA) using the SYBR Green Realtime PCR Master Mix (Bio-Rad; Hercule, CA, USA) to monitor double-stranded DNA products. Data were calculated based on the comparative threshold cycle method.
Subcellular localization of MGT1 and MGT2
The coding sequence of MGT1, MGT2, or MGT6 gene was in-frame fused with the VENUS fluorescence protein sequence under the control of Arabidopsis ubiquitin10 gene promoter. The plant expression constructs were generated on the backbone of pCambia-1300 and transformed into the A. tumefaciens GV3101 strain. For plant transient expression, Agrobacterium strains carrying different constructs were infiltrated into the leaves of N. benthamiana in the presence of p19, a viral gene silencing suppressor. Three days after infiltration, protoplasts were isolated with the enzyme solution containing 0.4 M mannitol, 20 mM MES-K (pH 5.7), 10 mM CaCl2, 5 mM β-mercaptoethanol, 0.1% BSA, 1% cellulase R10 (Yakult Honsha; Tokyo, Japan), and 0.3% macerozyme R10 (Yakult Honsha; Tokyo, Japan). Plant vacuoles from the protoplasts were further released by an osmotic shock using 200 mM sorbitol. Fluorescence in the protoplasts was imaged with a confocal laser scanning microscope (LSM710, Carl Zeiss; Oberkochen, Germany). For VENUS fluorescence proteins, the filters were set at 514 nm for excitation and 535–600 nm for emission; for chlorophyll, filters were set at 488 nm for excitation and 650–720 nm for emission.
Yeast complementation
Two yeast (S. cerevisiae) strains, mrs2Δ (MATa, leu2-0, met15-0, ura3-0, his3-1, mrs2Δ::KanMX4) and mnr2Δ (MATα, ade2, can1-100, his3-11 15, leu2-3 112, trp1-1, ura3-52, mnr2Δ::SpHIS5), were used as background strains for functional complementation assays. To express Arabidopsis MGT transporters in mrs2Δ, an upstream fragment of the yeast MRS2 gene encoding the first 103 amino acids for mitochondrial targeting and the native promoter region was amplified by PCR from the yeast genome. The amplified fragment was in-frame fused to the plant MGT genes at the N-terminus and cloned into the p416GPD vector backbone (Mumberg et al., 1995) in which GPD promoter was exercised. The resulting constructs were delivered into mrs2Δ cells using the PEG/LiAc method and the transformants were selected on SD-Ura medium. Several independent transformant clones were picked and cultured in liquid YPD medium until OD600 reached 1.0. Three microliters of serial decimal dilutions were spotted onto agar-solidified YPD medium or nonfermentable YPG medium with glycerol as the main carbon source. For functional complementation test in mnr2Δ, the yeast vector p416GPD (Mumberg et al., 1995) was employed to express various plant MGT transporters, followed by yeast transformation using the PEG/LiAc method. Tolerance tests to high-Mn or high-Ca conditions were performed with different genotypes grown on the YPD medium supplemented with 1.5 mM MnCl2 or 200 mM CaCl2. All the yeast cultures on the plate were incubated at 28°C and growth phenotypes of yeast cells were recorded after 3 d.
Measurement of ion concentration in different plant tissues
Plant samples were harvested from different plant tissues, and briefly washed with double distilled H2O for 10 s. The samples were then thoroughly dried up for 2 d in the oven at 80°C. The dry matters were collected in the 15 mL centrifuge tubes and digested with 1 mL ultrapure HNO3 (Sigma-Aldrich; Darmstadt, Germany) in the water bath at 95°C for 4 h. Digested samples were diluted to the appropriate concentrations with double-distilled H2O, and the concentration of each ion was determined by inductively coupled plasma optical emission spectroscopy (ICP-OES; PerkinElmer, MA, USA). The analytical standard from the manufacturer (Fisher Scientific; MA, USA) was diluted with 2% HNO3, similar to the solution used to prepare plant samples. Major cations in plant samples (Mg, Ca, K, and Mn) were analyzed in comparison with the standard solutions using the following wavelengths settings on ICP-OES: Mg, 280.271 nm; Ca, 393.366 nm; K, 776.409 nm; Mn, 257.61 nm.
Accession numbers
All the Arabidopsis genes involved in this study can be found in The Arabidopsis Information Resource (TAIR; www.arabidopsis.org): MGT1 (AT1G80900), MGT2 (AT1G16010), MGT3(AT2G03620), MGT4 (AT3G19640), MGT6 (AT3G58970), MGT7 (AT5G09690), CBL2 (AT5G55990), CBL3 (AT4G26570), ACA13 (AT3G22910), ARD3 (AT2G26400), CAX3 (AT3G51860), CML37 (AT5G42380), CYP81D8 (AT4G37370), GPT2 (AT1G61800), NDB2 (AT4G05020), WRKY46 (AT2G46400), SEN1(AT4G35770), and ACTIN2 (AT3G18780). Yeast gene information is available at The Saccharomyces Genome Database (SGD; www.yeastgenome.org): MNR2 (YKL064W) and MRS2 (YOR334W).
Supplemental data
The following materials are available in the online version of this article.
Supplemental Figure S1. Expression of MGT1 and MGT2 in response to different external Mg2+ concentrations.
Supplemental Figure S2. Functional analysis of MGT transporters in the yeast mrs2Δ mutant strain.
Supplemental Figure S3. Identification of T-DNA insertional mutants of mgt1 and mgt2 in Arabidopsis.
Supplemental Figure S4. Phenotype of mgt1 mgt2 mutant under different ionic stress conditions.
Supplemental Figure S5. Expression of MGT1 or MGT2 in the mgt1 mgt2 double mutant rescues the growth defect under low-Mg conditions.
Supplemental Figure S6. Ca concentration in Col-0 and mgt1 mgt2 plants under various growth conditions.
Supplemental Figure S7. Ca, K, and Mn concentrations in different tissues of Col-0 and mgt1 mgt2 adult plants.
Supplemental Table S1. Primers used in this study.
Supplemental Data Set S1. Raw experimental data collection from this study.
R.-J.T. and S.L. conceived the study and designed the experiments; R.-J.T. performed all the experiments with the assistance by Y.Y., Y.-W.Y., D.-D.M., H.-M.Y., and F.-G.Z.; C.W. helped with the data analysis and preparation of some of the figures; R.-J.T. and S.L. wrote the manuscript.
The author responsible for distribution of materials integral to the findings presented in this article in accordance with the policy described in the Instructions for Author (https://dbpia.nl.go.kr/pphys/pages/general-instructions) is: Sheng Luan ([email protected]).
Acknowledgements
We thank Dr. Colin W. MacDiarmid for DY1514 and mnr2Δ yeast strains.
Funding
This work was supported by the National Science Foundation (Award # 2041585 to S.L.). Y.-W.Y, D.-D.M., and H.-M.Y. were supported, in part, by fellowships from the China Scholarship Council.
Conflict of interest statement. We declare no conflicts of interest.
References
Author notes
Senior author