-
PDF
- Split View
-
Views
-
Cite
Cite
Min Xiong, Jiawen Yu, Jindong Wang, Qiang Gao, Lichun Huang, Chen Chen, Changquan Zhang, Xiaolei Fan, Dongsheng Zhao, Qiao-Quan Liu, Qian-Feng Li, Brassinosteroids regulate rice seed germination through the BZR1-RAmy3D transcriptional module, Plant Physiology, Volume 189, Issue 1, May 2022, Pages 402–418, https://doi.org/10.1093/plphys/kiac043
- Share Icon Share
Abstract
Seed dormancy and germination, two physiological processes unique to seed-bearing plants, are critical for plant growth and crop production. The phytohormone brassinosteroid (BR) regulates many aspects of plant growth and development, including seed germination. The molecular mechanisms underlying BR control of rice (Oryza sativa) seed germination are mostly unknown. We investigated the molecular regulatory cascade of BR in promoting rice seed germination and post-germination growth. Physiological assays indicated that blocking BR signaling, including introducing defects into the BR-insensitive 1 (BRI1) receptor or overexpressing the glycogen synthase kinase 2 (GSK2) kinase delayed seed germination and suppressed embryo growth. Our results also indicated that brassinazole-resistant 1 (BZR1) is the key downstream transcription factor that mediates BR regulation of seed germination by binding to the alpha-Amylase 3D (RAmy3D) promoter, which affects α-amylase expression and activity and the degradation of starch in the endosperm. The BZR1-RAmy3D module functions independently from the established Gibberellin MYB-alpha-amylase 1A (RAmy1A) module of the gibberellin (GA) pathway. We demonstrate that the BZR1-RAmy3D module also functions in embryo-related tissues. Moreover, RNA-sequencing (RNA-seq) analysis identified more potential BZR1-responsive genes, including those involved in starch and sucrose metabolism. Our study successfully identified the role of the BZR1-RAmy3D transcriptional module in regulating rice seed germination.
Introduction
Seed dormancy and germination, two distinct physiological processes that are characteristic of seed-bearing plants, are critical for both plant development and crop production. Successful seed germination is an important beginning for the next round of the plant life cycle, and thus leads to robust and healthy seedling development and thus high yielding crops. Seed germination begins with the absorption of water and ends with the protrusion of the radicle. Suitable temperatures and sufficient availability of water and oxygen are the prerequisites for normal germination (Holdsworth et al., 2008). Once the seeds begin to germinate after water absorption (imbibition), seed storage reserves, including starch and storage proteins, are degraded by various hydrolytic enzymes and then converted into energy and nutrients for radicle protrusion and subsequent seedling growth. In rice (Oryza sativa) seed germination, the carbon source needed for the early heterotrophic embryo (the sink) is completely dependent on the carbohydrate reserve in the endosperm (the source; Scofield et al., 2007). Starch, as the primary source of carbohydrate during germination, plays an important role in the source–sink communication. In cereal endosperms, the degradation of starch into glucose requires a number of enzymes, including α-amylase, β-amylase, limit dextrinase (LDA), and maltase (Zeeman et al., 2010). Briefly, α-amylase attacks starch granules to release branched and linear glucose polymers, which are then transformed into the linear glucans by LDA. Subsequently, α-amylase and β-amylase further catalyze the degradation of linear glucans into glucose, which is then used for embryo growth (Noronha et al., 2018). In barley (Hordeum vulgare), during the gradual degradation of seed starch, size of the surface pores of the starch granule are increased, and internal erosion and disintegration of the granules are intensified (Andriotis et al., 2016).
As a pivotal enzyme that catalyzes the degradation of starch in cereal seeds, α-amylase has been reported to be closely related to multiple key agronomic traits, such as germination rate (Asatsuma et al., 2005), seedling vigor (Karrer et al., 1992), and hypoxia stress (Hwang et al., 1999). In cereals, the α-amylase family contains a number of members. For example, there are 10 members in rice (Damaris et al., 2019), 12 in barley (Zhang and Li, 2017), and 22 in wheat (Triticum aestivum; Huang et al., 1992). Based on the sequence polymorphism of α-amylase genes, they can be divided into two major groups in rice, AmyA and AmyB. The AmyA group is further subdivided into the Amy1 and Amy2 subfamilies, and the AmyB group includes the Amy3 subfamily (Huang et al., 1992). The members of these subfamilies are designated alpha-amylase 1A (RAmy1A), RAmy1B, RAmy1C, RAmy2A, RAmy3A, RAmy3B, RAmy3C, RAmy3D, RAmy3E, and RAmy3F. Except for RAmy3F, most α-amylase genes have been experimentally verified. Regulating the expression of α-amylase genes is a common strategy by which plants coordinate growth and development. Multiple conserved cis-elements in the α-amylase gene promoters are crucial for this regulation. For example, gibberellin (GA) activates α-amylase expression through the GA response complex (GARC) motif (Lanahan et al., 1992). GARC includes two closely correlated components, namely the GA response element (GARE) and TA box (Chen et al., 2006). Gibberellin MYB (GAMYB), a GA-induced key transcription factor, binds directly to GARE and positively regulates the expression of a series of hydrolase genes, including amylase genes (Gubler and Jacobsen, 1992; Washio, 2003). In barley, abscisic acid (ABA) antagonizes GA by inhibiting GAMYB expression and subsequent α-amylase expression, thus modulating seed dormancy and germination (Gómez et al., 2001). Under conditions of sugar starvation, a transcription factor called MYBS1 is induced and interacts with the TA box in the RAmy3D promoter, thus promoting seed germination (Lu et al., 2007). Moreover, GA promotes the co-nuclear transport of GAMYB and MYBS1 to form the (MYBS1-GAMYB)-DNA (GARE-TA box) complex, which induces amylase gene expression (Hong et al., 2012). Therefore, the two MYB transcription factors play key roles in coordinating the expression of α-amylase by sugars and GA. There are also other abiotic factors that directly or indirectly regulate seed germination, such as salinity (Liu et al., 2018). In addition, chemicals, such as maleic hydrazide, eugenol, and uniconazole reduce the expression of RAmy3B and RAmy3E in hybrid rice seeds, thus preventing pre-harvest sprouting (PHS; Hu et al., 2016). PHS is one of the most severe problems affecting rice production that causes reductions in rice yield, grain quality, and seed vitality. Therefore, α-amylase genes are crucial determinants of both seed germination and PHS.
Although considerable progress has been made in understanding how GA and ABA regulate seed germination and dormancy, the mechanisms by which the other phytohormones modulate these processes are still mostly obscure. For example, brassinosteroids (BRs), a major class of growth promoting phytohormones, are also involved in regulating seed germination (Tong and Chu, 2018; Li et al., 2020). In Arabidopsis (Arabidopsis thaliana), BR promotes seed germination via antagonizing the suppression effect of ABA, mechanistically by targeting ABSCISIC ACID-INSENSITIVE5 (ABI5), a key component in ABA signaling pathway (Hu and Yu, 2014; Zhao et al., 2019). Moreover, BR and GA co-induce seed germination via activating GASA6 in Arabidopsis (Zhong et al., 2021). Further dissecting the mechanism of action of BRs in rice germination will not only advance our understanding of BR signaling in crops, but also boost its future application in rice breeding programs. Considering that BRs play multifaceted roles in several key agronomic traits of rice, optimizing the manipulation of these hormones for desired beneficial traits without adverse effects is vital to their practical application in agriculture. In general, rice shares a similar primary BR signaling pathway with Arabidopsis. In brief, the BR signal is sensed by the BR insensitive 1 (OsBRI1) receptor and the BRI1-associated receptor kinase 1 (OsBAK1) coreceptor which triggers a series of downstream phosphorylation events, including the activation of BR-signaling kinase 3 (OsBSK3) kinase and the suppression glycogen synthase kinase 2 (GSK2) kinase activity (Tong and Chu, 2018). OsGSK2, a GSK3/SHAGGY-like kinase homologous to BR-insensitive 2 (BIN2) in Arabidopsis, acts as the central negative regulator of the BR signaling pathway by phosphorylating and inactivating the downstream transcription factors, including brassinazole-resistant 1 (OsBZR1) and dwarf and low-tillering (DLT; Bai et al., 2007; Tong et al., 2012). At present, it is still unclear whether starch, the most abundant seed storage reserve, is also involved in BR-regulated rice germination. The underlying molecular mechanism remains to be discovered. In this study, we describe a novel regulatory module of seed germination, from the BR receptor to the downstream key transcription factor BZR1 and its specific target genes, and we highlight the central roles of the new BZR1-RAmy3D module in starch mobilization.
Results
BR signaling promotes seed germination and post-germination growth
To determine whether BR-signaling affects seed germination in rice, a number of BR-related rice and mutant lines were used in a germination assay, including d61 and its wild-type Taichung 65 (TC65), Go, dlt, Do, and their wild-type Zhonghua 11 (ZH11). Since various BR mutants were produced in different recipient rice varieties, specific wild-type should be used as the control for corresponding BR mutants in various experiments. d61 is a mild BR-insensitive mutant with a defect in the BR receptor BRI1 (Yamamuro et al., 2000), whose plant height, grain size, and 1,000-grain weight were all significantly declined (Supplemental Figure S1, A–F). Go is a transgenic rice line that overexpresses GSK2 (Tong et al., 2012). Similarly, its plant height, grain size, and 1,000-grain weight were also all decreased (Supplemental Figure S1, G–L). In addition, dlt and Do are the DLT mutant and overexpression lines, respectively (Tong et al., 2012). Interestingly, plant height in both the Do overexpression lines and dlt mutant was decreased compared to the wild-type (Supplemental Figure S1M). Moreover, the grain length of rice Do was increased, while its grain width was decreased; the change of grain length and width of dlt mutant was opposite to that of Do (Supplemental Figure S1, N–R). The result of germination assay showed that the BR receptor mutant d61 was ∼12 h slower than the wild-type control to reach 100% germination (Figure 1A). Moreover, the shoot lengths of the germinating d61 seeds were shorter than those of the wild-type (Figure 1B; Supplemental Figure S2). As expected, the germination rate and shoot length of seeds from plants overexpressing OsGSK2 (Go) were similar to those of the d61 mutant (Figure 1, C and D). However, both the overexpression line (Do) and the mutant (dlt) of DLT, a GSK2-regulated transcription factor that is important in the BR signaling pathway, showed no observable differences in seed germination compared with the wild-type (Figure 1, E and F). Therefore, BR positively regulates seed germination and embryo growth, but the regulation is not mediated by the transcription factor DLT.
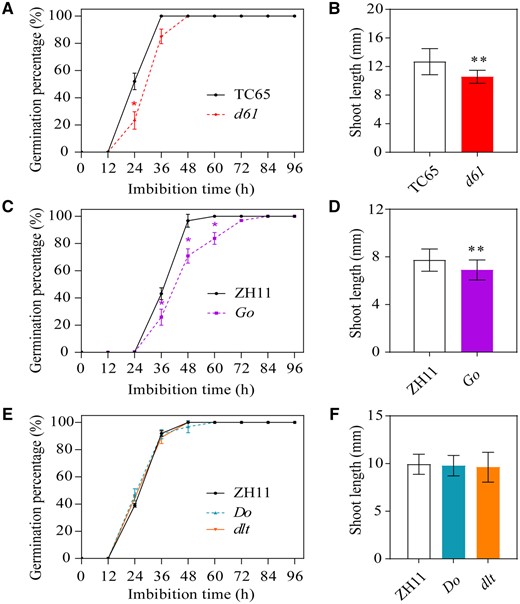
Germination analysis of BR-insensitive mutant seeds. A–F, Germination rate from 0 to 96 HAI and shoot length at 96 HAI in d61 and wild-type TC65 seeds (A and B), Go and wild-type ZH11 seeds (C and D), and Do, dlt, and ZH11 seeds (E and F). Error bars represent sd (n = 3 experiments, each replicate contained 30 seeds). *P < 0.05; **P < 0.01 (Student’s t test).
The bzr1 mutant shares similar germination features with d61
In addition to DLT, OsBZR1 is another key transcription factor in the BR signaling pathway. Therefore, we further tested whether it is OsBZR1 that connects the upstream BR signal with the downstream specific target genes that control seed germination. First, we generated OsBZR1 overexpression (BZR1-OX) and knockout (bzr1) lines under the genetic background of the japonica cultivar Nipponbare (Nip). Gene expression analysis indicated that OsBZR1 expression was notably increased in seeds of the overexpression lines (Figure 2A). Western blotting further confirmed that the BZR1-3XFLAG fusion protein was successfully expressed in the test lines (Figure 2B). Further pharmacological analyses showed that the BZR1 protein abundance slightly decreased in response to brassinazole (BRZ) treatment, a BR biosynthesis inhibitor (Figure 2C). While brassinolide (BL) treatment notably induced the dephosphorylation of BZR1, thus activating the BR signaling pathway (Figure 2D). As for the bzr1 mutants generated via clustered regularly interspaced short palindromic repeats/CRISPR associated protein 9 (CRISPR/Cas9) genome editing, DNA sequencing showed that two homozygous mutants were obtained, bzr1-i1 and bzr1-d4, which resulted from a one basepair (bp) insertion and a 4-bp deletion in the first exon, respectively (Figure 2E).
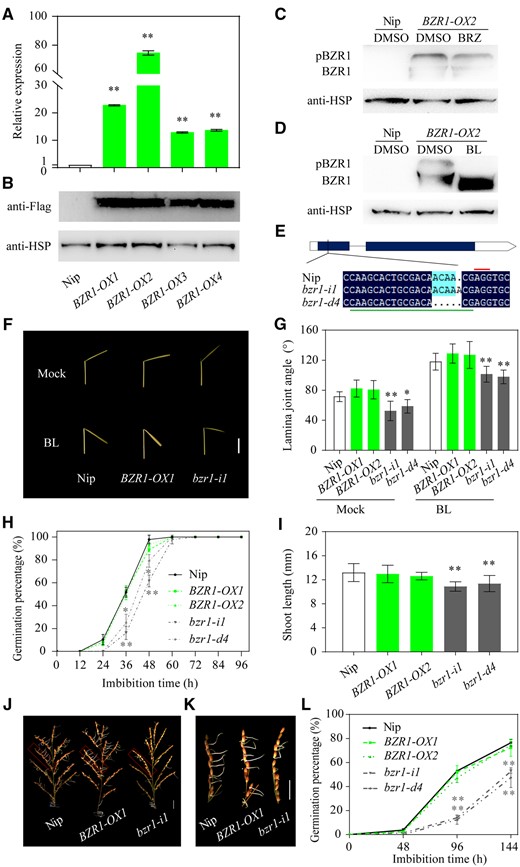
Germination of transgenic BZR1-OX and bzr1 mutant rice seeds. A, Expression of BZR1 in the seeds of four OsBZR1-OX lines and “Nip”. Actin1 was used as the internal control for normalization of gene expression data. B, Expression analysis of BZR1-3XFlag fusion protein in the leaves of OsBZR1-OX rice seedlings. C, Effect of BRZ (2 µM) treatment on the abundance of BZR1-3XFlag. The 5-d-old seedlings were grown in water supplemented with 2-µM BRZ or DMSO mock for another 5 d. BRZ, a specific BR biosynthetic inhibitor. D, Effect of BL (1 µM) treatment on the phosphorylation status of BZR1-3XFlag. The leaves of 10-d-old rice seedlings were treated with 1-µM BL or DMSO for 3 h in vitro. BL, the most active BR. Detection of heat shock protein (HSP) was used as the reference for protein loading. BZR1-3XFlag and HSP proteins were detected with antibodies directed against the 3XFlag tag epitope and HSP, respectively. E, Schematic diagram of the target sequence for OsBZR1 gene editing and the sequences of the two bzr1 mutants generated using CRISPR/Cas9. bzr1-i1 and bzr1-d4 have a 1-bp insertion and a 4-bp deletion, respectively, in the OsBZR1 target site (underlined in green). The 5′-AGG-3′ protospacer-adjacent motif is marked with a red line. F, BL sensitivity test of BZR1-OX1, bzr1-i1, and wild-type seedlings using the lamina joint inclination assay. Scale bar = 1 cm. G, Quantitative analysis of the lamina joint inclination assay data as described in (F). Error bars represent sd (n = 3 experiments, each replicate contained 20 seedlings). H and I, Germination rate (H) and shoot length at 96 HAI (I) of germinated BZR1-OX1, bzr1-i1, and wild-type Nip seeds. J and K, PHS phenotypes of mature rice panicles (J) and spikelets (K) of BZR1-OX1, bzr1-i1, and the wild-type after 6 d of imbibition in water. Scale bar = 2 cm. L, Time-course analysis of seed germination rates of the panicles from wild-type, two BZR1-OX lines, and two bzr1 mutants. Error bars represent sd (n = 3 experiments, each replicate contained 30 seeds or 10 panicles). In this figure, *P < 0.05; **P < 0.01 (Student’s t test). The images were digitally extracted for comparison in panels F, J, and K.
We next examined several important agronomic traits of the OsBZR1-related rice lines, including plant height, grain size, and 1,000-grain weight. Plant height in both the OsBZR1 overexpression and knockout lines was slightly decreased compared to the control (Supplemental Figure S3, A and B), which is similar to that in DLT mutant and overexpression rice (Supplemental Figure S1M; Tong et al., 2009, 2012). We hypothesize that the reduction of plant height in OsBZR1 and DLT overexpression lines should be due to the dose-dependent effect of BR on cell elongation, that is low concentrations of BR increase while high concentrations of BR often reduce plant height (Tong et al., 2014). As to grain size, no change was observed in the OsBZR1-OX line, but the length, width, and thickness of bzr1 mutant grains were all slightly reduced (Supplemental Figure S3, C–F). Hence, the 1,000-grain weight of the bzr1 mutants were reduced by 7%–8% compared to the wild-type, while the BZR1-OX grains were not significantly different (Supplemental Figure S3, C–G). Moreover, bzr1 seedling height was also reduced compared to wild-type, while that of BZR1-OX seedlings was not (Supplemental Figure S4). Next, the lamina joint inclination test was performed to examine the leaf angles of BZR1-related materials, which is the most distinctive BR-responsive architectural trait in cereals. Ample evidence demonstrates that the leaf angles of BR-deficient or -insensitive mutants are reduced while that of BR-treated rice is increased (Sakamoto et al., 2006; Tong et al., 2012; Sun et al., 2015). The test result showed that although the leaf angle in BZR1-OX seedlings was unchanged, the leaf angles of the bzr1 mutants was smaller than in the wild-type, both with or without BR treatment (Figure 2, F and G), indicating that knockout of OsBZR1 expression reduces seedling leaf angle and BR sensitivity. Since rice plants with erect leaves (small leaf angle) can improve grain yield per unit area in dense planting populations (Sinclair and Sheehy, 1999), bzr1 mutants may have the potential to enhance rice grain yield.
In addition, germination of the bzr1 mutant seeds was delayed, while that of BZR1-OX seeds was essentially unchanged compared to the control (Figure 2H). In more detail, the dynamic germination pattern of the bzr1 mutants was similar to that of the d61 mutant and the OsGSK2 overexpression line; both took at least 12-h longer than the wild-type to reach 100% germination (Figure 1, A and C). Moreover, shoot length in the bzr1 mutants was also significantly reduced (Figure 2I; Supplemental Figure S5). Most importantly, PHS in the bzr1 mutants was less severe than in the wild-type under conditions of high temperature and humidity (Figure 2, J and K). The germination rates of the bzr1 mutants were notably lower than in the wild-type at each time-point examined (Figure 2L), and the shoot lengths were also shorter in the bzr1 mutants (Figure 2K). Therefore, although mutations in OsBZR1 cause a 12-h delay in seed germination and a slight reduction in shoot length, their effect on enhancing PHS tolerance in rice was substantial.
BR signaling enhances starch mobilization in germinated seeds
Since α-amylase and starch mobilization play important roles in regulating seed germination, we investigated whether BZR1 also regulates seed germination in this way. Thus, α-amylase activity in the BR-related rice lines was determined qualitatively and quantitatively. First, starch plate test was performed for evaluation of the α-amylase activity. In more detail, the embryo-less half-seeds can synthesize and secrete α-amylases for degradation of starch, thus producing the colorless halo around the seed. Therefore, the halo size positively corresponds to α-amylase activity of seeds. The results of starch plate test showed that the clear zones around d61, Go, and bzr1 seeds were smaller than the zones around seeds from their respective wild-types (Figure 3, A–F; Supplemental Figure S6, A and B), suggesting that less α-amylase was produced in these seeds during germination. Next, we directly measured the α-amylase activity of the germinating seeds, and the results were consistent with those of the starch plate test (Figure 3, G–I; Supplemental Figure S6C), implying that reduced α-amylase activity could be one important reason for the delayed germination in seeds of BR-insensitive rice.

Analysis of α-amylase activity in BR signaling-related rice lines. A–C, Representative images of qualitative comparisons of α-amylase activity between BR signaling-related rice lines and their corresponding wild-types at 96 HAI in the starch plate test. Scale bar = 10 mm. D–F, Quantitative analysis of the halo diameters in the starch plate test. G–I, Quantitative analysis of α-amylase enzyme activity data in the rice samples at 96 HAI. Error bars represent sd (n = 3 experiments, each replicate contained 30 seeds). *P < 0.05; **P < 0.01 (Student’s t test).
To further confirm our hypothesis that BR regulates α-amylase activity and that starch degradation affects seed germination, we measured the contents of total starch and soluble sugar of the different BR-insensitive mutants. The results showed that blocking BR signaling indeed reduces the degradation of total starch (Supplemental Figure S7, A–C) and results in less soluble sugar contents in the germinating seeds at 96 h after imbibition (HAI; Supplemental Figure S8, A–C), which is consistent with above α-amylase activity test. Although the soluble sugar content of Go seeds was higher than in the wild-type before germination, after 96 h of imbibition, the soluble sugar content of the Go seeds was lower (Supplemental Figure S8B). These findings suggest that BRs likely fine-tune seed germination by regulating the conversion efficiency of stored starch to soluble sugar.
OsBZR1 directly regulates RAmy3D expression
To further determine which α-amylase family member directly connects the BR pathway and endosperm starch degradation, we examined the expression of rice α-amylase genes. First, we evaluated BZR1 expression at different time points during seed germination. The results showed that BZR1 transcription is highest at the initial stage of seed imbibition (2 HAI) followed by a sharp decline at 24 HAI, after which it gradually increased and reached a second peak at 72 HAI (Supplemental Figure S9). Considering the essential role of α-amylase in the first step of starch degradation and that BZR1 expression was the highest at 2 HAI, we examined the transcription of all reported α-amylase genes in the seeds at 2 HAI. In general, the expression of RAmy3D was the highest in the early stage of seed germination (Figure 4, A and B). In d61, the expression of RAmy3D was downregulated, while the expression of three other family members with moderate expression levels varied; RAmy3E expression was also downregulated while RAmy3A and RAmy1A were upregulated (Figure 4A). In addition, RAmy3D had a much larger expression change in BZR1-related rice lines than in the d61 mutant, with an increase in BZR1-OX seeds and a decrease in the bzr1-i1 mutant (Figure 4B). Furthermore, we also monitored the expression pattern of RAmy3D during seed germination. Interestingly, its expression pattern was somewhat similar to that of BZR1; that is, its highest expression occurs at the initial stage of seed germination (Supplemental Figure S10). Based on these data, we hypothesized that RAmy3D could be the potential target of BZR1 that is responsible for BR-regulated starch degradation during seed germination.
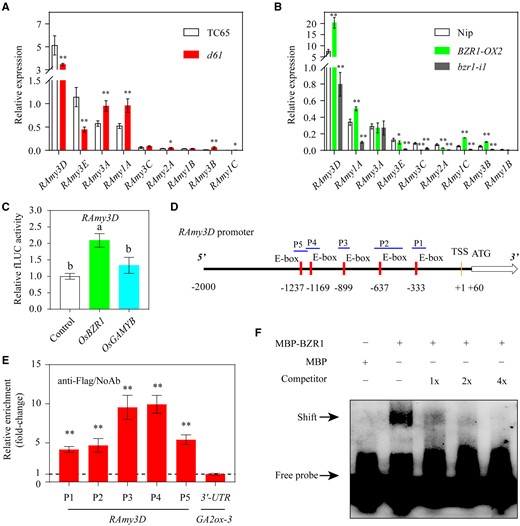
OsBZR1 binds directly to the promoter of RAmy3D and activates its expression. A, RT-qPCR analysis of nine α-amylase family genes in seeds of the d61 mutant and the wild-type TC65 at 2 HAI. B, RT-qPCR analysis of the α-amylase family genes in the seeds of BZR1-OX and the bzr1-i1 mutant and the wild-type Nip at 2 HAI. In A and B, Actin1 was used as the internal gene expression control. Error bars represent sd (n = 3 experiments). *P < 0.05; **P < 0.01 (Student’s t test). C, BZR1, but not GAMYB, activates the RAmy3D promoter. The relative activation values are shown by the LUC/REN ratios. The LUC/REN value for the empty vector plus RAmy3D promoter reporter control sample was set to 1. LUC, firefly luciferase; REN, renilla luciferase. Error bars represent sd (n = 6 experiments). Different letters indicate significant differences at P < 0.05 by Duncan’s multiple range test. D, Distribution of five BZR1-binding E-box motifs in the 2-kb region upstream of the RAmy3D transcription start site (TSS). The P1 to P5 regions marked with blue lines represent the positions used for the ChIP-qPCR assay. E, Relative enrichment of the OsBZR1 binding sites compared with the samples without antibody (NoAb) determined by the qPCR assay. All values were normalized to an unrelated Actin1 intron region, and a DNA fragment from the 3′ untranslated region (UTR) of GA2ox-3 was used as the negative control (Tong et al., 2014). Error bars represent sd (n = 3 experiments). **P < 0.01 (Student’s t test). F, OsBZR1 binding to the P4 region of the RAmy3D promoter shown in an EMSA. The MBP–BZR1 recombinant protein was mixed with the labeled P4 probe together with different amounts of the unlabeled probe as competitor. − or + represent the absence or presence of the indicated components, respectively. The first lane without MBP–BZR1 protein did not cause a mobility shift in the labeled probe.
To further confirm this notion, a dual-luciferase reporter approach was used to test how BZR1 regulates RAmy3D. The promoter of RAmy3D was fused with LUC to generate a reporter plasmid, and the 35S::BZR1 construct was used as the effector (Supplemental Figure S11). Consistent with our previous results, BZR1 overexpression significantly upregulated the expression of LUC driven by the RAmy3D promoter (Figure 4C). Considering that BZR1 is an important transcription factor that can directly bind to the BR response element (BRRE) elements and E-box in the target gene promoters (He et al., 2005), we analyzed the RAmy3D promoter for potential OsBZR1 binding sites. We identified a total of five E-box motifs in the 2-kb region upstream of the RAmy3D transcription start site (Figure 4D). Hence, specific primers were designed to confirm the direct binding of BZR1 to these motifs using the chromatin immunoprecipitation ChIP quantitative polymerase chain reaction (ChIP-qPCR) assay. This analysis indicated that all five test regions were enriched, with the highest enrichment in the P3 and P4 regions (Figure 4E). To further verify the direct binding of BZR1 to the RAmy3D promoter, we performed an electrophoretic mobility shift assay (EMSA). Here, the P3 and P4 regions were selected for analysis due to their high level of enrichment in the ChIP-qPCR assay. Unsurprisingly, the EMSA result showed that maltose binding protein (MBP)–BZR1 directly interacts with both the P3 and P4 promoter regions (Figure 4F; Supplemental Figure S12). As the amount of unlabeled competitor probes increased, the BZR1-labeled probe complexes were gradually eliminated (Figure 4F; Supplemental Figure S12). Therefore, we conclude that OsBZR1 regulates RAmy3D expression by directly binding to its gene promoter.
The BZR1-RAmy3D module is independent of the GAMYB-RAmy1A module
GA can activate the GAMYB transcription factor and enhance its binding to the GARE motif in the RAmy1A promoter, thus promoting its expression (Washio, 2003). Because it is well-known that BR interacts with GA to coordinate multiple plant growth and developmental events, it will be interesting to determine whether they also show crosstalk in modulating starch mobilization and the subsequent seed germination. Therefore, a dual luciferase reporter analysis was performed, and the results confirmed that GAMYB promoted the expression of LUC driven by the RAmy1A promoter, whereas BZR1 did not (Figure 5A; Supplemental Figure S11). In contrast, the promotion effect of GAMYB on the RAmy3D promoter was not significant (Figure 4C). These data imply that BR regulates starch degradation via the specific BZR1-RAmy3D module, while GA acts through the particular GAMYB-RAmy1A module. Interestingly, although three E-boxes and one BRRE element are present in the RAmy1A promoter (Figure 5B), the ChIP-qPCR result showed no enrichment of these regions (Figure 5C). Moreover, RAmy1A exhibited a different expression pattern to RAmy3D (Supplemental Figure S13). In addition, RAmy3D is not the target of GAMYB because it lacks the GARE motif. Correspondingly, its transcription is not regulated by GAMYB based on the result of dual luciferase reporter assay (Hong et al., 2012; Figure 4C). Furthermore, BZR1 did not directly interact with GAMYB (Figure 5D). Therefore, BZR1-RAmy3D and GAMYB-RAmy1A appear be two independent regulatory modules that control α-amylase gene expression and starch degradation.
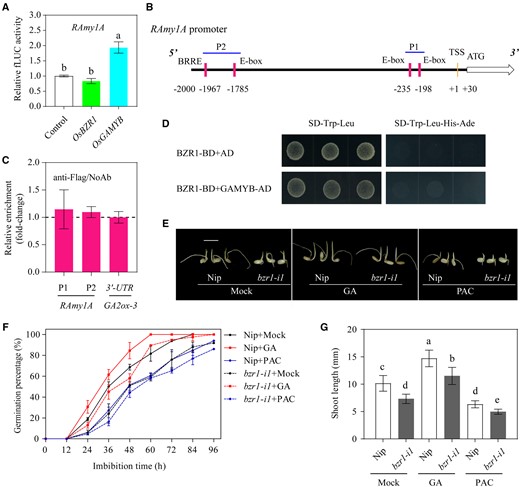
The BZR1-RAmy3D transcriptional module is independent of the established GAMYB-RAmy1A module. A, GAMYB, not BZR1, activates transcription in the RAmy1A promoter. The relative activation is shown by the LUC/REN ratio. The LUC/REN value of the empty vector plus the RAmy1A promoter reporter control sample was set to 1. LUC, firefly luciferase; REN, renilla luciferase. Error bars represent sd (n = 3 experiments). Different letters indicate significant differences at P < 0.05 by Duncan’s multiple range test. B, Distribution of three E-box motifs and one BRRE in the 2-kb region upstream of the RAmy1A transcription start site (TSS). The P1 and P2 regions marked with blue lines represent the position of the DNA fragments used for the ChIP-qPCR assay. C, Relative enrichment of the OsBZR1 binding sites compared with the samples without antibody (NoAb) determined by qPCR assay. All values were normalized to an unrelated Actin1 intron region, and a DNA fragment located in the 3′–untranslated region (UTR) of GA2ox-3 was used as the negative control (Tong et al., 2014). Error bars represent sd (n = 3 experiments). D, BZR1 does not directly interact with GAMYB in a yeast two-hybrid assay. The empty pGADT7 (AD) vector was used as the negative control. E, Morphology of germinating seeds at 96 HAI of the bzr1 mutant and the Nip wild-type treated with gibberellin (GA, 10 µM), paclobutrazol (PAC, 10 µM), or the ethanol mock treatment. PAC is a GA biosynthesis inhibitor. Scale bar = 10 mm. F and G, Germination rate (F) and shoot length at 96 HAI (G) in germinated seeds of the bzr1 mutant and Nip treated with GA, PAC, or ethanol (mock). Error bars represent sd (n = 3 experiments, each replicate contained 30 seeds). *P < 0.05; **P < 0.01 (Student’s t test). The images were digitally extracted for comparison in (E).
We also evaluated the sensitivity of bzr1-i1 to GA and paclobutrazol (PAC), a GA biosynthesis inhibitor, during seed germination (Figure 5E). The data from this experiment showed that GA treatment promoted while PAC treatment delayed seed germination of both Nip and the bzr1 mutant when compared with their respective ethanol-treated controls (Figure 5F). On the other hand, the bzr1 mutant always germinated slower than the wild-type under the same germination conditions, either with GA, PAC, or mock treatment (Figure 5F). Moreover, GA only partially restored, whereas PAC further aggravated, the suppressive effect of the bzr1-i1 mutation on shoot elongation (Figure 5, E and G). The above data suggest that BZR1-regulated seed germination is at least partially independent of GA.
BR promotes shoot elongation by increasing the degradation of transient starch in the embryo
Considering that RAmy3D promoted starch degradation is correlated with the release of energy for shoot elongation under anoxic conditions (Damaris et al., 2019), we further investigated whether the BZR1-RAmy3D module is also directly involved in rice shoot elongation. Since bending in growing shoots is often caused by the unequal growth of cells on opposite sides, we examined the faster and slower growing sides of the shoots, which we called the outside and inside parts, respectively. Interestingly, more transient starch granules were found to accumulate in the inside cells compared to the outside cells (Figure 6, A–N), implying that increased transient starch granule accumulation is negatively correlated with shoot growth. Furthermore, it was obvious that more starch granules accumulated in each side of d61 shoots compared to their counterparts in the wild-type (Figure 6, A–G). Similar phenomena were observed in the bzr1-i1 mutant and its wild-type (Figure 6, H–N). Moreover, the general cell size in both the d61 and bzr1-i1 mutants was smaller than that in their respective wild-types (Supplemental Figure S14), which is consistent with previous reports in Arabidopsis (Zhong et al., 2021).

The BZR1-RAmy3D transcriptional module also plays essential roles in tissues of the embryo. A–F, Microscopic observation of starch granules that have accumulated in the shoots of Taichung 65 (TC65) (A–C) and d61 (D–F) at 36 HAI. The samples were embedded in LR white resin, cut into semi-thin sections, and stained with iodine solution. (B) and (C) are enlargements of the rectangles marked with blue boxes on the inside parts and green boxes on the outside parts of the shoots in (A), respectively. E and F are enlargements of the rectangles marked with blue boxes on the inside parts and green boxes on the outside parts of the shoots in (D), respectively. Scale bar = 20 µm. G, Statistical analysis of the average number of starch granules per cell in shoots of the d61 mutant and TC65 control. H–M, Microscopic observation of the starch granules that have accumulated in the shoots of Nip (H–J) and the bzr1-i1 mutant (K–M) at 36 HAI. I and J are the enlargements of the rectangles marked with blue boxes on the inside parts and green boxes in the outside parts of the shoots in (H), respectively. L and M are the enlargements of the rectangles marked with blue boxes on the inside parts and green boxes on the outside parts of the shoots in (K), respectively. N, Statistical analysis of the average number of starch granules per cell in shoots of the bzr1-i1 mutant and Nip control. In (G) and (N), error bars represent sd (n = 3 experiments, each replicate contained 30 cells). Different letters indicate significant differences at P < 0.05 by Duncan’s multiple range test. O and P, Morphology (O) and quantitative analysis (P) of the plumule lengths in the d61 mutant and TC65 control cultivated in vitro for 120 h supplemented with Glc or Man or the mock control. Q and R, Morphology (Q) and quantitative analysis (R) of the plumules length in the bzr1-i1 mutant and Nip control cultivated in vitro for 120 h supplemented with Glc or Man or mock. Glc, glucose; Man, mannitol. Scale bar = 1 mm. Error bars represent sd (n = 3 experiments, each replicate contained 20 plumules). **P < 0.01 (Student’s t test). The images were digitally extracted for comparison in (O) and (Q).
To verify whether the BZR1-RAmy3D module also functions in the embryo tissues, we first performed reverse transcription quantitative polymerase chain reaction (RT-qPCR). The results showed that RAmy3D expression is notably decreased in d61 and bzr1-i1 plumules compared to their respective wild-type controls (Supplemental Figure S15). To further clarify this observation and to eliminate potential interference from the endosperm (the different glucose flow from degraded starch in various endosperm sources, for example), the in vitro embryogenic culture system was used. In this assay, isolated embryos were cultured in vitro. The results indicated that the shoot lengths of the d61 and bzr1-i1 mutants showed no significant differences from their respective wild-types when cultured with water only (Figure 6, O–R). However, in the presence of glucose, the shoot lengths of the wild-types markedly increased, while shoot lengths of the d61 and bzr1-i1 mutants showed no significant change (Figure 6, O–R). We used the same concentration of mannitol to rule out the possibility that the effect on shoot growth resulted from osmotic pressure (Figure 6, O–R). In summary, blocking BR signaling attenuated BZR1 activity and RAmy3D expression, thus triggering the slower degradation of transient starch in embryo cells and the consequent inhibition of embryo growth.
Transcriptome profile of BZR1-responsive genes during seed germination via RNA-seq
To explore other BZR1-responsive targets involved in regulating rice germination, an RNA-seq experiment was performed using the BZR1-OX2 line. A total of 1,682 differentially expressed genes (DEGs) were identified in the BZR1-OX2 line (fold change >1.5, adjusted P <0.05), including 228 upregulated and 1,634 downregulated genes (Supplemental Figure S16A). Not surprisingly, both OsBZR1 and RAmy3D were in the list of upregulated genes (Supplemental Table S1). Based on the Kyoto Encyclopedia of Genes and Genomes (KEGG) analysis, the five most enriched pathways of the DEGs included “phenylpropanoid biosynthesis”, “plant hormone signal transduction”, “plant–pathogen interaction”, “starch and sucrose metabolism”, and “fatty acid elongation” (Supplemental Figure S16B). We then randomly selected four genes from each group of DEGs to verify the RNA-seq results. RT-qPCR assays showed that the expression changes in these genes in the BZR1-OX2 line were the same as determined by the RNA-seq data, while seven out of the eight genes showed the opposite changes in expression in the bzr1 mutant compared to BZR1-OX2 (Figure 7, A and B), suggesting that the RNA-seq data is credible.
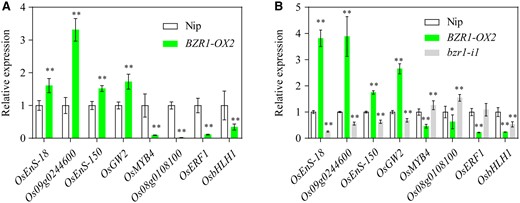
Validation of RNA-seq data for the representative target genes by RT-qPCR. A, RNA-seq data of eight representative DEGs in BZR1-OX2 seeds at 2 HAI. The gene expression levels in Nip were set to 1. B, Verification of the DEGs by RT-qPCR in both BZR1-OX2 and bzr1-i1 mutant seeds at 2 HAI. The gene expression levels in Nip were set to 1. Actin1 was used as the internal control for normalization of gene expression. Error bars represent sd (n = 3 experiments, each replicate contained 30 seeds). *P < 0.05; **P < 0.01 (Student’s t test).
All our data demonstrate that the BZR1-RAmy3D transcriptional module functions in both the rice endosperm and embryo to accelerate seed germination and post-germination growth (Figure 8).
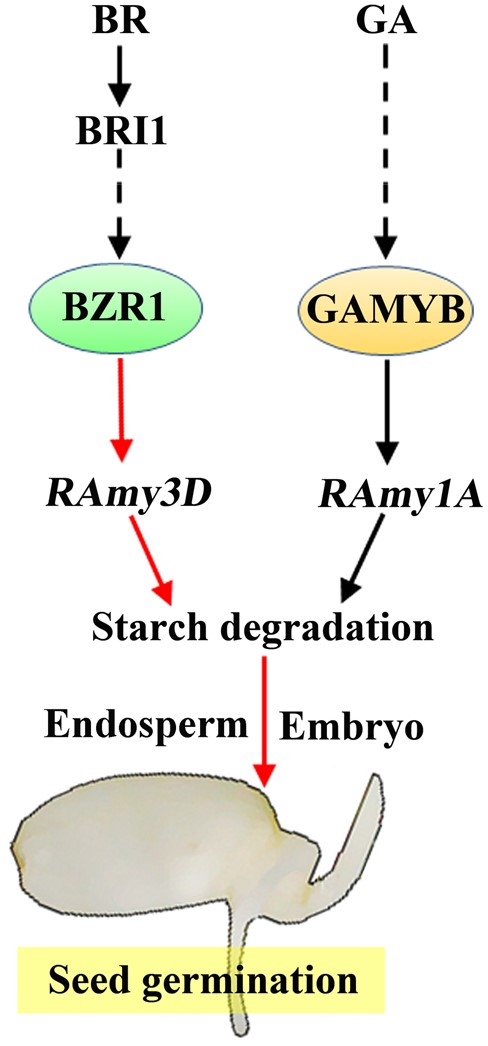
Working model for the BZR1-mediated transcriptional module in the regulation of rice seed germination. BR promotes germination in rice seeds via the key transcription factor BZR1 that mediates the expression of downstream target genes. One representative direct target of BZR1 is RAmy3D, a gene that encodes an important α-amylase that plays a crucial role in starch degradation at the initial stage of seed germination. The BZR1-RAmy3D transcriptional module, which is independent of the GAMYB-RAmy1A module, functions in both the endosperm and in embryo-related tissues. Moreover, both RAmy3D and RAmy1A promote starch turnover, thus providing more sugars for seed germination and embryo growth. Broken lines indicate indirect or multistep regulation. Arrowheads represent positive regulation.
Discussion
BR promotes seed germination and post-germination growth
Seed germination is closely correlated to robust and healthy seedling development, and hence influences the final crop yield. BR plays multiple roles in controlling a number of important agronomic traits in rice, including plant height, leaf angle, grain filling, grain shape, stress resistance, and seed germination. The BR-insensitive rice mutant d61, which has a mutation in the receptor BRI1, has shorter coleoptile and root lengths when germinated in the dark (Yamamuro et al., 2000), which is consistent with our data (Figure 1, A and B; Supplemental Figure S2). Moreover, d61 and the OsGSK2 overexpression line had shorter mesocotyl lengths, while OsGSK2-RNAi had longer mesocotyls (Sun et al., 2018). Furthermore, we also analyzed seed germination in several BR signaling mutants or transgenic rice lines. The results showed that the germination of d61, Go, and bzr1 rice seeds is similar, with delayed germination and shorter shoot length than their respective controls, while modulation of DLT had no observable effect on seed germination (Figures 1, 2, H and I). These results suggest that BR signaling regulates seed germination and post-germination growth via the BRI1-GSK2-BZR1 signaling cascade.
BZR1 enhances starch mobilization by promoting RAmy3D expression
Seed dormancy and germination are tightly controlled by multiple exogenous and endogenous stimuli (Finkelstein et al., 2008; SHu et al., 2016; Yang et al., 2020). Although a number of new mechanisms involved in seed germination have been discovered, such as post-translational modifications, RNA-binding protein, microRNAs, and alternative splicing, they are all directly or indirectly correlated to the mobilization of seed storage reserves (Müntz et al., 2001; Xue et al., 2021; Yu et al., 2021). In general, seed germination follows a classic triphasic model, and phase II is the most important stage during which all the metabolic reactions required by germination are reactivated, including starch hydrolysis and storage protein degradation (He and Yang, 2013). Although storage reserve degradation affects seed germination, the detailed regulatory mechanisms are still unclear. Our previous study showed that BR and GA co-regulate seed germination and embryo growth by modulating glutelin mobilization (Xiong et al., 2021). Starch is the most abundant component of the endosperm, accounting for 90% of the dry weight. Since the breakdown of starch into sugar provides substrates for rice embryo growth, the rate of degradation affects the germination speed. The slower consumption of starch and less soluble sugar production in TRS (a line with high resistant starch) endosperm delays seed germination (Pan et al., 2018). In maize, the coleoptile length of the starch branching enzyme 1a (sbe1a) mutant is reduced because more starch remains in the seeds during germination (Xia et al., 2011). Due to a mutation in Isoamylase 1 (ISA1), the preharvest sprouting 8 (phs8) rice mutant accumulates more sugar in the endosperm, which leads to the PHS phenotype (Du et al., 2018). These studies demonstrate a positive correlation between starch degradation and seed germination. In the present study, we have shown that blocking BR signaling can suppress α-amylase expression and activity (Figure 3; Supplemental Figure S6), thus delaying starch degradation (Supplemental Figure S7) and reducing the soluble sugar content (Supplemental Figure S8), and consequently inhibiting seed germination (Figure 2, H and I).
BZR1 is a key transcription factor that mediates the regulatory effect of BR on plant growth and development by directly binding to various downstream target genes. For example, BZR1 also promotes accumulation of OsmiR396d, which negatively regulates the expression of the rice growth-regulating factors 4 (OsGRF4) and OsGRF6 that regulate leaf angle and plant height, respectively (Tang et al., 2018). In addition, BZR1 directly suppresses the expression of the aldehyde dehydrogenase (OsALDH2B1) and allene oxide synthase (AOS2) genes to regulate the jasmonic acid pathway, thus modulating the growth-defense tradeoff in rice (Ke et al., 2020). In this study, we successfully identified RAmy3D as a reliable direct target of BZR1 (Figure 4). RAmy3D encodes an α-amylase with positive roles in controlling seed germination (Lu et al., 2007). The overexpression of RAmy3D promotes rice germination under normal conditions, which could be further enhanced in response to abiotic stress (Chen et al., 2019). In addition, our RNA-seq data also demonstrated that OsBZR1 can modulate the starch and sucrose metabolic pathways during seed germination (Supplemental Figure S16B).
The BZR1-RAmy3D module is essential for regulating seed germination by enhancing starch mobilization in both the rice embryo and endosperm
Our study identified a novel BR-regulated molecular cascade that modulates RAmy3D expression and starch degradation in rice. BZR1 binds directly to the E-box in the RAmy3D promoter and positively regulates its expression (Figure 4), thus increasing α-amylase activity and soluble sugar content (Figure 3; Supplemental Figure S8). Therefore, the BZR1-RAmy3D module functions in the rice endosperm to regulate storage starch degradation, which provides soluble sugars for embryo growth. However, it is still mostly unknown what happens after the sugars are transported into the embryo. A previous study showed that RAmy3D is expressed in both the embryo and aleurone during seed germination (Karrer et al., 1991). Transient starch will then be newly synthesized and will accumulate in the vascular tissue of the embryo when sufficient sugar is provided (Matsukura et al., 2000). In addition, more starch grains were observed in shoots of the GA-deficient mutant osko1 (ent-kaurene oxidase 1), leading to slower germination (Zhang et al., 2020). Interestingly, we also observed a similar transient starch accumulation phenotype in the BR insensitive mutants d61 and bzr1 (Figure 6, A–N). Moreover, the transient starch accumulation was unequal even within the same tissue, and regions with more starch granules had smaller cells (Figure 6, A–N; Supplemental Figure S14). This implies that the number of starch granules in the plumule cells is negatively correlated with starch mobilization efficiency and, consequently, cell size. When BR signaling is blocked, the stability and activity of BZR1 are attenuated (Li et al., 2012, 2018a), thus leads to decreased expression of RAmy3D and increased starch granules in the embryo (Supplemental Figure S15). The in vitro plumule elongation assay is useful to eliminate the different sugar flow from the endosperm, and it further demonstrated that the embryo also requires an intact BR signaling pathway to utilize glucose and promote embryo growth (Figure 6, O–R).
Furthermore, RAmy1A, another α-amylase gene, also plays crucial roles in promoting seed germination (Asatsuma et al., 2005). RAmy1A expression is directly induced by the transcription factor GAMYB (Washio, 2003). Our study clarified that the newly established BZR1-RAmy3D module is independent of the previously described GAMYB-RAmy1A module. First, the RAmy3D expression pattern is quite different from that of RAmy1A during the course of seed germination (Supplemental Figures S10 and S13). Second, BZR1 binds specifically only to the RAmy3D promoter, not the RAmy1A promoter (Figures 4 and 5A). The situation is opposite to that of GAMYB (Figures 4, C and 5, A–C). Finally, the germination speed and shoot length in the bzr1 mutant were further decreased in response to PAC treatment (Figure 5, E–G). Although the BZR1-RAmy3D and GAMYB-RAmy1A modules are relatively independent, crosstalk between BR and GA does exist. GA recovers germination in seeds deficient in BR biosynthesis by modulating glutelin mobilization (Li et al., 2020). When the BR concentration is low in rice, BZR1 promotes GA biosynthesis and subsequent cell elongation. However, when the BR concentration is high, the inhibitory feedback regulation of both BR and GA biosynthesis mediated by BZR1 is activated (Tong and Chu, 2018).
bzr1 can be used to prevent PHS
BR is considered to be a potential biotechnological target for enhancing crop yield and stress tolerance (Divi and Krishna, 2009; Gruszka et al., 2021). However, the pleiotropic effects of BR hinder its wide application in crop breeding programs (Khripach et al., 2000). For instance, direct modulation of BR biosynthesis or signaling will affect a series of agronomic traits in rice (Tong and Chu, 2018). Therefore, the dissection of downstream specific transcription factors or BR-responsive target genes will facilitate the application of BR in crop breeding with few adverse effects. At present, due to the decrease in arable agricultural land and the increased demand for food, breeding rice with compact plant architecture for dense planting is a feasible strategy to reduce the competition for water, light, and nutrients (Sakamoto et al., 2006). In maize, knocking down Related to ABI3/VP1-Like 1 (ZmRAVL1), a gene regulating BR biosynthesis, changed the endogenous BR content and the leaf angle, which increased yield at high density planting (Tian et al., 2019). In rice, the yield of the densely planted BR-deficient mutant osdwarf4-1 with erect leaves was 32.0% higher than that of the wild-type under conventional planting (Sakamoto et al., 2006). Moreover, although the 1,000-grain weight of d61-7, a weak BRI1 mutant, was about 21% lower than in wild-type, the yield was almost the same as that of wild-type under high-density planting (Morinaka et al., 2006). In the present study, knocking out OsBZR1 led to plants with compact architecture that were slightly reduced in height (Figure 2, F and G; Supplemental Figure S3, A and B), traits that meet the requirements of modern crop breeding programs. Although the 1,000-grain weight of the bzr1-i1 and bzr1-d4 mutants decreased by 8.4% and 6.9%, respectively (Supplemental Figure S3G), they have the potential to produce higher yields under appropriately dense planting conditions. Most importantly, PHS in the bzr1 mutants was notably lower than in the wild-type control, which will prevent great losses in rice yield and grain quality.
Materials and methods
Plant materials, growth conditions, and agronomic trait analysis
A number of BR-related rice materials (O. sativa ssp. japonica) were used in this study, including d61 and its wild-type TC65 (Yamamuro et al., 2000), Go, dlt, Do, and their wild-type ZH11 (Tong et al., 2012), BZR1-OX, bzr1, and their wild-type Nip. BZR1-OX and bzr1 are BZR1 overexpression and knockout mutant rice lines, respectively, which were generated in our laboratory. In brief, various BR signaling mutants are produced in different recipient rice varieties. Therefore, specific wild-type should be chosen and taken as control for corresponding BR signaling mutant in various experiments.
All rice plants were grown under the same climatic and management conditions during the summer in a paddy field at Yangzhou University (Yangzhou, Jiangsu province, China). At seed maturity, plant height and 1,000-grain weight were measured. Mature seeds from superior spikelets were collected for analysis of grain size and seed germination.
Transgene constructs and rice transformation
To construct the BZR1-OX vector, the BZR1 coding sequence (CDS) was cloned into the plant binary vector pCAMBIA1300 under control of the OsActin promoter and fused to the 3×FLAG epitope. To create the CRISPR/Cas9 gene editing vectors, the specific target site for BZR1 was designed and cloned into the pC1300-Cas9 vector (Shen et al., 2017; Xie et al., 2017). The verified constructs were then transferred into the japonica rice cultivars “Nip” via Agrobacterium tumefaciens-mediated transformation. All primers used here are listed in Supplemental Table S2.
Analysis of seed germination and in vitro embryo culture
The procedure for germination analysis has been described previously (Li et al., 2020). Seeds with a radicle >1 mm in length were considered to have successfully germinated (Cheng et al., 2013).
The method for in vitro culture of rice embryos was described previously (Xiong et al., 2021). In brief, the isolated embryos were cultured in 100-mM glucose or mannitol solution. Five days later, the shoot lengths were measured using ImageJ software.
To evaluate the level of PHS of BZR1-related materials, five superior rice panicles were collected, immersed in water, and incubated at 30°C under a 14-h/10-h light/dark cycle (Liao et al., 2018). The number of germinated seeds was counted and recorded every 2 d.
Lamina joint inclination assay
The lamina joint test was performed following a well-established procedure (Li et al., 2018b). After floating on distilled water for 24 h, the entire segments consisting of 1 cm of leaf blade and sheath were incubated in 2.5-mM maleic acid potassium solution containing 1-µM BL or dimethyl sulfoxide (DMSO; mock) for 48 h in the dark. The angles of 20 samples were then measured for each treatment.
Analysis of soluble sugar and total starch contents
The anthrone-H2SO4 colorimetric method was used to determine the soluble sugar content of the germinated seeds. The method is described in detail in Pan et al. (2018).
Total starch content of rice seeds was determined by using Megazyme Total Starch Assay Kit (K-TSTA). The detailed procedure follows the protocol of the kit.
Qualitative and quantitative analysis of seed α-amylase activity
To qualitatively evaluate the α-amylase activity of BR-related materials during germination, we used the starch plate test following the method of Liu et al. (2018) with slight modifications. The rice seeds were first sterilized with 70% (v/v) ethanol for 15 min and then washed twice with Milli-Q water. Next, the embryo-less half-seeds were placed on 2% (w/v) agar in Petri dishes with the cut face in tight contact with the agar. Instead of potato starch, rice starch was used here since it is the actual substrate of α-amylase in rice seeds. After incubation at 28°C for 4 d in the dark, the starch plate was soaked in iodine solution, 0.1% I2 (w/v) and 1% KI (w/v), for 5 min. Colorless halos around the half seeds form as a result of starch hydrolysis by α-amylase. The halo size is positively correlated with α-amylase activity.
Furthermore, the α-amylase activity was quantified using a colorimetric α-amylase test kit (Comin, Suzhou, Jiangsu, China). The major principle behind the kit assay is that amylase catalyzes starch hydrolysis into reducing sugars, and reducing sugars can change 3,5-dinitrosalicylic acid into a brown-red substance, which can then be measured by a UV–visible spectrophotometer.
RNA extraction, RT-qPCR assays, and RNA-Seq analysis
Total RNA was extracted from germinated seeds using the RNAprep pure Plant Kit (Tiangen, Beijing, China). Two-micrograms total RNA from each sample were pretreated with DNase I and used for reverse transcription. RT-qPCR was performed on a MyiQ real-time system (Bio-Rad, California, USA) with ChamQ Universal SYBR qPCR Master Mix (Vazyme Biotech, Jiangsu, China). OsActin1 was used as the internal reference for normalization. Three biological replicates were included for each sample, and the primer information is shown in Supplemental Table S2. Seeds germinated for 2 h were used for RNA-seq analysis as described by Zhu et al. (2021).
Western blotting
For BRZ treatment, the 5-d-old rice seedlings were grown in water supplied with BRZ (2 μM) or its mock DMSO for 5 d. For BL treatment, the leaves of 10-d-old rice seedlings were cultured in water containing BL (1 μM) or DMSO for 3 h. The samples were then collected and ground into powder in liquid nitrogen.
Total protein was extracted from rice leaf samples and heated at 95°C for 10 min, and separated by denaturing polyacrylamide gel electrophoresis (SDS–PAGE). After electrophoresis, the proteins were transferred to a poly(vinylidene fluoride) membrane and then immunodetected with antibodies recognizing the FLAG tag (F3165, Sigma-Aldrich) or heat shock protein (BPI; http://www.proteomics.org.cn).
ChIP-qPCR assay
The ChIP assay was performed following the published protocol of Saleh et al. (2008) with minor modifications. In brief, young panicles of BZR1-OX rice plants were fully ground into a powder with liquid nitrogen and suspended in buffer. After protein–DNA cross-linking with formaldehyde, the nuclei were isolated and the chromatin DNA was broken into 200- to 1,000-bp fragments by sonication. The DNA–protein complexes were immunoprecipitated with an anti-FLAG monoclonal antibody (1:200) and enriched using immunomagnetic beads. The collected complexes were de-crosslinked to release the DNA fragments which were used for subsequent qPCR analysis following purification.
ChIP-qPCR was performed as described previously (Tong et al., 2014). A region of the Actin1 intron was used for expression normalization, and a part of the 3′-untranslated region of the GA2ox-3 gene was used as the negative control. Each DNA region test was repeated three times. The primers used are shown in Supplemental Table S2.
Dual-luciferase reporter assay
The Agrobacterium-mediated transient assay system was used to co-express the reporter and effector constructs in Nicotiana benthamiana leaf epidermal cells (Zhu et al., 2021). To generate reporter constructs, 2-kb promoter regions upstream of the RAmy1A and RAmy3D genes were individually cloned to generate effector constructs, and the CDSs of OsBZR1 and OsGAMYB were each cloned into the pGreenII 62-SK reporter vector. Both a reporter and an effector construct were then introduced together into N. benthamiana leaves by agroinfiltration. Forty hours later, the infiltrated leaves were used for transcriptional activity assays. The firefly and Renilla luciferase signals were examined using the Dual Luciferase Reporter Assay Kit (Vazyme, Jiangsu, China).
EMSA
The OsBZR1 CDS was cloned to generate the MBP–BZR1 fusion gene. The MBP–BZR1 recombinant protein was then induced with 0.5-mM IPTG in Escherichia coli strain BL21 and purified using the PurKine MBP-Tag Protein Purification Kit (Abbkine, Hubei, China). The probe was labeled with biotin using the DNA 3′-End Biotinylation Kit (Thermo Scientific). The unlabeled probe was used as the competitor. The detailed procedure is described in Wu et al. (2019).
Yeast two-hybrid assay
The CDSs of OsBZR1 and OsGAMYB were cloned into the pGBKT7 (BD) and pGADT7 (AD) vectors, respectively. The BZR1-BD and GAMYB-AD plasmids were then co-transformed into the yeast (Saccharomyces cerevisiae) strain AH109 and the positive transformants were then screened for protein–protein interactions.
Sectioning and iodine staining shoots of germinating seeds
The shoots of germinating seeds 36 HAI were harvested and immediately fixed in a solution consisting of 0.1-M Na-phosphate buffer and 2.5% glutaraldehyde. The fixed samples were soaked in phosphate buffer, dehydrated in an ethanol series, and then embedded in LR white resin. The samples were cut into 1.8-μm semithin sections using a Leica Ultrathin Microtome (EM UC7). Finally, the sections were stained with iodine solution, 0.1% I2 (w/v) and 1% KI (w/v), and observed for the presence of starch.
Statistical analysis
In this study, most statistics were presented as mean ± sd. For experiments with single pairwise comparisons, Student’s t test was used to determine the level of significance (*P < 0.05, **P < 0.01). For experiments with multiple comparisons, the data were analyzed with Duncan’s multiple range test at P < 0.05 (with different letters).
Accession numbers
Genes sequence information in this study could be found in the Rice Annotation Project Database (RAP-DB) (https://rapdb.dna.affrc.go.jp/) under the following accession numbers: OsBZR1 (Os07g0580500), RAmy1A (Os02g0765600), RAmy1B (Os01g0357400), RAmy1C (Os02g0765400), RAmy2A (Os06g0713800), RAmy3A (Os09g0457400), RAmy3B (Os09g0457600), RAmy3C (Os09g0457800), RAmy3D (Os08g0473900), RAmy3E (Os08g0473600), GAMYB (Os01g0812000), GA2ox-3 (Os01g0757200), OsEnS-18 (Os01g0805400), OsEns-150 (Os12g0464400), OsGW2 (Os02g0244100), OsMYB4 (Os04g0517100), OsERF1 (Os04g0546800), OsbHLH1 (Os01g0928000).
Supplemental data
The following materials are available in the online version of this article.
Supplemental Figure S1. Phenotypes of BR-insensitive mutants and their corresponding wild-types, including d61 and Taichung 65 (TC65), Go, Do, dlt, and Zhonghua 11 (ZH11).
Supplemental Figure S2. Morphological changes in seeds of BR-insensitive mutants at 96 HAI.
Supplemental Figure S3. Phenotypes of BZR1-related rice mutants and overexpression transgenic lines.
Supplemental Figure S4. Plant height in the 2-week old rice seedlings.
Supplemental Figure S5. Morphological changes in seeds of BZR1-OX, bzr1-i1, and wild-type Nip at 96 HAI.
Supplemental Figure S6. Analysis of α-amylase activity in BZR1-OX2, bzr1-i1, and wild-type Nip seeds.
Supplemental Figure S7. Starch contents of germinating seeds of BR-related rice lines and the corresponding wild-type controls at 2 HAI and 96 HAI.
Supplemental Figure S8. Soluble sugar contents of germinating seeds of BR-related rice lines and the corresponding wild-type controls at 2 HAI and 96 HAI.
Supplemental Figure S9. Analysis of the expression pattern of BZR1 in germinating rice seeds from 2 to 96 HAI.
Supplemental Figure S10. Analysis of the expression pattern of RAmy3D in germinating rice seeds from 2 to 96 HAI.
Supplemental Figure S11. Schematic maps of the reporter and effector constructs used in the dual-luciferase reporter assay.
Supplemental Figure S12. The OsBZR1 protein binds to the P3 region of the promoter as shown by EMSA.
Supplemental Figure S13. Analysis of RAmy1A expression in germinating rice seeds from 2 to 96 HAI.
Supplemental Figure S14. Quantitative data of the length and width of cells in the shoots of d61, bzr1-i1 mutants and the corresponding wild-type controls.
Supplemental Figure S15. RT-qPCR analysis of RAmy3D expression in embryos of d61, bzr1-i1 and their corresponding wild-types at 6 HAI.
Supplemental Figure S16. Transcriptomic analysis of BZR1-OX transgenic rice seeds.
Supplemental Table S1. The DEGs in BZR1-OX2 transgenic rice compared to WT by RNA-seq.
Supplemental Table S2. Primers used in this study.
Q.F.L., Q.Q.L., and M.X. conceived the project and designed the experiments. J.Y., J.W., L.H., and C.C. generated and analyzed the transgenic rice plants. M.X., J.Y., J.W., and Q.G. performed the other experiments. M.X., C.Z., X.F., D.Z., Q.Q.L., and Q.F.L. analyzed the data. M.X., Q.Q.L., and Q.F.L. wrote the manuscript. Q.Q.L, and Q.F.L. revised the manuscript.
The author responsible for distribution of materials integral to the findings presented in this article in accordance with the policy described in the Instructions for Authors (https://dbpia.nl.go.kr/plphys/pages/general-instructions) is: Qian-Feng Li ([email protected])
Acknowledgments
We thank H.N. Tong (Institute of Crop Sciences, Chinese Academy of Agricultural Sciences) and C.C. Chu (Institute of Genetics and Developmental Biology, Chinese Academy of Sciences) for providing the dlt mutant as well as Go and Do transgenic rice.
Funding
This work was supported by the National Natural Science Foundation of China (31825019, 32071984), Science Fund for Distinguished Young Scholars of Jiangsu Province (BK20200045), Jiangsu Six Talent Peaks (SWYY-154), other programs from Jiangsu Province Government (PAPD, Qinglan, and “333”).
Conflict of interest statement. None declared.
References
Author notes
Senior authors.
These authors contributed equally (M.X., J.Y., J.W.).