-
PDF
- Split View
-
Views
-
Cite
Cite
Yaxin Li, Huan Liu, Xuehui Yao, Jiang Wang, Sheng Feng, Lulu Sun, Si Ma, Kang Xu, Li-Qing Chen, Xiaolei Sui, Hexose transporter CsSWEET7a in cucumber mediates phloem unloading in companion cells for fruit development, Plant Physiology, Volume 186, Issue 1, May 2021, Pages 640–654, https://doi.org/10.1093/plphys/kiab046
- Share Icon Share
Abstract
In the fleshy fruit of cucumbers (Cucumis sativus L.), the phloem flow is unloaded via an apoplasmic pathway, which requires protein carriers to export sugars derived from stachyose and raffinose into the apoplasm. However, transporter(s) involved in this process remain unidentified. Here, we report that a hexose transporter, CsSWEET7a (Sugar Will Eventually be Exported Transporter 7a), was highly expressed in cucumber sink tissues and localized to the plasma membrane in companion cells of the phloem. Its expression level increased gradually during fruit development. Down-regulation of CsSWEET7a by RNA interference (RNAi) resulted in smaller fruit size along with reduced soluble sugar levels and reduced allocation of 14C-labelled carbon to sink tissues. CsSWEET7a overexpression lines showed an opposite phenotype. Interestingly, genes encoding alkaline α-galactosidase (AGA) and sucrose synthase (SUS) were also differentially regulated in CsSWEET7a transgenic lines. Immunohistochemical analysis demonstrated that CsAGA2 co-localized with CsSWEET7a in companion cells, indicating cooperation between AGA and CsSWEET7a in fruit phloem unloading. Our findings indicated that CsSWEET7a is involved in sugar phloem unloading in cucumber fruit by removing hexoses from companion cells to the apoplasmic space to stimulate the raffinose family of oligosaccharides (RFOs) metabolism so that additional sugars can be unloaded to promote fruit growth. This study also provides a possible avenue towards improving fruit production in cucumber.
Introduction
The development and growth of sink tissues such as fruit, flower, and root depend on sugar supplied from source tissues (Patrick, 1997). The transport of sugars from source leaves to sink organs occurs through three main steps: phloem loading (Zhang and Turgeon, 2018), phloem transport (Jensen, 2018), and phloem unloading (Milne et al., 2018). Phloem unloading is the process by which photoassimilates leave the sieve element/companion cell (SE/CC) complex in sink tissues by one of two pathways: flowing into phloem parenchyma cells through plasmodesmata (symplasmic unloading) or transport through the apoplasm mediated by a protein carrier (apoplasmic unloading; Oparka, 1990).
In sink tissues, like seeds, the filial tissues (embryo and endosperm) are symplasmically isolated from maternal plants (Milne et al., 2018). Earlier biochemical experiments suggested that about half of the sucrose is unloaded by a sucrose/proton antiport from the seed coat of bean (Vicia faba L.; Fieuw and Patrick, 1993), and the rest is likely unloaded by a facilitator mechanism (Zhou et al., 2007). SWEET (Sugar Will Eventually be Exported Transporter) proteins function as facilitators using different sugars as substrates (Chen et al., 2010). Due to their ability to export sugars, they have drawn extensive attention to biological questions directly or indirectly related to phloem unloading (Eom et al., 2015; Milne et al., 2018). In Arabidopsis (Arabidopsis thaliana), AtSWEET11, 12 and 15, belonging to clade III SWEETs that mainly transport sucrose, function in seed filling, as the atsweet11;12;15 mutant show increased starch accumulation in seed coat and reduced lipid content in seeds (Chen et al., 2015). Similarly, rice (Oryza sativa) caryopses highly express OsSWEET11 and 15, which are responsible for sucrose efflux from nuclear epidermis and the ovular vascular to the apoplasm (Yang et al., 2018). Maize (Zea mays) ZmSWEET4c (clade II SWEETs that transport hexose) mediates hexose transport across the basal endosperm transfer layer and is essential for seed filling and basal endosperm transfer layer (BETL) differentiation (Sosso et al., 2015). Soybean (Glycine max) GmSWEET15 is essential for embryo development due to its critical role of exporting sucrose from the endosperm to the embryo during early seed development (Wang et al., 2019). GmSWEET10a and GmSWEET10b (clade III), are specifically expressed in the seed coat, where they contribute to sugar transport to the embryo, and were the targets of stepwise selection during soybean domestication, leading to simultaneous changes in seed size, oil content and protein content (Wang et al., 2020). AtSWEET9 (clade III), is responsible for nectar secretion (Lin et al., 2014). In the potato (Solanum tuberosum) tuber, StSWEET11, together with the Flowering Locus T (FT) homolog Self-Pruning 6A (StSP6A), regulates source-sink communication and sugar partitioning (Abelenda et al., 2019). In some species that produce a fleshy fruit (e.g., cucumber, apple, tomato, and grape), their primary harvestable sink organs use the apoplasmic phloem unloading strategy (Ruan and Patrick, 1995; Zhang, et al., 2004; 2006; Hu et al., 2011). However, few evidence was shown with respect to which transporter(s) is responsible to export sugar from SE/CC to apoplasmic space in fruit phloem unloading. Recently, MdSWEET15a and 9b were associated with QTLs for sugar content and may regulate sugar accumulation in apple (Malus domestica) fruit (Zhen et al., 2018). The expression levels of grape (Vitis vinifera) VvSWEET11 and 15 increased during grape maturation (Breia et al., 2020). However, direct lines of evidence supporting the biological functions of SWEETs in fruit development are still missing.
Many species transport carbohydrates within their phloem as sucrose (such as Arabidopsis and most crops), some species transport sugar alcohols (such as rose and apple), and some species transport raffinose family of oligosaccharides (RFOs), such as cucumber and Alonsoa meridionalis (Rennie and Turgeon, 2009). For sucrose-transporting species, the unloaded sugar is also sucrose (Braun et al., 2014). However, it remains unknown what sugars are unloaded from species using RFOs and what mechanism is responsible for the unloading process.
In cucumber (Cucumis sativus ) fruit, where an apoplasmic phloem unloading strategy is employed (Hu et al., 2011), the dominant sugars are glucose and fructose (Hu et al., 2009), although the majority of transported sugars are raffinose and stachyose. Stachyose and raffinose are likely hydrolyzed to sucrose by α-galactosidase (α-Gal) and then converted into hexoses by sucrose synthase (SUS) or invertase (INV; Ma et al., 2019a). The invertase enzymes can be divided into two families: neutral/alkaline or cytoplasmic invertases (CINV) and acid invertases (either a soluble form residing in the vacuole, VINV, or a form tightly bound to the cell wall, CWINV; Wan et al., 2018). Therefore, one would assume that RFOs may be metabolized into sucrose and hexose before they reach the fruit parenchyma cells. However, it is unknown which form of sugar is exported from the SE/CC and which protein carriers mediate sugar efflux into the apoplasm from the phloem of main vascular bundle (MVB) in the fruit (Sui et al., 2018).
Based on the cumulative knowledge about the roles of SWEETs in phloem unloading, the CsSWEETs were our best candidates for sugar transport within cucumber fruit. Seventeen cucumber CsSWEET genes have been identified, and some are expressed in the fruit (Li et al., 2017b). Here, we report that CsSWEET7a was highly and specifically expressed in the phloem of the cucumber sink tissues, including fruit, flower, and root. We found that CsSWEET7a is a hexose transporter that is exclusively localized to the plasma membrane in the phloem companion cells, and contributes to carbohydrate allocation in sink organs, especially the cucumber fruit. The regulatory model involving the synergy of SWEET transporter and soluble sugar hydrolases (α-Gal, SUS, and INV) herein provides new insights into the molecular action of sugar transports that modulates fruit development, which has potential agricultural application to enhance fruit yield.
Results
Spatiotemporal expression analysis of CsSWEET7a
In previous research, 17 genes in the SWEET family were identified in cucumber and classified into four clades (Hu et al., 2017; Li et al., 2017b). RNA sequencing (RNA-Seq) data showed that CsSWEET7a was exclusively expressed in the vascular bundle in the fruit with an mRNA level approximately 100 times higher than other SWEET family genes (Supplemental Figure S1). In this paper, we isolated CsSWEET7a (Csa4G054810, belonging to clade II; Li et al., 2017b) from a cDNA library constructed using vascular bundle tissue of cucumber fruit (Sui et al., 2018).
RT-qPCR was used to determine more detailed spatiotemporal expression patterns of CsSWEET7a (Figure 1A-D). CsSWEET7a was strongly expressed in the sink tissues, including the root, male and female flowers, and fruit (Figure 1A). The cucumber fruit includes the peduncle, gynophore, the transition tissue that does not contain seeds, and the fruit, which is divided into exocarp, main vascular bundle, mesocarp, and placenta (Figure 1B). CsSWEET7a was expressed in the peduncle, gynophore, and the whole fruit sample (Figure 1C). Within the fruit, there are four types of vascular bundles, the bicollateral main vascular bundle (MVB), the peripheral vascular bundle (PeVB), the collateral carpel vascular bundle (CVB), and the placental vascular bundle (PlVB; Sui et al., 2018). Of these four, MVB is most likely the major site where assimilate/sugar is unloaded in cucumber fruit. CsSWEET7a was more highly expressed in MVB (Figure 1C) compared to other fruit tissues. From 2 d before anthesis until the marketable mature stage on the 9th d after anthesis (9 DAA), the transcriptional level of CsSWEET7a increased over time in the peduncle, gynophore, and fruit (Figure 1D). Substantially, a large increase in the mRNA level of CsSWEET7a was detected in the MVB from 3 DAA to 9 DAA (Figure 1D), which coincided with the fastest growth stage of the fruit (Hu et al., 2011, Ando et al., 2012, Sui et al., 2017). These data suggested that the transcriptional level of CsSWEET7a was positively corresponded with development of cucumber fruit, indicating that CsSWEET7a may play a role in carbohydrate translocation during phloem unloading in sink tissues.
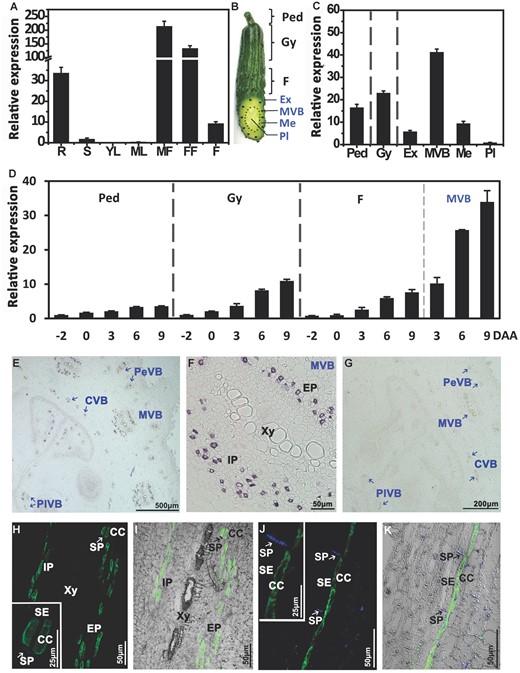
Spatial and temporal expression of CsSWEET7a in cucumber. A, The expression pattern of CsSWEET7a in the whole plant by reverse transcription quantitative PCR. R, root; S, stem; YL, young leaf; ML, mature leaf; MF, male flower; FF, female flower; F, marketable mature fruit (9 d after anthesis, DAA). B, Cucumber fruit, showing the sampling sites: Ped, Peduncle; Gy, Gynophore; Ex, Exocarp; MVB, main vascular bundle; Me, Mesocarp; Pl, Placenta. C, Expression of CsSWEET7a in 6 tissues of 9 DAA fruit. D, Expression of CsSWEET7a during the development of the peduncle, gynophore, fruit, and fruit MVB, from 2 d before anthesis to 9 DAA. E-K, Immunohistochemical localization of CsSWEET7a in cucumber fruit (E-I) and peduncle (J and K). E, Transverse sections of cucumber fruit with alkaline phosphatase (AP) as secondary antibody. F, Close-up of the MVB region in (E). G, Sections incubated with pre-immune serum as control. H-K, Longitudinal sections of cucumber fruit MVB (H and I) and peduncle VB (J and K) with fluorescein isothiocyanate (FITC) as secondary antibody. The boxed insets in (H and J) were the close-up images of the SE/CC region. The sieve plate (SP) emits blue fluorescence due to aniline blue staining. White or black arrows indicate SP. SE, sieve element; CC, companion cell; Xy, xylem; Ph, phloem; EP, external phloem; IP, internal phloem; PeVB, CVB, and PlVB, peripheral, carpel, and placental vascular bundle, respectively. Mean values ± SE of three independent biological replicates are given.
CsSWEET7a is a plasma membrane protein in companion cells
Due to the high expression of CsSWEET7a in the cucumber fruit, especially the MVBs, we examined the cell-type-specific accumulation of CsSWEET7a protein in the cucumber fruit (Figure 1, E-K). A polyclonal antibody was generated using two CsSWEET7a-specific peptides (TKLQKEEREGKGQVVLS and KNDNIESGNPFAEVHGA; Supplemental Figure S2). The recognition and specificity of the antibody was tested using protein extracts from yeast expressing CsSWEET7a (Supplemental Figure S3A) and from fruit vascular tissue (Supplemental Figure S3B). A consistent band with a size of 28.9 kDa was detected in the protein extracted from the yeast transformed with CsSWEET7a and the membrane protein fraction from fruit vascular tissue, while no bands were detected from either yeast harboring an empty vector (Supplemental Figure S3A) or the soluble proteins from the fruit vascular tissue (Supplemental Figure S3B). Thus, this antibody specifically recognizes CsSWEET7a.
Results from immunohistochemical staining using the anti-CsSWEET7a antibody and an alkaline phosphatase (AP) conjugated secondary antibody showed a strong immunolabelling signal in the vascular bundle (VB) region of the fruit (Figure 1E), including the MVB, PeVB, CVB and PlVB. The signal was observed in both the external (EP) and internal phloem (IP) of MVB (Figure 1F), which is consistent with the detected expression of CsSWEET7a in EP and IP regions (Supplemental Figure S1). Aniline blue counterstaining, a fluorescent dye that binds callose, revealed discrete deposits of sieve plates, which is a significant marker of sieve elements (SEs; Palmer et al., 2015; Figure 1, H-K). Using a FITC-labeled secondary antibody, CsSWEET7a was detected exclusively in the perimeter of cells adjacent to sieve element cells, which are likely to be companion cells (CCs), in fruit MVB (Figure 1, H and I) and peduncle VB (Figure 1, J and K).
In A. thaliana protoplasts expressing CsSWEET7a-GFP, CsSWEET7a was localized to the plasma membrane (PM; Figure 2A). In onion (Allium cepa) epidermal cell (Figure 2B) and Nicotiana benthamiana pavement cell (Figure 2C), CsSWEET7a-GFP co-localized with the mCherry-labeled plasma membrane marker (PM-rk; CD3-1007). Additionally, CsSWEET7a was clearly located on the outer side of the nuclei (Figure 2C) as delineated by the RFP-labeled nuclei marker H2B (Martin et al., 2009). Together, these subcellular localizations and immunohistochemical analyses suggested that CsSWEET7a may function on the plasma membrane of companion cells in the phloem region.
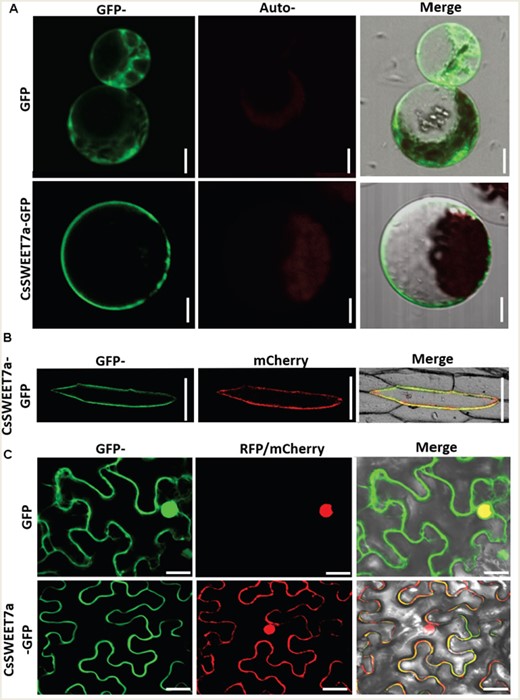
Subcellular localization of a CsSWEET7a fusion with green fluorescent protein (GFP) in A. thaliana protoplasts (A), in onion epidermal cell (B) and in N. benthamiana pavement cell (C). An mCherry-labeled plasma membrane (PM) marker (PM-rk; CD3-1007) and RFP-labeled nuclei marker (H2B) were used to mark the plasma membrane and nuclei position, respectively. Scale bars = 10 μm in (A), 50 μm in (B) and 20 μm in (C).
Heterologous expression and characterization of CsSWEET7a in yeast
To determine the substrates of CsSWEET7a, functional characterization of CsSWEET7a was performed by expressing CsSWEET7a in the hexose uptake-deficient yeast mutant EBY.VW4000 (Wieczorke et al., 1999), which lacks 17 hexose transporters. A spotting assay showed that EBY.VW4000 transformants with CsSWEET7a/pDR196 grew well when given 2% (w/v) glucose or galactose but grew only slightly better than the negative control when given 2% (w/v) fructose or mannose (Supplemental Figure S4). The uptake capacity of CsSWEET7a was concentration dependent (Figure 3A). CsSWEET7a was a low-affinity transporter for glucose (Km = 10.0 ± 0.8 mM, Figure 3B), which is consistent with other measured Km values of SWEETs that show them to be low affinity transporters.
![Functional and kinetic characterization of CsSWEET7a in yeast. A, Concentration-dependent [14C]Glucose uptake rate. FW, fresh weight. B, Kinetic analysis of CsSWEET7a. The Km was 10.0 ± 0.8 mM. C, Substrate specificity of CsSWEET7a expressed in EBY.VW4000. Substrate specificity was determined by competitive inhibition of [14C]Glucose uptake. The sample without competitor was the control, the concentration ratio of the competing sugar relative to that of glucose was 10. The values are presented as units relative to the values from the control, taken as 100%. Mean values ± SE of three independent biological replicates are given. Significance compared to control was determined by Student’s t test at *P < 0.05, **P < 0.01, and ***P < 0.001.](https://oup.silverchair-cdn.com/oup/backfile/Content_public/Journal/plphys/186/1/10.1093_plphys_kiab046/2/m_kiab046f3.jpeg?Expires=1750303821&Signature=n~KIMo82jmfgA~jB0qgDxQAi6Zykvci0tYzWIgPPr55CaLT98XpVKRpDWqTUX9YmHg2jTWnRQuj6OtcoXl5PVj7LJ4Hb99I-hcEnSlqGmOQFr-FLQzPVBomcdtc15GUzeVtCQSolpgETsgcvruUjELgtwo1o2NFPSlcEEo0vbitMa9l0FNq7ddmDYBPNujr8bsIfuUrBdeCT-eRohJ7Htzy0Zien1tnGnKmYJyfC50cjAx1~dpCOcXcgG7eIILa~3EupYpWryuCSuDFTvO--uMTrul7y3COdtEIIt9~WJW22xYHPcsC5Eo~Vep-NXIiWalB8whkvIjWohbHh6Gra9g__&Key-Pair-Id=APKAIE5G5CRDK6RD3PGA)
Functional and kinetic characterization of CsSWEET7a in yeast. A, Concentration-dependent [14C]Glucose uptake rate. FW, fresh weight. B, Kinetic analysis of CsSWEET7a. The Km was 10.0 ± 0.8 mM. C, Substrate specificity of CsSWEET7a expressed in EBY.VW4000. Substrate specificity was determined by competitive inhibition of [14C]Glucose uptake. The sample without competitor was the control, the concentration ratio of the competing sugar relative to that of glucose was 10. The values are presented as units relative to the values from the control, taken as 100%. Mean values ± SE of three independent biological replicates are given. Significance compared to control was determined by Student’s t test at *P < 0.05, **P < 0.01, and ***P < 0.001.
To determine the substrate specificity of CsSWEET7a, a competition experiment was conducted using [14C]Glu with another sugar. This assay showed that glucose and galactose were the favored substrate among all tested sugars. The uptake of [14C]Glu in yeast was inhibited strongly by either glucose or galactose, slightly by fructose or mannose, but barely at all by sucrose, raffinose or stachyose (Figure 3C). These results showed that CsSWEET7a not only functions as a glucose transporter with a presumably low affinity but also transports other hexoses, including galactose, fructose, and mannose.
CsSWEET7a is involved in cucumber fruit development
As a fresh fruit product, cucumber is usually harvested around 9 DAA (marketable mature stage). However, cucumber fruit length, diameter, and cell area will keep increasing until 15 DAA (Yang et al., 2013). Coincidentally and interestingly, the transcript level of CsSWEET7a kept increasing from 9 DAA to 15 DAA (Figure 4A). To further test the function of CsSWEET7a in fruit, we generated multiple CsSWEET7a overexpression (OE) lines and RNAi lines (Supplemental Figure S5, A–C). The CsSWEET7a expression level significantly increased in the vascular bundle of either 9 DAA (Supplemental Figure S5D) or 15 DAA fruits (Figure 4B) in the OE lines, and it was significantly reduced in the RNAi lines compared to wild type plants.
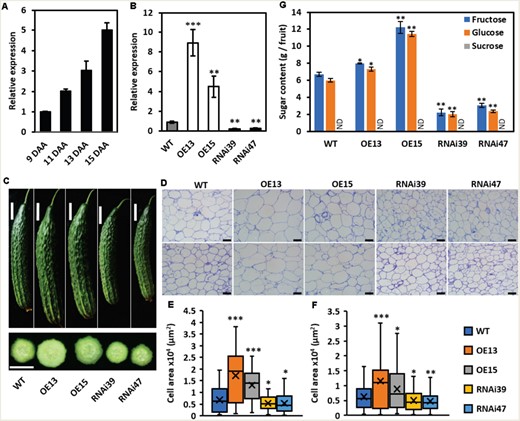
Phenotype of fruit at 15 DAA in CsSWEET7a transgenic lines. A, Transcriptional levels of CsSWEET7a in fruit vascular bundle of wild type (WT) from 9 DAA to 15 DAA. B, Transcriptional levels of CsSWEET7a in fruit vascular bundle of WT, overexpression (OE), and RNA interference (RNAi) transgenic lines at 15 DAA. C, Phenotype of fruit at 15 DAA in WT and transgenic lines. Morphology of fruits (top) and hand cross section of fruits (bottom). D, Paraffin cross (top) and longitudinal sections (bottom) of mesocarp of fruit at 15 DAA. E and F, Cell area of mesocarp cells in cross (E) and longitudinal sections (F) in WT and transgenic lines at 15 DAA. Cell area was measured by ImageJ. More than 100 cells were measured for each line. Center line, median cell area; ‘X’, average cell area; box limits, upper and lower quartiles; whiskers, 1.5x interquartile range. G, Sugar content of 15 DAA fruit. Mean values ± SE of three independent biological replicates are given in (A, B, G). Significance compared to WT was determined by Student’s t test at *P < 0.05, **P < 0.01, and ***P < 0.001. Scale bars = 5 cm in (C), 100 μm in (D). ND, data was not detected.
Furthermore, compared to the wild type, the CsSWEET7a-OE lines produced bigger fruit in both size and weight, while the RNAi lines yielded smaller and lighter fruits at both 9 DAA (Supplemental Figure S5, E, and F) and 15 DAA stage (Figure 4C). The increased area of individual mesocarp cells in the OE lines and the decreased cell area in RNAi lines agree with the morphological phenotypes (Figure 4, D–F).
Previous reports showed that the dominant sugars accumulated in the mature cucumber fruit are monosaccharides (Handley et al., 1983; Hu et al., 2009). Our measurements showed that glucose and fructose were the major sugars, while only a trace amount of sucrose was detected in 9 DAA fruit, and sucrose was undetectable in 15 DAA fruit (Supplemental Figure S5G and S6; Figure 4G). Moreover, the amount of glucose and fructose per fruit significantly increased in the CsSWEET7a-OE lines but decreased in the CsSWEET7a-RNAi plants compared to wild type plants in both 9 DAA (Supplemental Figure S5G) and 15 DAA fruits (Figure 4G).
CsSWEET7a participates in carbohydrate distribution in sink organs
To directly examine how CsSWEET7a affects photoassimilate allocation and phloem unloading in sink tissues, especially in fruit, 14CO2 feeding assay was conducted using CsSWEET7a overexpression and RNAi lines at the 4-node stage without fruits and 15-node with fruits. At 28 h after 14CO2 labeling for 20 min, we quantified the amount of the 14C incorporated into the different tissues using a scintillation counter (Figure 5).
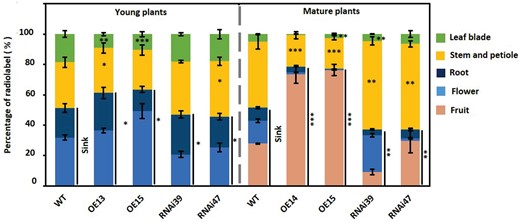
Distribution of 14C-labeled carbohydrate in wild type and CsSWEET7a-transgenic lines. The distribution of 14C-carbohydrate in young (with 4 nodes) and mature (with 15 nodes) plants. Sinks in the young plants included root and flower, and in the mature plants included root, flower, and fruit. Mean values ± SE of three independent biological replicates are given. Significance compared to WT was determined by Student’s t test at *P < 0.05, **P < 0.01, and ***P < 0.001.
In the young wild type plants, 14C-labeled photoassimilates were allocated into flowers (34%) and roots (20.7%) as the major sink tissues, whereas in mature plants 27.5% of the 14C-labeled assimilates were allocated to fruits, followed by flower (14.6%) and root (8.6%; Figure 5), which indicates that the demands for sugar import among the different sink tissues vary at different development stages. Compared to wild type plants, more 14C-labeled photoassimilates were transported to flowers and roots in young OE plants (a total of 62.4% compared to 54.7% in WT), and 77.2% of the fixed-14C entered sink tissues of the mature plants, with a significantly high proportion in the fruit (up to 74.4%; Figure 5). By contrast, the allocation of fixed 14C into sink tissues was reduced dramatically in the CsSWEET7a-RNAi lines regardless of the developmental stages (Figure 5). Notably, a large amount of 14C-labeled carbohydrate remained in the stem and petiole, the main transport system, whether in young or mature plants of RNAi lines (Figure 5). Taken together, these results demonstrated that CsSWEET7a plays a critical role in allocating sugar among the whole plant and particularly affects carbohydrate flow into the fruit.
CsSWEET7a may participate in apoplasmic hexose unloading in cucumber fruit
As we demonstrated, CsSWEET7a functions as a hexose transporter and is localized specifically on the plasma membranes of companion cells. To understand how cucumber makes glucose and fructose available for CsSWEET7a to export from companion cells of the fruit, we examined whether the key enzymes responsible for stachyose and raffinose metabolism colocalize with CsSWEET7a. The genes encoding RFO catabolism enzymes, such as alkaline α-galactosidase 1 (AGA1), alkaline α-galactosidase 2 (AGA2), sucrose synthesis 4 (SUS4), and some invertases (like CINV1, CINV2, and CWINV3), are widely expressed in the phloem of cucumber fruit (Sui et al., 2018, Fan et al., 2019). A previous study (Ohkawa et al., 2010) has shown that raffinose and stachyose start their break-down into sucrose and hexose at cucumber peduncle, which creates the need for AGA and SWEET7a. Moreover, the cells of vascular bundle in peduncle are compactly organized, which is highly favorable to achieve consecutive sections with clear vascular bundle signatures.
Next, in consecutive tissue sections from the peduncle, immunohistochemical analysis showed that CsAGA1 and CsAGA2 were detected in some small cells of phloem tissues (Figure 6, B and C), which partially overlapped with the cells where CsSWEET7a was localized (Figure 6, A and D). As CsSWEET7a is localized in companion cells (Figure 1, H-K), CsAGA1 and/or CsAGA2 may also be expressed accordingly in companion cells. Longitudinal sections of cucumber peduncle showed that CsAGA1 protein localized primarily in the cytosol of vascular bundle cells (Figure 6, E-G). Furthermore, the aniline blue counterstaining, a marker of sieve elements (Palmer et al., 2015), demonstrated that AGA2 protein was distributed exclusively in the companion cells in both peduncle VB and fruit MVB (Figure 6, H-K). Consistently, the transcript (Sui et al., 2018) and protein (Fan et al., 2019) of CsSUS4 are reported to be present in cucumber peduncle and fruit vascular bundles, especially in the external phloem of the fruit MVB, which is involved in sugar breakdown and indicative of phloem unloading in the fruit (Sui et al., 2018).
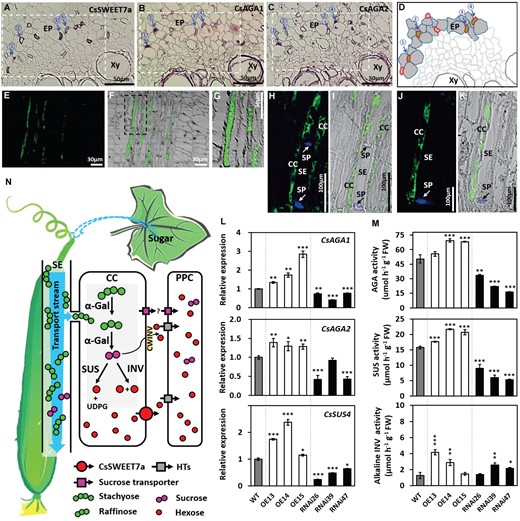
CsSWEET7a collaborates with other sugar metabolic enzymes in sugar unloading and allocation in phloem tissues of cucumber fruit. A-C, Immunohistochemical co-localization of CsSWEET7a (A), and alkaline α-galactosidase 1 (CsAGA1) (B) and alkaline α-galactosidase 2 (CsAGA2) (C) in the phloem of cucumber peduncle. Consecutive cross sections with alkaline phosphatase (AP) as secondary antibody. The consecutive sections came from the same wax-embedded sample and were incubated with different primary antibodies. Blue arrows with the same number indicate the same cell. D, Schematic diagrams showing representative phloem cell images from regions marked with squares in (A, B and C). The red and green color indicate SWEET7a and α-galactosidase localization, respectively. Both exist in companion cells. E-K, Immunohistochemical localization of CsAGA1 in the peduncle VB (E-G), and CsAGA2 in the phloem of peduncle VB (H and I) and fruit MVB (J and K). Longitudinal sections with fluorescein isothiocyanate (FITC) as secondary antibody. The sieve plate (SP) emits blue fluorescence due to aniline blue staining in (H to K). L, Expression levels of genes encoding alkaline α-galactosidase (CsAGA1 and CsAGA2) and sucrose synthase (CsSUS4) in fruit phloem region of wild type (WT) and transgenic lines. M, Enzyme activities of AGA, SUS, and neutral/alkaline invertase (cytosol invertase, CINV) in fruit of WT and transgenic lines. N, Model of CsSWEET7a function in fruit phloem unloading. Sugar (mostly stachyose and raffinose with a small amount of sucrose) synthesized in source leaves is transported to and unloaded in sink organs, like fruit. Some of the stachyose and raffinose are hydrolyzed to sucrose by α-galactosidase (α-Gal) in the release phloem of the peduncle, gynophore and fruit MVB, and then may be broken down into hexoses by SUS or INV in companion cells. CsSWEET7a, localized in companion cells, exports hexoses to the apoplasmic space. Cell wall acid invertase (CWINV) would most likely break down sucrose into hexose in the cell wall space. The apoplasmic hexoses could be taken up or retrieved into phloem parenchyma cells (PPCs) by some hexose transporters (HTs). This process could be conducted to reduce the hexose level in apoplasmic space and to facilitate sugar unloading. There may exist a sucrose unloading pathway mediated by an unidentified sucrose exporter and importer (purple square). Abbreviation: VB, vascular bundle; MVB, main vascular bundle; Xy, xylem; EP, external phloem; SE, sieve element; CC, companion cell; PPC, phloem parenchyma cell; UDPG, UDP-Glucose. Gene IDs: CsAGA1 (Csa4G631570), CsAGA2 (Csa4G167980), CsSUS4 (Csa5G322500). Mean values ± SE of three independent biological replicates are given. Significance compared to WT was determined by Student’s t test at P < 0.05, **P < 0.01, and ***P < 0.001.
We further tested the transcriptional and translational levels of CsAGAs, CsSUS, and CsINV in the CsSWEET7a transgenic lines (Figure 6, L and M; Supplemental Figure S7). The transcriptional levels of CsAGA1, 2, and CsSUS4 significantly increased in the OE plants but decreased in the RNAi lines (Figure 6L). The genes encoding invertases (CsCINV1, CsCINV2, CWINV3) significantly increased in some OE lines but not all and were also slightly higher in some of the CsSWEET7a-RNAi lines (Supplemental Figure S7). The enzyme activity of AGA increased around 27% in CsSWEET7a-OE lines and reduced by half in RNAi plants (Figure 6M). SUS enzyme activity significantly increased in the CsSWEET7a-OE plants, but significantly decreased in the RNAi lines compared to wild type plants (Figure 6M). The enzyme activity of INV was upregulated in two out of three OE lines and two out of three RNAi lines (Figure 6M). This may be explained by the much higher activity levels of SUS, which indicates that SUS was probably more active in fruit than INV. Overall, we propose that CsSWEET7a may be responsible for hexose efflux from fruit companion cells to stimulate sugar allocation in synergy with several important hydrolases (particularly, AGA and SUS) during phloem unloading (Figure 6N).
Discussion
SWEET sugar transporters have been widely studied in many plant species, including Arabidopsis, rice, and maize. However, the functions of SWEETs remain unknown in the cucumber plant, especially in the fruit, which provides abundant amino acids, vitamins, and trace elements essential to our diet. In this study, we characterized CsSWEET7a and found that it was present in the companion cells (CCs) of the sink organs using RNA-Seq, RT-qPCR, and immunohistochemical localization analyses.
CsSWEET7a functions in fruit phloem companion cells
Increased sink demands generally require increased carbon allocation to sink tissues (Ainsworth and Bush, 2011). In legume seeds, increased sugar flux from the seed coat coincides with a high rate of seed growth (Fader and Koller, 1985). GmSWEET10a and 10b have recently been reported to contribute to this increased transport activity and are progressively expressed at greater levels during seed development (Wang et al., 2020). The continuously increasing size of the carbon pool after anthesis in cucumber (Hu et al., 2009; Yang et al., 2013) indicates enhanced sugar transport into the cucumber fruit. The up-regulation of CsSWEET7a transcripts until 15 DAA in fruit vascular bundle (Figure 1D; Figure 4A) is consistent with the increasing demand of the fruit for photoassimilates. This increase in demand is further supported by detection of the CsSWEET7a protein in the fruit phloem (Figure 1). In our study, the glucose and fructose content, along with the fruit weight and diameter changed in the transgenic cucumber lines (Figure 4; Supplemental Figure S5). Both cell division and expansion contribute to fruit size (Yang et al., 2013; Azzi et al., 2015). Although the differential cell area in transgenic cucumber fruits agree with morphological phenotypes, we cannot exclude cell number (cell division) may also contribute to the fruit phenotype. Rapid cell division in cucumber fruit commenced before anthesis and maintained until 5 DAA, while cell expansion was typically in effect from 5 DAA to 14 DAA (Marcelis and Baan Hofman-Eijer, 1993; Yang et al., 2013). In the present study, the expression level of CsSWEET7a increased sharply after 3 DAA and kept at a high level until 15 DAA (Figure 1D; Figure 4A), indicating that CsSWEET7a might play more important roles during the cell expansion stage of fruit development. A similar fruit phenotype was also reported in tomatoes where a size regulator (CSR-D) gene increases fruit weight by enlarging the pericarp cell size (Mu et al., 2017).
It is worth noting that CsSWEET7a is not only expressed in fruits, but also in other sink tissues including roots and flowers (Figure 1A). Furthermore, compared to the wild type plants, the biomass of the root and flower in CsSWEET7a transgenic lines were altered to some extent (Supplemental Figure S8). This indicates that CsSWEET7a may be involved in phloem unloading in these heterotrophic tissues that depend on sugar supply from source tissues. Therefore, the reduced size of the cucumber fruit in the RNAi mutant lines, or the increased size of the fruit in the overexpression lines, could be due to the pleiotropic effects from roots, flowers, and fruits. It is not surprising to observe that many CsSWEET7a orthologs are also expressed in sink tissues, such as MdSWEET7a and 7b in apples (Zhen et al., 2018), SlSWEET7a in tomatoes (Shammai et al., 2018), VvSWEET7 in grapes (Breia et al., 2020) and SbSWEET4-3 in sorghum (Mizuno et al., 2016).
Consistent with our observation, the expression of SlSWEET1a in the mature fruit of wild and cultivated tomato species is correlated with the fructose-to-glucose ratio, and its expression determines the hexose composition of the ripening tomato fruit (Shammai et al., 2018). With additional expression in the unloading veins of the sink leaves, SlSWEET1a is involved in glucose import into the sink leaves, implicating the role of SlSWEET1a in sugar unloading (Ho et al., 2019). AtSWEET4 is expressed in the vascular tissue and encodes a hexose transporter involved in feeding axial tissues with sugar during plant growth and development (Liu et al., 2016). Here, our immunohistochemical localization provides cell type-specific information showing specific expression of CsSWEET7a in companion cells of vascular tissues (Figure 1, E-K). The companion cell is where phloem loading and unloading take place (Rennie and Turgeon, 2009; Ma et al., 2019a). For species using an apoplasmic unloading pathway, sugars unloading into the apoplasm from the SE/CC complex is a transporter-mediated process. Ren et al. (2020) reported a sugar transporter ClVST1 which was mislocalized from tonoplast to plasma membrane in sweet watermelon to speed up the phloem unloading process. In cucumber, it has been indicated that the phloem-transported stachyose and raffinose were hydrolyzed by α-galactosidase into sucrose in the vascular bundle of the peduncle (Ohkawa et al., 2010), where abundant CsSWEET7a accumulates (Figure 1). However, it is unclear whether sucrose, a sucrose-derived hexose, or both, are exported out of the phloem. The sucrose synthase SUS4, responsible for sucrose metabolism by catalyzing the reversible conversion of sucrose to fructose and UDP-glucose, is expressed in companion cells and phloem parenchyma cells, and its down-regulation results in decreased sugar level and overall reduction in size and weight of flowers and fruits (Fan et al., 2019). In addition, the sucrose-cleaving cell wall invertase is present in the cell wall space of phloem apoplasm (Hu et al., 2011) along with a high level of hexoses and a negligible amount of stachyose and raffinose in the mesocarp (Hu et al., 2009). These observations, along with our findings that the hexose transporting CsSWEET7a is highly expressed and functional in companion cells, support the idea that sucrose metabolism takes place at both CCs and the apoplasm in phloem. We also observed that the mRNA levels and enzyme activities of soluble sugar hydrolases (including alkaline α-Gal and SUS) were in synergy with CsSWEET7a activities in the fruit of transgenic lines (Figure 6), while the invertase activity (including acid INV and alkaline INV) showed more complicated responses to changes in CsSWEET7a levels. A slight upregulation of alkaline INV activity was unexpectedly observed in two out of three RNAi lines (Figure 6), whereas INV activity reacted similarly in the three overexpression lines. This indicates that maintaining hexose to a certain level in fruit may be important, since hexose regulates cell proliferation as a signal (Wang and Ruan, 2013) in addition to providing carbon skeletons. In CsSWEET7a RNAi plants, the increased activity of alkaline INV could compensate for the reduction of SUS activity, which would reduce hexose production, to provide minimal hexose levels demanded by the growing fruits.
It is generally considered that SUS and INV are extensively regulated by sugar availability in plants (Koch, 2004). Recent study has shown that externally supplied glucose will significantly inhibit the expression of SUS and CWINV genes in rice leaves, which is likely through the hexokinase (HXK)-dependent sugar signaling pathway (Li et al., 2020). In cotton, VINV-derived hexose most likely acts as signal to regulate fiber initiation in a dosage-dependent manner through MYB transcription factors and auxin signaling (Wang et al., 2014b). Similarly, CWINV-mediated sugar signaling could probably be perceived or transmitted by hexose transporters, such as SWEET8 and STP9, or receptor-like kinases to modulate downstream MADS-box transcription factors and auxin signaling, and thus plays a pivotal role in Arabidopsis ovule development (Liao et al., 2020). And more notably, SWEET8 and STP9 were spatially co-expressed with two CWINV genes during ovule initiation. In this study, there is a possibility that downstream sugar transporter could affect upstream sugar metabolic enzymes through the feedback regulation mechanisms. When elevated CsSWEET7a in OE transgenic lines was in effect, more hexose will be unloaded from CCs, resulting in less hexose retained in cytosol of CCs, which will likely shift the enzymatic reactions (such as cytosolic AGA1, AGA2 and SUS4) in favor to produce more hexoses. This work is worthy of further discussion in the future.
The presence of both α-Gal and SUS in the phloem is also consistent with the identification of the hexose transporters CsHT2, CsHT3, and CsHT4 in cucumber fruit (Cheng et al., 2015), which may transport the hexose released from the companion cells into other types of cells from the cell wall space. For example, CsHT3 provides the hexose needed for cellulose synthesis during peduncle development by shifting its localization first from the sclereids of young peduncles to the phloem fiber cells and then to the companion cells in the phloem (Cheng et al., 2019). In addition, the proton sucrose transporter CsSUT4 is found primarily expressed in cucumber mesocarp tissues abutting vascular tissues, indicating its role in taking up sucrose from the apoplasm (Hu et al., 2011). One would expect both sucrose and hexose are unloaded apoplasmically from the release phloem in fleshy fruits. However, it remains unclear whether this process is mediated by SUT reversal, SWEETs, and/or unidentified energy coupled transporters (Milne et al., 2018). Clade III SWEETs (SWEET11 to SWEET15) in Arabidopsis, which using sucrose as a substrate, may likely mediate such process in the root of Arabidopsis under drought stress (Durand et al., 2016); however, no histochemical evidence was presented to show the exact cell types. Here we propose that CsSWEET7a, which is highly expressed in peduncle and fruit vascular bundle, exports the hexoses hydrolyzed by AGA, SUS, and INV in companion cells to accelerate phloem unloading in the fruit (Figure 6).
CsSWEET7a participates in the distribution of carbohydrate within or to sink organs
The efficiency of photoassimilate partitioning from source tissue to harvestable tissues is one key determinant of yield potential (Long et al., 2015), which is regulated by source supply, phloem transport, and sink utilization. The efficiency of phloem unloading particularly has a substantial impact on overall plant growth and productivity (Rossi et al., 2015; Lu et al., 2020). 14CO2 source-leaf feeding assays showed that CsSWEET7a overexpression lines delivered more fixed 14C-carbon to sink tissues, while RNAi lines transported less compared to wild type in both young (4-node plants without fruit) and mature (15-node plants with fruit) plants (Figure 5). This control over the allocation of fixed 14C-carbon to fruit by the expression level of CsSWEET7a has consequences on the size and sugar contents in the fruit (Figure 4). This is physiologically beneficial to a plant, as fruit growth is a high priority sink in assimilate partitioning compared to other sink tissues (Wardlaw, 1990). The CsSWEET7a RNAi lines retained the majority of labeled carbon in the stem and petiole, whereas CsSWEET7a overexpression lines transported more carbon to fruits when compared to the wild type (Figure 5). This also supports the idea that CsSWEET7a is responsible for sugar unloading into fruit from phloem. The impaired phloem unloading, resulting in temporary storage of photossimilates in the stem, makes sense considering that cucumber is a vine plant with dozens of nodes that can produce new flowers and fruit further down the stem. We also observed a significant amount of 14C entered fruits in CsSWEET7a-RNAi lines, which could be explained by any remaining CsSWEET7a or the presence of sucrose or other hexose transporters in SE/CC contributing to sugar efflux from the SE/CC. We also cannot exclude contribution from other uncharacterized transporters.
In conclusion, CsSWEET7a is a hexose transporter localized to the plasma membrane in the phloem companion cells of the fruit of cucumber that mediates the efflux of hexose during phloem unloading for fruit development. Genetic manipulation of CsSWEET7a caused altered sugar catabolism, carbon allocation, as well as fruit hexose content and fruit weight and size in transgenic cucumber plants.
Materials and methods
Plant material and bacteria/yeast strains
Cucumber (Cucumis sativus cv Xintaimici) plants were grown in a greenhouse under natural light conditions from February to July or from the end of August to December at China Agricultural University in Beijing. For spatial and temporal expression analysis, different organs, including root, stem, male flower, female flower, and fruit, were sampled from two-month-old plants. For 14C isotope labeling experiments, plants were grown in the growth room under 16-h light (28 °C)/8-h dark (18 °C). The DH5α Escherichia coli strain was used for cloning. Yeast (Saccharomyces cerevisiae) strain EBY.VW4000 was used for heterologous protein expression.
Gene isolation
The sequence of CsSWEET7a (Csa4G054810) was obtained from the Cucurbit Genomics Database (Cucumber Chinese long genome v2 http://cucurbitgenomics.org/organism/2) and used to design primers (Supplemental Table S1). The full-length coding region of the CsSWEET7a gene was amplified from cDNA in male flowers.
Spatial and temporal expression by reverse transcription quantitative PCR
Total RNA was extracted from specified tissues (root, stem, young and mature leaves, male and female flowers, fruit) using RNeasy Plant kit (Huayueyang, Beijing, China) according to the manufacturer’s protocol. The RNA was reverse transcribed using FastQuant RT Kit (with gDNase; Tiangen, Beijing, China). The cDNA samples were used as a template for RT-qPCR analysis. Primers are listed in Supplemental Table S1.
Gene expression analyses were performed using RT-qPCR with the SYBR green detection protocol (TaKaRa, Japan) and the ABI 7500 system (Bio-Rad, United States). All experiments were repeated three times using independently prepared RNA/cDNA templates.
Heterologous expression in yeast
The open reading frame (ORF) of CsSWEET7a was amplified by PCR from cDNA in male flowers and cloned into the S. cerevisiae/E. coli shuttle vector pDR196 (Fan and Zhang, 2009). PCR primers are listed in Supplemental Table S1. The recombinant vector containing CsSWEET7a or the empty pDR196 vector (control) were separately transferred into the hexose uptake-deficient yeast strain EBY.VW4000 as previously described (Cheng et al., 2015). The transformed cells of the hexose uptake-deficient strain were grown on SD (synthetic deficient) medium supplemented with 2% (w/v) maltose and auxotrophic requirements. Serial dilutions (×10, ×102, ×103) of yeast cell suspensions were dropped (2 μL) on solid SD media containing either 2% maltose (w/v control) or 2% (w/v) glucose, fructose, galactose, or mannose plus respective auxotrophic requirements (Cheng et al., 2015). Growth was documented by pictures after 3 d of growth at 30 °C.
For SWEET7a kinetic analysis, 5 glucose concentrations (0, 5, 10, 25, and 50 mM), and 4 time points (10s, 2.5 mins, 5 mins, and 10 mins) for each concentration were used to collect yeast cell samples and measure glucose uptake. [14C]glucose (0.1 μCi D-[U-14C] glucose; 275 mCi/mmol) was used in each sample. The uptake was stopped by iced water. Samples were transferred to a scintillation vial containing 5 ml Ultima Gold XR Scintillation liquid (Perkinelmer). Radioactivity for each vial was measured by a liquid scintillation spectrometry. Substrate specificity was determined by competitive inhibition of [14C]glucose uptake. The sample without competitor was the internal control, and the time interval for [14C]glucose uptake was 6 min. The values are presented as units relative to the values from the internal control taken as 100%.
Immunohistochemical localization
Two specific peptide fragments (TKLQKEEREGKGQVVLS and KNDNIESGNPFAEVHGA) derived from the CsSWEET7a protein sequence were used to immunize two rabbits (specific pathogen-free) by Beijing B&M Biotech. For immunohistochemical analyses, the peduncle and fruit samples were collected at the day of anthesis. All samples were fixed in FAA (formaldehyde – acetic acid – ethanol) solution, followed by series dehydration, and were embedded in wax as described (Wang et al., 2014a). Peduncle and fruit samples were cut into 5-μm thick slices.
For immunohistochemical localization, the slices were blocked in 37 °C for 2 hours with blocking reagent [PBS, pH 7.0, containing 1.5% (w/v) Glycine, 5% (w/v) BSA, 0.1% (v/v) Tween-20]. The tissues were then incubated in the primary antibody at 4 °C overnight before incubating at 37 °C for 1 hour. The slices were washed 3–4 times using 100 mM PBS for 5 min followed by incubation in AP-labeled Goat Anti-Rabbit IgG for traverse slices and in FITC-labeled Goat Anti-Rabbit IgG for longitudinal section of fruit main vascular bundle and peduncle for 2 hours at 37 °C. After immunolabeling, samples were incubated in 0.1% (w/v) aniline blue for 20 min in dark conditions and washed with 100 mM PBS for 3 times. Coverslips were applied using 80% (v/v) glycerin. Slices were imaged with a confocal laser scanning microscope (Zeiss LSM510 META). Argon laser excitation wavelength and emission bandwidth were 488 nm and 500-550 nm for FITC.
GFP fluorescence localization assays
The ORF of CsSWEET7a without stop codons was amplified and cloned into the pCAMBIA super 1300 vector to generate C-terminal fusions with the GFP reporter. Primers are listed in Supplemental Table S1. Transient expression of the CsSWEET7a-GFP fusion proteins in A. thaliana protoplasts, onion epidermal cells, and Nicotiana benthamiana pavement cell were performed according to Ma et al. (2008), Wang et al. (2014a), and Martin et al. (2009). A plasma membrane marker (PM-rk; CD3-1007) was shared by Ma et al. (2008). And a nuclei marker (H2B; Martin et al., 2009) was used to indicate the nuclei position. The empty vector expressing untargeted GFP was used as a control. GFP fluorescence was visualized using an Olympus Confocal Laser Scanning Microscope (FV1000, Japan). Argon laser excitation wavelength and emission bandwidth were 488 nm and 500-550 nm for GFP, 543 nm and 560-620 for mCherry and RFP.
Carbohydrate analyses
Soluble sugars were extracted according to Ma et al. (2019b). Fruit samples were harvested at 2 pm when the carbohydrate transportation rate is high in fruit (Miao et al., 2007). In brief, a 0.5-g fruit sample was homogenized in 5 mL of 80% (v/v) ethanol and incubated at 80°C for 30 min. The samples were centrifuged at 4000 g for 10 min, and the supernatants were collected. This extraction was repeated three times, and the supernatants were combined. The supernatants were evaporated to dryness at 40°C in an oven. The samples were resuspended in 1 mL of deionized water and filtered through a 0.45-µm filter membrane. All these samples were analyzed by HPLC (High-Performance Liquid Chromatography).
Radiolabeling of cucumber leaves with 14CO2 was performed according to Turgeon and Gowan (1992). 15 node-plant with 9 DAA fruit (mature plant) and 4 node-plant without fruit were used for radiolabeling. Shoot apical meristem (a strong sink) was removed before 14CO2 feeding. For mature plant, the leaf which is near to the 9 DAA fruit (at the similar node position in cucumber plant) were chosen as the feed leaf; while, in young plant, the third leaf were chosen as the feed leaf. Attached leaves were sealed in a cuvette and exposed under sunlight for 20 min to 1.0 Mbq 14CO2 generated from NaH14CO3 and excess 80% (v/v) lactic acid. The petioles were wrapped in aluminum foil to prevent inadvertent photosynthetic fixation of 14CO2 by the petiole. After 20 min of exposure to the 14CO2, the leaves were removed from the cuvette and exposed to the normal light for 1 d. Leaves, petioles, stems, flowers, fruits and roots were sampled 28 hours after feeding and dried in oven to constant weight. For young plants with 4 nodes, samples were collected 4 hours after feeding. Dried samples were ground into powder and transferred to a scintillation vial containing 1 ml Ultima Gold XR Scintillation liquid (Perkinelmer) for radioactive analysis. Radioactivity for each vial was measured by a liquid scintillation spectrometry.
Agrobacterium-mediated cucumber transformation
Fragments of CsSWEET7a were amplified with specific primers (Supplemental Table S1). The ORF of CsSWEET7a was cloned into the expression vector pBI121 for overexpression (Supplemental Figure S5A). For RNA interference, silencing constructs were made according to standard methods using pFGC-1008 (Supplemental Figure S5B). These recombined constructs were transformed into cucumber by the Agrobacterium tumefaciens (LBA4404)-mediated method (Sui et al., 2012; Cheng et al., 2015; Li et al., 2017a).
Transgenic lines were screened in selection medium (Li et al., 2017a) and verified by PCR (DNA level) and RT-qPCR (RNA level). T2 transgenic lines (heterozygous or homozygous) were used for functional study.
Membrane protein extraction and western blot
Extraction of membrane proteins was according to Wang et al. (2014a). Briefly, sample (1 g) was homogenized in extraction buffer [50 mM HEPES-NaOH, pH 7.5, 10 mM MgCl2, 1 mM EDTA, 2.5 mM DTT, 0.1% (w/v) BSA, 0.5% (v/v) TritonX-100) and centrifuged at 13,000 x g for 20 min. The supernatant was removed to a new tube and centrifuged at 100,000 x g for 2 h. The precipitate was dissolved with elution buffer (WA-009, Invent). The yeast membrane protein was isolated by Mem-PER™ Eukaryotic Membrane Protein Extraction Kit (Thermo, 89842) according to the protocol. For western blot, samples were separated on SDS-PAGE, blotted onto nitrocellulose, and incubated with anti-CsSWEET7a antibody.
Cell area measurement
For cross- and longitudinal-section observation of fruit mesocarp cells, samples were harvested from the maximal width of cucumber fruit at 15 DAA. Samples were fixed and embedded as previously described (Wang et al., 2014a). Fruit samples were cut into 5-μm thick slices and dewaxed before incubation in 0.02% (w/v) Toluidine Blue in PBS buffer until cells outline were clearly stained. Images were taken under a D72 light microscope (Olympus). Cell area was measured by ImageJ. More than 100 cells from three different regions were measured.
Enzyme extraction and assay
Fruit material (0.6 g) was homogenized in 50 mM HEPES-NaOH extraction buffer (pH 7.5), containing 1 mM ethylenediamine tetraacetic acid (EDTA), 10 mM magnesium chloride (MgCl2·6H2O), 2.5 mM dithiothreitol (DTT), 0.1% (w/v) BSA and 0.05% (v/v) TritonX-100 and centrifuged at 13,000 x g for 20 min at 4 °C. The supernatant was harvested for enzyme activity analysis.
Sucrose synthesis (SUS) activity was determined as described by Lowell et al. (1989), Wang et al. (2014a), and Fan et al. (2019) with some modifications. The 0.7-mL volume reaction contained 80 mM MES (pH 5.5), 5 mM NaF, 100 mM sucrose, 5 mM UDP, and 0.5 mL enzyme extract. Reactions were incubated for 30 min at 30°C and were terminated by boiling with 1 mL 3, 5-dinitro salicylic acid for 10 min. The absorbance was read at 540 nm using a spectrophotometer. A Change in absorbance was considered SUS activity. Reaction medium without UDP was used as the control.
The alkaline invertase activities were assayed following Merlo and Passera (1991) and Wang et al. (2014a) with some modifications. The reaction was carried out for 60 min at 37°C in a reaction mixture containing 0.6 mL of 0.1M acetic acid-sodium acetate solution and 100 mM dipotassium hydrogenphosphate-sodium citrate solution (pH 7.2 for alkaline forms), 100 mM sucrose and 0.2 mL enzyme extract. Reactions were terminated by boiling with 3, 5-dinitro salicylic acid for 5 min. An absorbance change at 540 nm was considered invertase activity. Distilled water was used instead of sucrose in the control reaction.
The α-galactosidase activity was assayed following Wang et al. (2016), with some modifications. The reaction contained 10 mM stachyose, 100 mM HEPES buffer (pH 7.4) and 80 μL enzyme extract and was incubated at 37 °C for 30 min. Then 2 mL Tris–HCl buffer containing 4 mM GSH (glutathione) and 0.4 mM nicotinamide adenine nucleotide (NAD) was added, and the reaction was stopped by boiling at 100°C for 5 min. β-galactose dehydrogenase (0.075 U) was added, and the absorbance at 340 nm was read right after the addition and again after 2 h. The enzyme activity was measured as the amount of galactose formed by calculating the difference between the absorbance OD340 nm at the two times.
Statistical analysis
Statistical analysis was conducted by two-tailed Student’s t test with equal variance using Excel.
Accession numbers
The accession number of all genes used in this paper were obtained from the Cucurbit Genomics Database (Cucumber Chinese long genome v2 http://cucurbitgenomics.org/organism/2) and listed in Supplemental Table S2.
Supplemental data
The following materials are available in the online version of this article.
Supplemental Figure S1. RNAseq expression analysis of 17 CsSWEETs in peduncle, gynophore, fruit vascular tissue, and fruit flesh tissue.
Supplemental Figure S2. Alignment of amino acid sequences of the cucumber SWEET family for antibody development.
Supplemental Figure S3. Specificity analysis of anti-CsSWEET7a antibody.
Supplemental Figure S4. Functional analysis of CsSWEET7a in yeast complementation assay.
Supplemental Figure S5. Phenotype of fruit at 9 DAA in CsSWEET7a transgenic lines.
Supplemental Figure S6. Sugar content in cucumber fruit of wild type at 9 d after anthesis (DAA).
Supplemental Figure S7. Expression level of genes encoding invertase in wild type plant and CsSWEET7a transgenic lines.
Supplemental Figure S8. Phenotype of root and flower in CsSWEET7a transgenic lines.
Supplemental Table S1. Primers used in this study.
Supplemental Table S2. Gene accession numbers used in this study.
Acknowledgements
We thank Prof. Zhenxian Zhang (China Agricultural University) for his constructive suggestions and comments on the project design. This work was supported by the National Key Research and Development Program of China (2019YFD1000300), the National Natural Science Foundation of China (31972398), the Beijing Innovation Consortium of Agriculture Research System (BAIC01), the earmarked fund for China Agriculture Research System (CARS-23), and the 111 Project of Ministry of Education of P.R.C. (B17043).
Conflict of interest statement. The authors declare that they have no conflicts of interest.
X.S. and Y.L. conceived the project and designed the experiments. Y.L., H.L., X.Y., J.W., S.F. and L.S. performed most of the experiments. S.M. and K.X. provided technical assistance to Y.L. Y.L., X.S., H.L., X.Y. and J.W. analyzed the data. Y.L., L-Q. C. and X.S. wrote the article. X.S. agrees to serve as the author responsible for contact and ensures communication.
The author responsible for distribution of materials integral to the findings presented in this article in accordance with the policy described in the Instructions for Authors (https://dbpia.nl.go.kr/plphys/pages/general-instructions) is: Xiaolei Sui ([email protected]).
References
Author notes
Senior author.
Yaxin Li and Huan Liu authors contributed equally to this work.