-
PDF
- Split View
-
Views
-
Cite
Cite
Xiang Li, Mingsen Yu, Pablo Bolaños-Villegas, Jun Zhang, Di'an Ni, Hong Ma, Yingxiang Wang, Fanconi anemia ortholog FANCM regulates meiotic crossover distribution in plants, Plant Physiology, Volume 186, Issue 1, May 2021, Pages 344–360, https://doi.org/10.1093/plphys/kiab061
- Share Icon Share
Abstract
Meiotic recombination increases genetic diversity and manipulation of its frequency and distribution holds great promise in crop breeding. In Arabidopsis thaliana, FANCM (a homolog of mammalian Fanconi anemia complementation group M) suppresses recombination and its function seems conserved in other species including the rosids Brassica spp. and pea (Pisum sativum), and the monocot rice (Oryza sativa). To examine the role of FANCM during meiotic recombination in lettuce (Lactuca sativa, an asterid), we characterized the function of lettuce LsFANCM and found that it can functionally substitute for AtFANCM in transgenic Arabidopsis plants. Moreover, three independent CRISPR/Cas9-edited lettuce Lsfancm mutants showed reduced pollen viability and seed setting. Unexpectedly, analyses of chromosome behavior revealed that 77.8% of Lsfancm meiocytes exhibited univalents. The normal formation of double-strand breaks in DNA and the discontinuous assembly of synaptonemal complex in Lsfancm mutants supports the hypothesis that LsFANCM might be dispensable for the initiation of meiotic recombination but required for normal synapsis. Furthermore, the frequency of lettuce HEI10 (Human Enhancer of Invasion 10) foci, a marker for Class-I crossovers (COs), was similar between wild-type (WT) and Lsfancm. Strikingly, the distribution of LsHEI10 foci and chiasmata in Lsfancm meiotic chromosomes was markedly different from the WT. A similar alteration in the distribution of Class-I COs was also observed in the Arabidopsis Atfancm mutant. Taken together, these results demonstrate that FANCM is important for shaping the distribution of meiotic Class-I COs in plants, and reveal an evolutionarily divergent role for FANCM in meiotic bivalent formation between Arabidopsis and lettuce.
Introduction
In most eukaryotes, sexual reproduction requires meiosis to halve chromosomes to form haploid gametes. The formation of meiotic crossovers (Cos) involves reciprocal exchanges between parental chromosomes, thus leading to genetic diversity among the offspring; this meiotic re-distribution of allelic variation underpins all crop breeding programs (Mercier et al., 2015). In particular, meiotic COs generate new combinations of alleles (haplotypes) along chromosomal regions, allowing desirable traits from different parental lines to be more stably inherited in the progeny. However, COs are relatively rare events in plants and other organisms (Fernandes et al., 2018), occurring at a rate of one to a few per chromosome pair during meiosis, and exhibit a restricted chromosomal distribution with hotspots and cold spots (Wang and Copenhaver, 2018). Therefore, genetic manipulation of CO frequency and distribution holds great promise to promote the efficiency of crop breeding.
Many eukaryotes including flowering plants possess two classes of COs (Higgins et al., 2004, Mercier et al., 2005). Class-I COs are interference-sensitive, meaning that the presence of a CO reduces the chance of formation of additional COs in neighboring chromosomal regions; their formation is dependent on a group of ZMM proteins [Zipper 1 (ZIP1), ZIP2, ZIP3, and ZIP4, Meiotic recombination 3 (Mer3), and MutS homologue 4 (MSH4) and MSH5] and MutL-homolog 1 (MLH1) and MLH3 (Higgins et al., 2004, 2008b; Mercier et al., 2005; Jackson et al., 2006). Class-II COs are interference-insensitive, and their establishment depends on the activity of the structure-specific endonuclease MUS81 (methyl methansulfonate and ultraviolet-sensitive gene clone 81; Higgins et al., 2008a; Berchowitz et al., 2007) or homolog of the Fanconi anemia D2 gene product (Kurzbauer et al., 2018).
Meiotic recombination is initiated by the programmed formation of double-strand breaks (DSBs) in DNA (Lam and Keeney, 2015). In Arabidopsis thaliana, usually 150–250 DSBs are generated and eventually yield about 10 COs, suggesting the existence of factors that limit the number of COs (Mercier et al., 2015). Previous genetic screens in Arabidopsis identified three, functionally distinct CO-suppressing pathways that mainly rely on the respective activities of AtFANCM, AtFIGL1 (Arabidopsis FIDGETIN-LIKE 1), and RecQ helicase AtRECQ4A/B (Crismani et al., 2012; Girard et al., 2015; Seguela-Arnaud et al., 2015). AtRECQ4 A/B limits CO formation through directing recombination intermediates toward the synthesis-dependent strand annealing (SDSA) pathway for repairing DSBs with non-COs (NCOs) as outcomes (Seguela-Arnaud et al., 2015), whereas AtFIGL1 prevents CO formation likely by regulating the single-end invasion step during meiotic recombination (Girard et al., 2015).
Among the three suppressors, AtFANCM was identified first, and its mutation causes a significant increase in meiotic CO frequency (Crismani et al., 2012) and partially restores bivalent formation in the zmm mutant (Crismani et al., 2012; Knoll et al., 2012). Moreover, AtFANCM is required for normal homologous chromosome synapsis, mitotic recombination, and fertility (Knoll et al., 2012). The anti-CO activity of FANCM orthologs was also reported in Brassica spp. (Blary et al., 2018), pea (Pisum sativum; Mieulet et al., 2018), rice (Oryza sativa; Mieulet et al., 2018), fission yeast (Schizosaccharomyces pombe; Lorenz et al., 2012), and Drosophila melanogaster (Kuo et al., 2014), indicating that the role of FANCM in CO suppression may be evolutionarily conserved. Further molecular study in fission yeast showed that FANCM facilitates NCO formation via SDSA pathway (Lorenz et al., 2012), and the accumulation of recombinant intermediates in fancm mutant are presumably resolved by MUS81 to consequently yield Class-II COs (Lorenz et al., 2012, Crismani et al., 2012).
Asterids include approximately 100,000 species, or about one-quarter of all angiosperms (Zhang et al., 2020). Members in the asterids with agricultural and economic importance include tomato (Solanum lycopersicum), potato (Solanum tuberosum), pepper (Capsicum annuum), tobacco (Nicotiana tabacum), celery (Apium graveolens), carrot (Daucus carota subsp. sativus), sweet potato (Ipomoea batatas), coffee (Coffea spp.), tea (Camellia sinensis), and many others. Lettuce (Lactuca sativa L.), an economically important vegetable, is consumed worldwide. It is a diploid (2x = 2n = 18) species of the Compositae family and displays tremendous morphologic variation among different varieties. Although the lettuce genome has been sequenced (Reyes-Chin-Wo et al., 2017), the molecular basis for developmental traits in lettuce remains largely unknown.
Due to the importance of meiotic recombination for crop breeding, this study aimed to manipulate meiotic CO in lettuce by editing a putative FANCM ortholog using Clustered Regularly Interspaced Short Palindromic Repeat (CRISPR)/CRISPR-associated system 9 (Cas9). Unexpectedly, unlike previously reported findings, we found that the lettuce LsFANCM is required for normal bivalent formation during meiosis. Mutations in LsFANCM caused the formation of univalents and the misallocation of chiasmata among chromosomes. We further demonstrated that the average of LsHEI10 foci per nucleus is indistinguishable between wild-type (WT) and Lsfancm but tended to form multi-foci on short chromosome stretches in Lsfancm. Moreover, the misallocation of Class-I CO was also observed in Arabidopsis Atfancm mutant. Taken together, our data reveal a role for FANCM in CO distribution in lettuce and Arabidopsis.
Results
Characterization of LsFANCM
By performing BLAST (Basic Local Alignment Search Tool) against the lettuce genome database using Arabidopsis FANCM protein sequence as a query, a lettuce locus (Lsat_1_v5_gn_8_141780.1) was identified and its predicted protein sequence (named LsFANCM) has 49.9% identity and 62.1% similarity with AtFANCM. Further validation of the sequencing product amplified by PCR showed that the LsFANCM genomic locus is 7,971 bp in length (from start to stop of transcription), comprising 21 exons and 20 introns, which encodes a protein with 1,254 amino acids. LsFANCM contains an N-terminal helicase domain consisting of DEXDc and HELICc segments (Supplemental Figure S1), which are conserved across plants, animals, and fungi (Meetei et al., 2005; Crismani et al., 2012; Lorenz et al., 2012; Kuo et al., 2014). We then constructed a phylogenic tree using FANCM protein sequences selected from representative plant species (Supplemental Figure S2). The topology of the FANCM gene tree mirrored the corresponding species tree, and most species on the tree showed a single copy of FANCM, suggesting that its function may be conserved and retained during plant evolution.
A previous study showed that AtFANCM is widely expressed in the flower and shoot apical meristems (Knoll et al., 2012). To examine the expression pattern of LsFANCM in lettuce, we sampled the roots, stems, leaves, and ray florets. As a Compositae species, lettuce forms a typical capitulum each with 20–30 ray florets, and meiocytes are generated in capitula about 1.5–2 mm in length. Reverse transcription quantitative PCR (RT-qPCR) analyses showed that LsFANCM is expressed ubiquitously in all tissues examined and appears to be highly expressed in florets at the meiotic stage (Figure 1A).
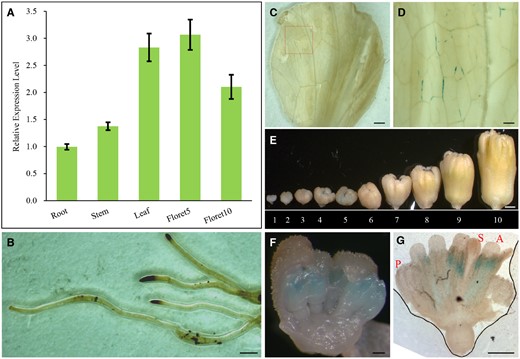
Expression analysis of LsFANCM. A, Expression level of LsFANCM was examined in root, stem, leaf, floret 5, and floret 10 by RT-qPCR. Florets 5 and 10 were collected from capitulum 5 and 10, respectively. Error bars indicate SD, n=3. B–G, Histochemical analysis of GUS staining of ProLsFANCM::GUS transgenic lettuce. B, Roots. C, Leaves. D, The enlargement of the red box in (C). E, Capitula 1–10 at different developmental stages in length= 0.6, 1 , 1.3, 1.6, 1.9, 2.2, 2.6, 3.5, 4.8, and 7.9 mm, respectively. F, The enlargement of anatomical capitulum 5. G, A floret from capitulum 5. S, stigma; A, anther; P, petal. Bars = 1 mm (B, C, and E), 200 µm (D, F, and G).
The expression pattern was further validated by introducing the native LsFANCM promoter fused with a β-glucuronidase (GUS) reporter gene (defined as ProLsFANCM::GUS) into lettuce plants for the subsequent histochemical GUS-staining assay (Figure 1, B–G). The corresponding ProLsFANCM::GUS signals were detected in vascular tissues of leaves (Figure 1, C and D) and in anthers at meiotic stage (Figure 1, E–G), which is generally in agreement with the RT-qPCR data.
LsFANCM suppresses CO formation in Arabidopsis Atfancm Atmsh4 double mutant
To further explore whether LsFANCM has a similar role in limiting meiotic COs, we conducted a complementation test of LsFANCM in the Arabidopsis Atfancm-9 Atmsh4 double mutant. The Atfancm-9 mutant overcomes the phenotype of reduced CO and infertility in zmm mutants (Crismani et al., 2012). Therefore, the rationale for conducting the complementation assay is that if LsFANCM and AtFANCM are functionally similar, the transformants carrying FLAG-tagged LsFANCM driven by the AtFANCM promoter in the Atfancm-9 Atmsh4 double-mutant background would show a phenotype similar to that of the Atmsh4 single mutant.
In total, we obtained 20 independent, confirmed ProAtFANCM::LsFANCM transgenic plants in the Atfancm-9/Atmsh4 homozygous background and selected two lines, namely ProAtFANCM::LsFANCM/Atfancm-9 Atmsh4-1 and ProAtFANCM::LsFANCM/Atfancm-9 Atmsh4-2, for subsequent characterization. The two representative lines were validated by PCR (Supplemental Figure S3A) and Western blotting using the anti-FLAG antibody (Supplemental Figure S3B). Compared with the Atfancm-9 Atmsh4 double mutant, ProAtFANCM::LsFANCM/Atfancm-9 Atmsh4-1 and ProAtFANCM::LsFANCM/Atfancm-9 Atmsh4-2 exhibited many inviable pollen grains and short siliques as in the Atmsh4 single mutant (Figure 2, A and B). Furthermore, we observed chromosome behavior of male meiocytes and estimated the frequency of chiasmata based on the conformation of metaphase I bivalents (Moran et al., 2001). In WT Arabidopsis, we observed 5 ± 0.00 (n = 30) bivalents and 9.52 ± 0.81 (n = 30) chiasmata, whereas only 1.15 ± 0.61 (n = 33) bivalents and 1.25 ± 0.79 (n = 33) chiasmata were found in Atmsh4 (Figure 2, C and D). In contrast, Atfancm-9 Atmsh4 restored the number of bivalents and chiasmata to 4.42 ± 0.71 (n = 31) and 6.71 ± 1.51 (n = 31), respectively (Figure 2, C and D). Compared with Atfancm-9 Atmsh4, ProAtFANCM::LsFANCM/Atfancm-9 Atmsh4-1 formed 1.41 ± 0.77 (n = 22) bivalents and 1.50 ± 0.78 (n = 22) chiasmata, and ProAtFANCM::LsFANCM/Atfancm-9 Atmsh4-2 formed 1.73 ± 0.81 (n = 26) bivalents and 1.77 ± 0.93 (n = 26) chiasmata (Figure 2, C and D; P < 0.01, two-tailed Mann–Whitney test), suggesting that LsFANCM possesses an anti-CO role similar to that of AtFANCM, possibly by promoting SDSA activity.
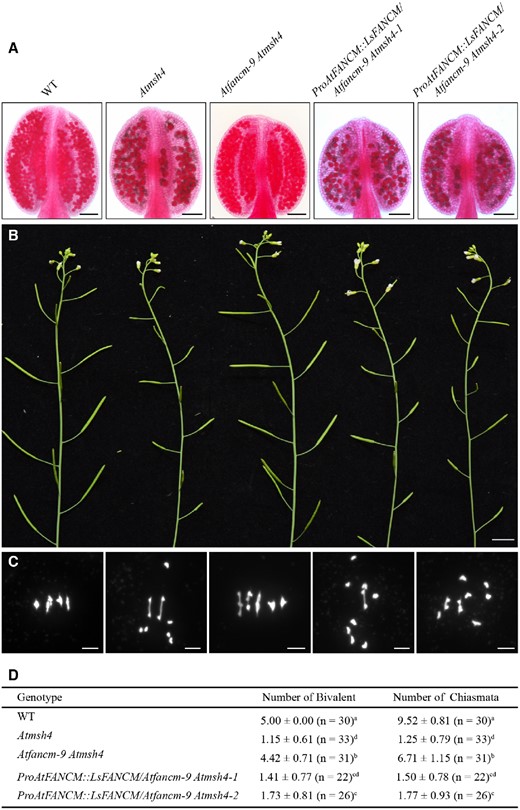
Assay of LsFANCM in the Atfancm-9 Atmsh4 double mutant. A–C, Phenotypic analysis of WT, Atmsh4, Atfancm-9 Atmsh4, ProAtFANCM::LsFANCM/Atfancm-9 Atmsh4-1, and ProAtFANCM::LsFANCM/Atfancm-9 Atmsh4-2. A, Anthers stained with Alexander Red. Images were digitally abstracted for comparison. B, Main apical shoots with mature siliques. C, Chromosome behaviors at metaphase I. D, Statistics of the number of bivalents and chiasmata in WT, Atmsh4, Atfancm-9 Atmsh4, ProAtFANCM::LsFANCM/Atfancm-9 Atmsh4-1, and ProAtFANCM::LsFANCM/Atfancm-9 Atmsh4-2. In the same column, the means followed by different letters (a, b, c, or d) are significantly different from each other (P <0.01, two-tailed Mann–Whitney test). Bars = 100 µm (A), 1 cm (B), 5 µm (C).
Generation of Lsfancm mutants by CRISPR/Cas9
CRISPR/Cas9, as a powerful tool for targeted gene editing, has been successfully applied in many crop species (Chen et al., 2019; Mao et al., 2019). To obtain lettuce Lsfancm mutants, we used CRISPR/Cas9 to knockout the putative LsFANCM gene. Three target sites on different LsFANCM exons were designed (Figure 3A). In total, 34% (n = 73) of all T0 LsFANCM transgenic plants were effectively edited and carried heterozygous mutation at designed sites (Figure 3B). The edited plants carrying the 26-bp deletion at target 1, the 2-bp deletion at target 2, and the 1-bp insertion at target 3 (hereinafter referred to as Lsfancm-1, Lsfancm-2, and Lsfancm-3, respectively) were retained for subsequent analysis. Owing to the premature termination of translation, Lsfancm-1, Lsfancm-2, and Lsfancm-3 possibly produce truncated LsFANCM proteins 229, 421, and 737 aa in length, respectively, compared to the WT full-length of 1,254 aa (Figure 3, C–E). T1 seeds were harvested from self-pollinated T0 lines. Among the T1 populations, about one quarter of all lines corresponding to target 1 (12/50), target 2 (13/50), and target 3 (13/50) were homozygous for the Lsfancm mutation and the mutation forms were maintained in the T1 plants (Figure 3, C–E), providing evidence that the mutations mediated by CRISPR/Cas9 in lettuce are stably inherited through the germline cells.
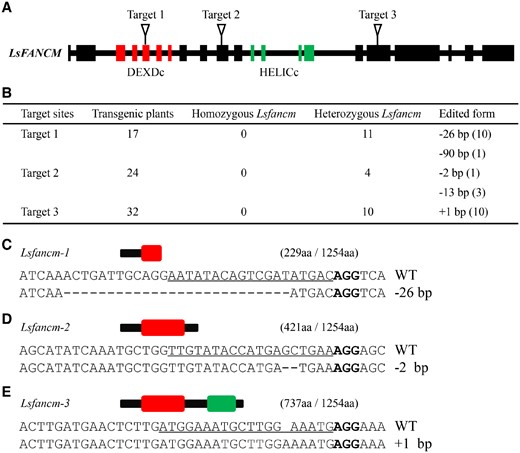
Sequence features of the Lsfancm mutants edited by CRISPR/Cas9. A, Schematic positions of the designed three target sites in LsFANCM. The red rectangles indicate DEXDc-coding region and the green rectangles indicate HELICc-coding region. B, Summary of the edited sites and efficiency in T0 transformants. C–E, The resulting alleles after editing with CRISPR/Cas9 and the schematics of the predicted truncated proteins. The red rectangle indicates DEXDc domain and the green rectangle indicates HELICc domain of LsFANCM protein. The numbers in parentheses represent the amino acid number of the truncated and WT LsFANCM. The PAM sequence is highlighted in bold and the target sequence is indicated by underlines.
It has been reported that in eukaryotic cells, the mRNA containing premature termination codons is rapidly degraded by the nonsense mRNA decay pathway (Conti and Izaurralde, 2005). Thus, we conducted RT-qPCR to compare the abundance of mRNA from LsFANCM between WT and Lsfancm mutants. Consistently, the mRNA level of LsFANCM was much lower in Lsfancm mutants than that of WT (Supplemental Figure S4), indicating that aberrant mRNAs with premature termination codon in Lsfancm might be degraded faster than that of WT one. A similar situation was previously reported in rice (Zhang et al., 2017) and soybean (Glycine max; Cai et al., 2018).
The three Lsfancm mutant alleles show obviously reduced fertility
Arabidopsis Atfancm mutant exhibits reduced fertility compared with WT (Knoll et al., 2012) and is synthetic lethal in combination with AtMUS81 mutation (Crismani et al., 2012; Dangel et al., 2014). We then investigated the phenotypes of WT lettuce and Lsfancm homozygous mutants. Both the WT and the Lsfancm homozygous mutants were segregated from Lsfancm heterozygous mutant, creating lines thought to have the same genetic contents except for the Lsfancm mutation. Compared with the WT lettuce, Lsfancm mutants had similar vegetative growth, capitulum organization, and floral organ identity (Figure 4, A–D; Supplemental Figure S5, A–C). However, pollen viability as assayed by Alexander Red staining showed that 71 ± 7.7% of pollen grains (n = 539 from 21 florets of 3 three plants) in Lsfancm-1 were viable (Figure 4, E–G). In contrast, 98 ± 2.4% of WT pollen grains (n = 457 from 21 florets of three plants) were viable (P < 0.01, two-tailed Mann–Whitney test; Figure 4G). Moreover, 79 ± 10% of WT seeds (n = 731 from 45 capitula of three plants) were normal, whereas in Lsfancm-1 only 40 ± 15% (n = 600 from 45 capitula of three plants) of seeds looked like normal (Figure 4, H–I, P < 0.01, two-tailed Mann–Whitney test). Similar fraction of viable pollen grains were also observed in Lsfancm-2 (67 ± 7.3%, n = 428 from 17 florets of three plants) and Lsfancm-3 (75 ± 5%, n = 405 from 16 florets of three plants; Supplemental Figure S5, D–F). Taken together, these results strongly support the idea that LsFANCM is required for normal fertility in lettuce.
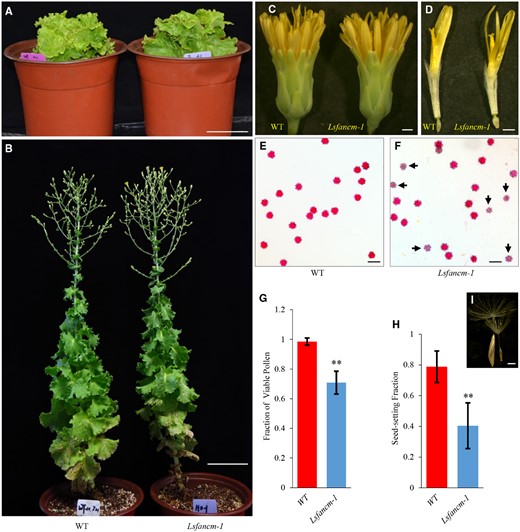
Phenotypic characterization of WT lettuce and Lsfancm-1mutant. A, Seedlings of WT and Lsfancm-1 before bolting. B, Mature plants of WT and Lsfancm-1 at flowering. C, Capitula of WT and Lsfancm-1. D, Florets of WT and Lsfancm-1. E and F, Alexander-red staining of pollen grains from WT and Lsfancm-1. The arrowheads indicate the aborted pollen grains, which are light-colored and smaller in size than the viable ones. G and H, Comparison of the fractions of viable pollen grains and seed set in WT and Lsfancm-1, respectively. Error bars indicate SD. n =21 for WT and Lsfancm-1 (G), n =45 for WT and Lsfancm-1 (H).**P ≤ 0.01. Two-tailed Mann–Whitney test. I, Example showing normal seed (left) versus aborted seed (right). Bars =10 cm (A and B), 1 mm (C and D), 50 µm (E and F), 1 mm (I).
LsFANCM is required for the formation of bivalents
To determine the processes leading to reduced fertility in Lsfancm, we analyzed the behavior of meiotic chromosomes in spreads stained with 4,6-diamidino-2-phenylindole (DAPI), in WT and Lsfancm male meiocytes. We found that WT lettuce showed similar chromosome morphologies to what has been reported in other plant species (Figure 5). At leptotene, chromosomes are condensed and form the axial element, which resembles a thin thread-like structure (Figure 5A). At zygotene, homologs begin to pair, and align with each other (Figure 5B). At pachytene, fully synapsed homologs appear as thick thread-like chromosomes (Figure 5C). At diplotene, disassembly of the synaptonemal complex (SC) leads to separation of homologs except at regions where chiasmata (a physical CO seen by microscopy) occur (Figure 5D). At diakinesis, further chromosome condensation leads to the formation of nine bivalents (Figure 5I). At metaphase I, the highly condensed bivalents align on the equatorial plate as they are pulled by spindle (Figure 5J). Then at anaphase I, the removal of sister chromatid cohesion on chromosome arms allows the separation of homologs (Figure 5K), thus leading to the formation of dyads at prophase II (Figure 5L).
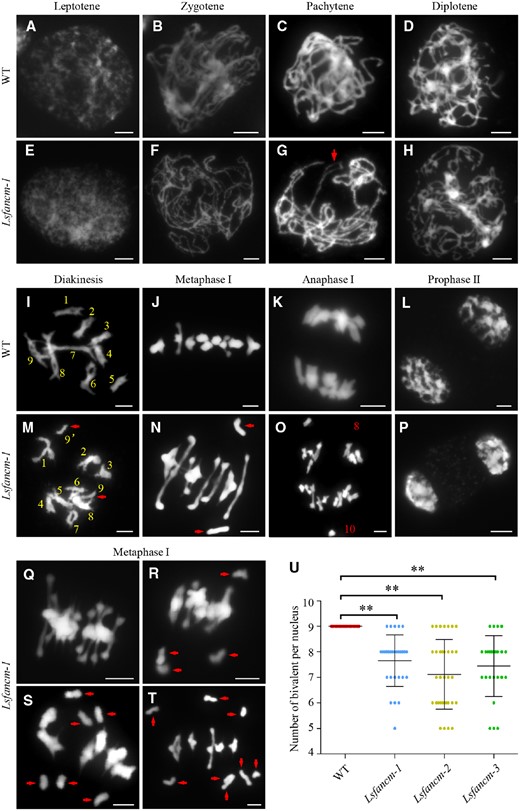
Meiotic chromosomes of WT and Lsfancm-1 mutant. A–D, WT meiotic chromosome behaviors at leptotene, zygotene, pachytene, and diplotene, respectively. E–H, Lsfancm-1 meiotic chromosome behavior at leptotene, zygotene, pachytene, and diplotene, respectively. Arrowhead in (G) indicates the bubble structure of pachytene chromosomes. I, J, WT nucleus forms nine bivalents at diakinesis and metaphase I. M, N, A pair of univalents (pointed by arrowheads) are observed in Lsfancm-1 at diakinesis and metaphase I. K, Nine pairs of homologs are separated equally toward two poles at anaphase I in WT. O, Unequal segregation of homologs with 8 and 10 chromosomes at different sides at anaphase I in Lsfancm-1. WT (L) and Lsfancm-1(P) chromosome behaviors at prophase II. Q–T, The meiocytes of Lsfancm-1 at metaphase I show none, two pairs, three pairs, and four pairs of univalents, respectively. Univalents are marked by arrowheads. Bars = 5 µm. U, Statistical analysis of the number of bivalents at metaphase I in WT and Lsfancm mutants. **P ≤ 0.01. Two-tailed Wilcoxon signed rank test.
In Lsfancm-1, chromosome behaviors were indistinguishable from the WT at both leptotene and zygotene (Figure 5, E and F). However, about 10% Lsfancm-1 pachytene chromosomes showed bubble or bifurcation structures (n = 58 for Lsfancm-1 and n = 80 for WT; Figure 5G), indicating incomplete synapsis. Relative to WT (n = 84) with nine bivalents at diakinesis, univalents were observed in 62% Lsfancm-1 diakinesis cells (Figure 5M; n = 68), indicating a potential loss of obligate COs. The presence of univalents was further observed in 77.8% of Lsfancm-1 metaphase I cells (n = 45 for Lsfancm-1 and n = 39 for WT; Figures 5, N, Q–T) with two univalents (n = 20; Figure 5N), four univalents (n = 12; Figure 5R), six univalents (n = 3; Figure 5S), and eight univalents (n = 1; Figure 5T). Consistently, meiocytes from the Lsfancm-2 and Lsfancm-3 mutants frequently exhibited univalents during metaphase I (Supplemental Figures S5, G–I). Due to the unbalanced distribution of univalents, unequal segregation of homologs was observed at 10% anaphase I cells in Lsfancm-1 (Figure 5O; n = 50 for Lsfancm-1 and n = 18 for WT).
We then counted the number of bivalents in WT and Lsfancm. As shown in Figure 5U, the number of bivalents in Lsfancm-1, -2, and -3 meiocytes was 7.66 ± 0.99 (n = 29), 7.12 ± 1.35 (n = 34), and 7.44 ± 1.17 (n = 25), respectively, which indicates a significant reduction when compared to a frequency of 9.00 ± 0.00 bivalents in WT (n = 30, P < 0.01, two-tailed Wilcoxon signed rank test), and provides strong evidence that LsFANCM is required for the formation of bivalents in lettuce.
LsFANCM is dispensable for meiotic DSB formation but required for normal synapsis
Meiotic recombination is initiated with the programmed formation of DSBs by the SPO11 topoisomerase (Keeney et al., 1997), which seems to be conserved in eukaryotes. In order to corroborate defects in DSB formation in Lsfancm, we examined the relative frequency of a conserved DSB marker, the phosphorylated histone H2AX (γ-H2AX; Lowndes and Toh, 2005), which has been extensively used previously (Su et al., 2017). We found that WT meiocytes at leptotene show on average 1,716 ± 90 (n = 10; Figure 6, A, E) γ-H2AX foci, which is more than the number reported for either rice or Arabidopsis (He et al., 2016, Xue et al., 2018), suggesting that plant species such as lettuce with a large genome size may generate more DSBs. At pachytene, with the gradual repair of DSBs, γ-H2AX foci were reduced to 783 ± 100 (n = 10) in WT (Figure 6, C and E). We did not observe a significant difference in the number of γ-H2AX foci between WT and Lsfancm-1 at either leptotene (1,761 ± 206, n = 10, P = 0.91, two-tailed Mann–Whitney test; Figure 6, B and E) or pachytene (766 ± 35, n = 10, P = 0.76, two-tailed Mann–Whitney test; Figures 6, D and E), providing evidence that LsFANCM is dispensable for the formation of DSBs.
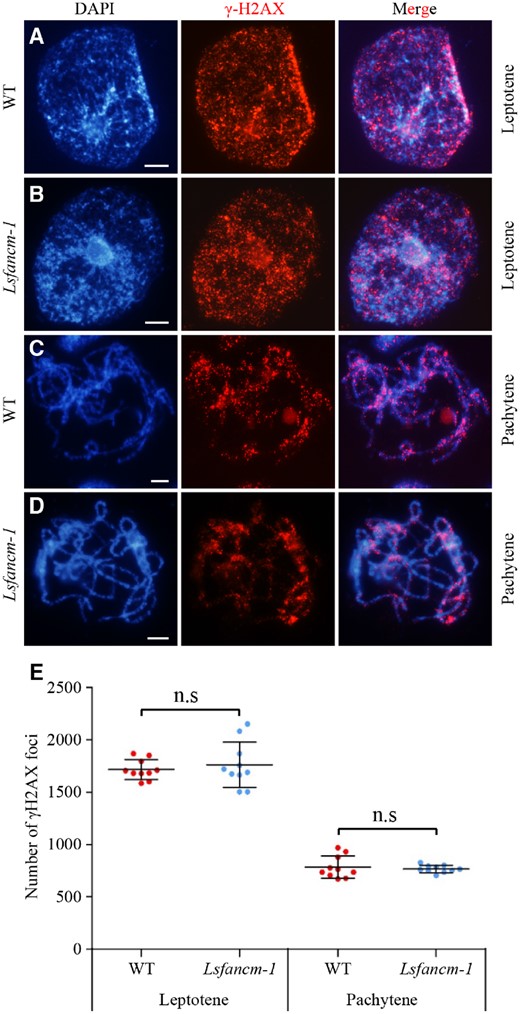
Immunostaining of γ-H2AX in WT and Lsfancm-1. The distribution of γ-H2AX foci are shown at leptotene (A and B) and pachytene (C and D). DAPI signals are shown in blue and γ-H2AX foci are shown in red. E, Statistics of the number of γ-H2AX foci at leptotene and pachytene in WT and Lsfancm-1. n.s, not significant, two-tailed Mann–Whitney test. Bars = 5 µm.
Observation of chromosome bubbles or bifurcated chromosomes at pachytene in Lsfancm indicates a potential defect in synapsis (Figure 5G). To further test this hypothesis, we generated a lettuce LsZYP1 antibody that targets the central element of the SC (Higgins et al., 2005), and immunostaining using this antibody shows specific signals in meiocytes, but not in adjacent somatic cells (Supplemental Figure S6A). In WT, LsZYP1 signals began to appear at leptotene on chromosomes (Supplemental Figures S7, A, C), and showed complete colocalization with the synapsed homologs at pachytene (Figure 7, A and B). No obvious differences of patterns for LsZYP1 signals during leptotene and zygotene were observed between WT and Lsfancm-1 (Supplemental Figure S7, B and D). However, unlike WT pachytene chromosomes (n = 42) with a linear distribution of LsZYP1, 9% Lsfancm-1 chromosomes (n = 54) exhibited discontinuous LsZYP1 signals at pachytene (Figure 7, C and D), further supporting that incomplete synapsis occurs in Lsfancm-1, which is similar to previous finding of an Arabidopsis Atfancm allele (Knoll et al., 2012).

Immunostaining of LsZYP1 during pachytene in WT and Lsfancm-1. LsZYP1 was detected at pachytene in WT lettuce (A and B) and Lsfancm-1 (C and D). B and D are the enlargement of the boxed in (A) and (C), respectively. The arrowheads in (D) indicate the discontinuous LsZYP1 signals at pachytene in Lsfancm-1. DAPI signals are shown in blue and LsZYP1 signals are shown in red. Bars = 5 µm.
Both LsFANCM and AtFANCM are required for the proper distribution of COs
The formation of univalents in Lsfancm mutants suggests the reduction or the misallocation of meiotic COs. To distinguish these possibilities, we first estimated the frequency and distribution of chiasmata in WT lettuce and Lsfancm based on the morphology of metaphase I bivalents. Usually, the presence of a rod bivalent indicates the formation of chiasma(ta) on one chromosome arm only, whereas a ring bivalent has chiasmata relatively distally on both arms (Moran et al., 2001; Supplemental Figure S8). However, this method has relatively low-resolution power for distinguishing multiple COs located on the same chromosome arm, possibly leading to an underestimation of actual number of COs. In WT lettuce, the average number of chiasmata per chromosome pair was 2.06 ± 0.57 (n = 270). In contrast, the corresponding values were 1.46 ± 0.86 (n = 261) for Lsfancm-1 and 1.41 ± 0.91 (n = 306) for Lsfancm-2.
Furthermore, we found different distribution patterns of chiasmata frequency among chromosomes between WT and Lsfancm mutants (Figure 8A; WT vs Lsfancm-1, P = 4.74E-11; WT vs Lsfancm-2, P = 4.11E-11, chi-square test). WT formed at least one chiasma per chromosome pair and 69% of bivalents possessed two recognized chiasmata. In contrast, 18% of Lsfancm chromosome pairs (15% for Lsfancm-1 and 21% for Lsfancm-2) had no chiasmata on average due to formed univalents (Figure 8A). Although the chromosome pairs with two chiasmata were also most frequent in Lsfancm-1 and Lsfancm-2, the percentage decreased to about 43% relative to the 69% of WT (Figure 8A). We also found that chiasmata distribution in Lsfancm deviated significantly from a Poisson distribution (Lsfancm-1 versus Poisson, P = 3.39E−5; Lsfancm-2 versus Poisson, P = 6.68E−6, chi-square test), suggesting that CO interference is still partially functional in Lsfancm and that some of the remaining COs are interference-sensitive.
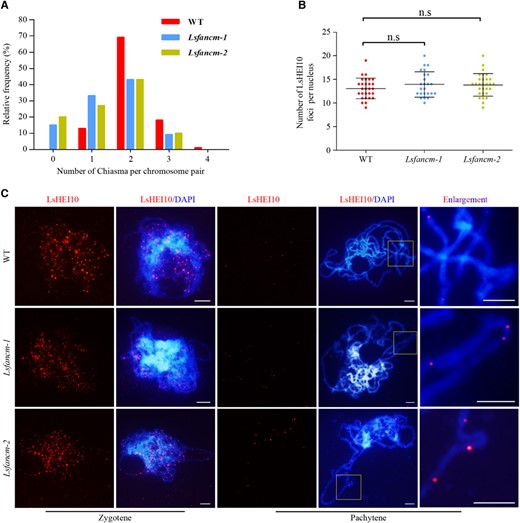
Estimation of CO distribution in WT lettuce and Lsfancm. A, Distribution of chiasmata per chromosome pair during metaphase I. The chiasmata distribution in WT deviates significantly from Lsfancm-1 and Lsfancm-2. WT vs Lsfancm-1, P = 4.74E-11; WT vs Lsfancm-2, P = 4.11E-11, Chi-square test. B, Statistical analysis of the number of LsHEI10 foci at pachytene in WT and Lsfancm-1 and Lsfancm-2. n.s, not significant, two-tailed Mann–Whitney test. C, Immunostaining of LsHEI10 in WT, Lsfancm-1, and Lsfancm-2 at zygotene and pachytene. DAPI signals are shown in blue and LsHEI10 foci are shown in red. Bars = 5 µm.
To further investigate the formation of Class-I CO in Lsfancm, we generated a rabbit polyclonal antibody against the lettuce LsHEI10, a protein marker of Class-I COs (Chelysheva et al., 2012; Wang et al., 2012), and examined the number and distribution of LsHEI10 signals in lettuce. The signals of anti-LsHEI10 antibody were specific in meiocytes and absent in adjacent somatic cells (Supplemental Figure S6B). We did not observe an obvious difference in the average total number of LsHEI10 foci between WT and Lsfancm at both zygotene (WT, 220.47 ± 23.01, n = 19; Lsfancm-1, 226.67 ± 22.38, n = 18, P = 0.35, two-tailed Mann–Whitney test) and pachytene (WT, 13.07 ± 2.19, n = 28; Lsfancm-1, 13.92 ± 2.67, n = 24, P = 0.35, two-tailed Mann–Whitney test; Figure 8B). Nevertheless, we found that ∼5% of meiocytes (3/62 for Lsfancm-1, 3/47 for Lsfancm-2) showed three LsHEI10 foci on a short chromosome stretch (Figure 8C), whereas this phenotype was not observed in the WT (n = 81). These results suggest that the distribution pattern of Class-I CO was altered in Lsfancm.
Changes in the distribution patterns of chiasmata and LsHEI10 foci among chromosomes in Lsfancm suggest that LsFANCM is required for the appropriate distribution of Class-I COs. To test whether the role of FANCM in shaping Class-I CO distribution is conserved in other species, we examined the number and distribution of AtHEI10 foci in chromosomes of Arabidopsis during meiotic diakinesis using the anti-AtHEI10 antibody. The antibody showed punctate signals in the WT meiocytes, which were absent in both WT somatic cells and Athei10-2 meiocytes (Supplemental Figure S6C). As shown in Figure 9, no significant difference in the number of AtHEI10 foci per nucleus was observed between WT (9.68 ± 1.07, n = 28) and Atfancm-9 (9.56 ± 1.79, n = 25; P = 0.44, two-tailed Mann–Whitney test). However, Atfancm-9 chromosomes showed a different distribution pattern of AtHEI10 foci compared with WT Arabidopsis (P = 2.66E−4, Chi-square test; Figure 9D). In WT, every bivalent formed at least one AtHEI10 focus and 66% (n = 140) of bivalents had two AtHEI10 foci (Figure 9, A and D). In contrast, in meiociyes of Atfancm-9, about 7% (n = 125) of all bivalents did not show any AtHEI10 foci, suggesting a lack of Class-I COs, whereas the percentage of bivalents with only two AtHEI10 foci decreased to 46% (Figure 9, B and D). In addition, the proportion of bivalents with at least three AtHEI10 foci was higher in Atfancm-9 than that in WT (Figure 9D). Furthermore, a difference in chiasmata distribution in chromosomes was also observed between Arabidopsis WT and Atfancm-9 (P = 2.16E−7, Chi-square test; Figure 9E). Together, these results support the idea that FANCM has a conserved role in plants in mediating the distribution of Class-I COs.
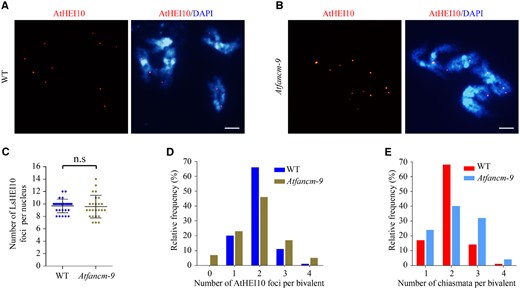
Estimation of type-I CO distribution in WT and Atfancm-9. A, B, Immunostaining of AtHEI10 in WT and Atfancm-9 at diakinesis. DAPI signals are shown in blue and AtHEI10 foci are shown in red. Bars = 5 µm. C, Statistical analysis of the number of AtHEI10 foci per nucleus at diakinesis in WT and Atfancm-9. n.s, not significant, two-tailed Mann–Whitney test. D, Distribution of bivalent by counting number of AtHEI10 foci at diakinesis. In Atfancm-9 mutant, the distribution of AtHEI10 foci deviates significantly from the WT. WT vs Atfancm-9, P = 2.66E−4, Chi-square test. E, Count of chiasmata frequency per bivalent. The distribution patterns are significantly different between WT and Atfancm-9. WT vs Atfancm-9, P = 2.16E−7, Chi-square test.
Discussion
LsFANCM and AtFANCM are required for the proper distribution of meiotic COs
In this study, we demonstrate that both lettuce LsFANCM and Arabidopsis AtFANCM are required for a proper distribution of COs during meiosis, whereas LsFANCM has an additional role in the formation of meiotic bivalents. Thus, we hypothesize that the reduced fertility in Lsfancm could be the result of a defect in meiotic recombination that causes univalents, which in turn leads to unbalanced segregation of homologs during meiosis. The reduced fertility in Lsfancm is consistent with a previous report on an allelic series of Arabidopsis Atfancm mutants (Knoll et al., 2012). However, Atfancm does not form univalents (Knoll et al., 2012), thus it seems that the reduced fertility in Atfancm and Lsfancm is caused by the activity of FANCM-associated factors. We show that Lsfancm displays a frequency of LsHEI10 foci similar to that of the WT, but in Lsfancm, LsHEI10 tend to form clusters of multiple-foci on pachytene chromosomes. Furthermore, the distribution pattern of chiasmata per chromosome pair in Lsfancm was different from that in WT (Figure 8). These results support the idea that the univalents formed in Lsfancm are probably the result of misdistribution of Class-I COs, instead of being caused by a reduction in CO frequency per se. Moreover, we demonstrate that Arabidopsis AtFANCM performs a similar function during the control of distribution of Class-I COs (Figure 9). Therefore, in addition to the previously reported function of promoting NCO generation within the SDSA pathway, our results indicate that FANCM is also required for guaranteeing the proper distribution of Class-I COs.
The molecular mechanism of how FANCM regulates Class-I CO distribution and how it coordinates the SDSA pathway need further investigation. Although 7% of bivalents in Atfancm-9 lack Class-I COs, univalents were not found because of an increase in the frequency of Class-II COs (Figure 2; Crismani et al., 2012). However, univalents form frequently in Lsfancm, suggesting that Class-II CO formation is not increased to maintain the levels of obligate COs in the absence of normal Class-I CO distribution. Therefore, the improper CO distribution in fancm chromosomes could be independent from an increase in the frequency of Class-II COs. One possible mechanism for FANCM in the control of Class-I CO distribution might be that either FANCM or its two partner proteins MHF1 (Mph1-associated histone fold-containing protein1) and MHF2 (Girard et al., 2014) interact with components of the ZMM pathway, thereby determining the position of COs along chromosomes.
AtAXR1 (Arabidopsis AUXIN RESISTANT1) and AtPSS1 (Arabidopsis Pollen Semi-Sterility1) have been reported to control the distribution of Class-I COs along chromosomes, but may not determine the frequency of Class-I COs in Arabidopsis (Duroc et al., 2014; Tagliaro-Jahns et al., 2014). Another protein called AtMCC1 (Arabidopsis MEIOTIC CONTROL OF CROSSOVERS1) was shown to have an opposite role in regulating CO distribution (Perrella et al., 2010). Overexpression of AtMCC1, a GCN5-related histone N-acetyltransferase coding gene, results in a shift of chiasmata distribution along chromosomes and formation of univalents, possibly through affecting chromatin structure (Perrella et al., 2010). In contrast, AtAXR1, an E1 enzyme of the Neddylation complex, activates a Cullin 4 RING ligase and regulates CO localization (Tagliaro-Jahns et al., 2014) independently of its role in affecting DNA methylation (Christophorou et al., 2020). It is thought that the motion of the Arabidopsis kinesin AtPSS1 along microtubules may generate cytoplasmic forces that are transduced via a SUN-WIP (Sad1/UNC-84-WPP domain–interacting protein) module and help coordinate synapsis and CO distribution (Duroc et al., 2014). Those results suggest that a normal distribution of COs is influenced by different pathways at different levels. The main consequences arising from the misdistribution of COs are: (1) the loss of formation of obligate COs, referring to the requirement that at least one CO per homologs pair is formed (Jones, 1984) and (2) the reduction in the ratio of CO interference. Particularly, Ataxr1 showed negative interference, which means that the presence of one CO may increase the probability of another CO being formed nearby (Tagliaro-Jahns et al., 2014), revealing a strong correlation between CO obligation and interference. This notion was supported by a modeling analysis that concluded the formation of obligate COs and CO interference are the result of the same process (Zhang et al., 2014). However, more experimental evidence is needed to clarify the relationship between CO interference and the formation of obligate COs in plants.
The potential mechanisms underlying the different meiotic phenotypes between Lsfancm and Atfancm
Although Lsfancm showed different meiotic chromosome phenotypes compared to Atfancm, several pieces of evidence support the notion that the meiotic functions shown by LsFANCM and AtFANCM could be evolutionarily conserved to a certain extent. First, the anti-CO activity of FANCM orthologs is likely to be evolutionarily conserved and has been reported in plants (Crismani et al., 2012; Blary et al., 2018; Mieulet et al., 2018), animals (Kuo et al., 2014), and fungi (Lorenz et al., 2012). Second, sequence alignment showed that AtFANCM and LsFANCM share high sequence similarity and feature the same protein domains (Supplemental Figure S1), indicative of similar functions between them. Third, a complementation test demonstrated that LsFANCM substitutes for AtFANCM in the regulation of NCO formation by possibly activating the SDSA pathway (Figure 2). Fourth, both LsFANCM and AtFANCM are required for the proper distribution of COs (Figures 8 and 9). Therefore, the differences observed during of meiotic recombination in Lsfancm and Atfancm might be triggered by additional factors that operate within the recombination machinery in the two species rather than because of a functional divergence between AtFANCM and LsFANCM.
Previous studies showed that, in Arabidopsis inbred lines, depletion of AtFANCM causes accumulation of massive aberrant intermediates, which are thought to be resolved by MUS81 to generate Class-II COs (Crismani et al., 2012). Therefore, despite our observation that 7% of all chromosomes fail to form Class-I COs in Atfancm-9, an increase in the activity of MUS81 may guarantee that every chromosome pair forms at least one CO. However, the anti-CO activity of AtFANCM is nearly absent in hybrids (Girard et al., 2015; Ziolkowski et al., 2015), suggesting that the capacity of FANCM in limiting meiotic COs is also regulated by associated genetic factors.
It is plausible that Atfancm meiocytes could form univalents in hybrids. Lsfancm mutants in this study were generated from an inbred line background. A complementation assay demonstrated that LsFANCM can direct NCO formation possibly through the SDSA pathway. In Lsfancm, aberrant intermediates may accumulate likely due to the attenuated SDSA activity. Unlike in Arabidopsis inbred lines where MUS81-dependent resolution of these intermediates yields COs, we think this alternative pathway either does not function or does not exist in lettuce meiosis. Therefore, the formation of Class-II COs might be unaltered in Lsfancm, as seen with Atfancm in hybrids.
Another possibility is that an increase in the formation Class-II COs is not enough to guarantee obligate CO formation for all bivalents when COs are maldistributed. However, no chromosome fragments were observed in Lsfancm, suggesting that those aberrant intermediates are likely processed by another NCO-generating pathway. One possible pathway lies in the double Holiday Junction dissolution pathway mediated by TOP3a (Topoisomerase 3a) and RMI1 (RecQ mediated instability1), which has been reported to play an important role in dissolving recombination intermediates and generating NCOs (Chelysheva et al., 2008; Hartung et al., 2008). In WT lettuce, the average of Class-I COs per bivalent is about 1.5, which is less than about 2 in Arabidopsis, but close to 1.3 in tomato, another asterids member (Anderson et al., 2014). Thus, the distribution of Class-I COs might be more sensitive to the functional FANCM in lettuce than in Arabidopsis; this notion is supported by the observation that 7% of bivalents lack Class-I CO formation in Atfancm-9, but about 18% of all chromosome pairs lose Class-I CO formation in Lsfancm, as evidenced by an increase in univalents.
In summary, our findings have at least two interpretations. On the one hand, our results have uncovered that AtFANCM and LsFANCM show differences in their requirement for regulating bivalent formation, because 78% Lsfancm meiocytes show univalents, whereas those of Atfancm do not. On the other hand, we show that both AtFANCM and LsFANCM are required for the appropriate distribution of COs, which could be a role conserved in plants. However, the different phenotypes observed in Lsfancm and Atfancm could be caused by additional factors or alternative processes that are affected by FANCM or its effectors in the two species rather than by the functional divergence in the proteins themselves.
Materials and methods
Plant materials and growth conditions
Arabidopsis thaliana (Columbia ecotype) mutants used in this study are listed below: Atfancm-9 (SALK_120621 or N620621; Crismani et al., 2012), Atmsh4 (SALK_136296; Higgins et al., 2004), Athei10-2 (SALK_014624; Chelysheva et al., 2012). Double mutants were generated by crossing single mutants and identified in the F2 generation. Arabidopsis plants were grown in a greenhouse at constant 22°C under a 16-h light and 8-h dark cycle. The lettuce (Lactuca sativa L.) variety used here was Japanese Butter Head (a inbred line; Jingu Horticulture Company, Tianjin, China) belonging to the butterhead horticultural type. Lettuce plants were grown in illumination incubators under a cycle of 16 h of light at 25°C and 8 h of dark at 17°C.
RT-qPCR assay
Total RNAs were extracted with TRIzol (15596026, Invitrogen, USA). cDNA was synthesized with a reverse transcription kit (RR047A, Takara, Japan). RT-qPCR was performed with a CFX96 Touch machine (Bio-Rad, USA) with SYBR Green as fluorochrome (#5125, Bio-Rad, USA). Gene expression levels were calculated by the 2−ΔΔCt method and normalized with the expression level of lettuce 18S rRNA. All the expression levels were the average of three biological replicates. The sequences of primers are listed in Supplemental Table S1.
β-GUS staining assay
The LsFANCM promoter 1,889 bp in length was amplified using high-fidelity polymerases (P515-AA, Vazyme, China) and was inserted into pCAMBIA1301 vector between PstI and NcoI sites upstream of the GUS reporter gene. The transgenic lettuce plants were generated by Agrobacterium-mediated transformation as reported previously with some minor modifications (Yu et al., 2017). Lettuce cotyledons were used as explants and regeneration was performed by somatic organogenesis. GUS expression was assayed according to a method described previously (Yao et al., 2018). GUS signals were observed and photographed using a Zeiss Stereo Discovery stereomicroscope (Zeiss, Heidelberg, Germany) and a Zeiss Axio Scope A1 microscope (Zeiss, Heidelberg, Germany).
Complementation vector construction and Arabidopsis transformation
For the genetic complementation assay, the ORF of LsFANCM was amplified and ligated into the pCAMBIA1306 vector by the NOVOREC PLUS DNA recombinase (NR001A, Novoprotein, China). The original CaMV35S promoter was replaced by the 2,593-bp AtFANCM promoter using EcoRI/SacI to drive the expression of LsFANCM. The ProAtFANCM::LsFANCM constructs were introduced into Agrobacterium GV3101. The Atfancm-9 Atmsh4 double mutants were infected through the floral dip method (Zhang et al., 2006). Positive T1 lines were screened on 1/2* MS plates containing 25 mg/L hygromycin for 2 weeks. Positive transformants were transferred to soil and grown in a greenhouse at a constant temperature of 22°C under a cycle of 16 h of light and 8 h of darkness.
Morphological analysis of plants
Whole plants were photographed by a digital camera (D7100, Nikon, Japan). Images of lettuce florets and seeds were taken using a Zeiss Stereo Discovery stereomicroscope (Zeiss, Heidelberg, Germany). Alexander Red staining was used to distinguish viable pollen grains from aborted ones (Peterson et al., 2010). The inflorescences were incubated with Alexander Red at 65°C for 40 mins and washed three times with 60% (v/v) glycerol in ddH2O. Images of the anthers or pollen grains were collected using a Zeiss Axio Scope A1 microscope (Zeiss, Heidelberg, Germany).
Generation of genome-edited lettuce plants
Three target sites were manually selected in the first half of the LsFANCM coding region and screened by BLAST against the lettuce reference genome (https://lgr.genomecenter.ucdavis.edu/) to avoid off-targeting. The target site sequences were cloned into the binary CRISPR/Cas9 vector pHSN401 as previously described (Xing et al., 2014). The Cas9 gene was codon optimized according to the codon preference of maize (Zea mays) and was driven by the CaMV35S promoter. The gRNA was transcribed by the Pol III AtU6 promoter. The resulting binary vectors were transformed into Agrobacterium strain GV3101. The transgenic lettuce plants were generated by Agrobacterium-mediated transformation (Yu et al., 2017). 17.0 mg·L−1 hygromycin was used to screen for positive transgenic plants. The edited plants were identified by amplicon sequencing. Genomic DNA was extracted from the leaves of transgenic plants using the CTAB method. PCR was performed using primer pairs spanning the target site. After purification, the PCR products were sequenced to detect the types of mutation. Primers for amplification and sequencing are listed in Supplemental Table S1.
Chromosome behavior observation
Chromosome spreads of Arabidopsis male meiocytes were prepared as described previously (Wang et al., 2014) and stained with DAPI (Vector Laboratories, CA, USA). The images were observed using a Zeiss Axio Imager A2 fluorescence microscope (Zeiss, Heidelberg, Germany). Chromosome spreads of lettuce male meiocytes were similar to the method for Arabidopsis, with minor modifications. The capitula 1.5–2 mm in length were fixed in Carnoy’s fixative (3 ethanol and 1 acetic acid, v/v%) for at least 4 h and digested by 1/2* digestion cocktail (0.5% (w/v) cytohelicase, 0.3% (w/v) cellulase, and 0.3% (w/v) pectolyase in 10 mM citrate buffer, pH 4.5) for 20 min at 37°C. Then, we washed the samples with 10-mM citrate buffer (4.45-mM sodium citrate and 5.55-mM citric acid) and collected the anthers from florets under a dissection microscope. After crushing the anthers on a slide, 25 μL 60% (v/v) acetic acid in ddH2O was mixed to the sample for 30 s on a heat block set at 45°C and chromosomes were spread out with 75-μL Carnoy’s fixative pre-chilled at −20°C. When the slides were dry, 5-μL DAPI were added and then covered with cover slips. Chromosome observation and image processing methods were the same as those for Arabidopsis.
Immunostaining assay
Immunostaining in lettuce was similar to the method used in Arabidopsis with some minor modifications (Armstrong et al., 2009). For the assay of LsHEI10, the meiocyte samples were prepared as Chromosome spreads. And for the assay of LsZYP1 and γH2AX, capitula 1.5–2 mm in length were fixed in 2% (w/v) paraformaldehyde with 0.1% (v/v) Triton X-100 vacuum infiltrated three times, each for 10 min. After fixation, the capitula were digested using an enzyme cocktail (0.5% (w/v) cytohelicase, 0.3% (w/v) cellulase, and 0.3% (w/v) pectolyase in 10 mM citrate buffer 4.5) for 30 min at 37°C. Then the samples were washed with ddH2O and the anthers were placed on slides under a dissection microscope. After the anthers were crushed, samples were covered with a cover slip and squashed gently using a glass rod. Then the slides were plunged in liquid nitrogen for 1 min and the cover slip was removed with a blade. The samples were either used immediately for immunostaining assay or stored in –80°C for up to 3 months. Anti-LsZYP1 antibody, Anti-LsHEI10 antibody, and Anti-AtHEI10 antibody were generated by raising peptides QNLNERKEKENTD, SPMKRPQLSRTRPQ, and PKDEIWPARQNS in rabbits, respectively (Ango Biotechnology, Shanghai, China). Rabbit-sourced polyclonal anti-γ-H2AX antibodies were used as described previously (Su et al., 2017). All the primary antibodies were diluted 1:200 in Goat serum (AR0009, Bosterbio, China). Secondary antibodies of Alexa Fluor® 555 Goat Anti-Rabbit IgG (H + L; A-21428, Invitrogen, USA) were used at a1:1,000 dilution rate. Images were captured with a Zeiss Axio Scope A1 microscope (Zeiss, Heidelberg, Germany).
Statistical methods
Data were analyzed statistically using Microsoft Excel 2007 (Microsoft, USA) for calculating the mean, the standard deviation. GraphPad Prism 5 was used to prepare scatter and bar diagrams and perform Mann–Whitney test, Wilcoxon signed rank test and Chi-square test. The reported P-values are either exact values or Gaussian approximations.
Phylogenetic analysis
Sequences for putative FANCM homologs in representative plant species were chosen according to the BLAST results online (https://phytozome.jgi.doe.gov/pz/portal.html). The full-length protein sequences were aligned by MUSCLE using default parameters. The phylogenetic tree was constructed using MEGA6 using the neighbor-joining method with 1,000 bootstrap replicates.
Accession numbers
The full-length amino acid sequences of lettuce genes were obtained from Lettuce Genome Resource (https://lgr.genomecenter.ucdavis.edu/) as the flowing accession numbers: LsFANCM (Lsat_1_v5_gn_8_141780), LsZYP1 (Lsat_1_v5_gn_1_3120), LsHEI10 (Lsat_1_v5_gn_7_101801). The Arabidopsis genes were obtained from the Arabidopsis database TAIR (https://www.arabidopsis.org/): AtFANCM (At1g35530); AtMSH4 (AT4G17380); and other amino acid sequences used in the phylogenetic analysis were obtained from Phytozome (https://phytozome.jgi.doe.gov/pz/portal.html) as per the following accession numbers: BsFANCM (Bostr.1774s0022); CrFANCM (Carubv10008097m); EsFANCMa (Thhalv10001810m); EsFANCMb (Thhalv10002370m); BrFANCM (Brara.E01856); TcFANCM (Thecc1EG036398); MtFANCM (Medtr1g103260); GmFANCM (Glyma.08G238200); VvFANCM (GSVIVG01034779001); MgFANCM (Migut.C01287); AcFANCM (Aqcoe6G016100); PhFANCM (Pahal.H00926); SvFANCM (Sevir.8G059600); SbFANCM (Sobic.005G061900); OsFANCM (Os11g07870); BdFANCM (Bradi4g23960); SpFANCM (Spipo9G0042900); AtrFANCM (evm_27.TU.AmTr_v1.0_scaffold00064); PpFANCM (Pp3c20_9530); SfFANCM (Sphfalx0049s0059); OlFANCM (e_gwEuk.7.70.1).
Supplemental data
The following materials are available in the online version of this article.
Supplemental Figure S1. Sequence alignment of the helicase region of representative plant FANCM homologs.
Supplemental Figure S2. Phylogenetic analysis of FANCM in plants.
Supplemental Figure S3. Identification of positive transgenic plants expressing LsFANCM in the Atfancm-9 Atmsh4 double mutant.
Supplemental Figure S4. Expression analysis of LsFANCM in Lsfancm mutants and WT.
Supplemental Figure S5. Phenotype observation of Lsfancm-2 and Lsfancm-3.
Supplemental Figure S6. Validation of antibodies by immunostaining.
Supplemental Figure S7. Immunostaining of LsZYP1 at leptotene and zygotene.
Supplemental Figure S8. Diagrammatic representations of the most common bivalent shapes observed at metaphase I in lettuce.
Supplemental Table S1. The sequence of primers used in this study.
Acknowledgments
We greatly thank both Kees van Dun and Cilia L. C. Lelivelt at the Rijk Zwaan company (Netherlands) for initiating the project and for constructive discussion during the preparation of the manuscript, Qijun Chen at China Agricultural University for kindly sharing the pHSN401 vector, Aiwu Dong at Fudan University for providing the anti-γ-H2AX antibody and Linbo Wang at Fudan University for his help in phylogenic analysis.
Funding
This work was supported by Rijk Zwaan (Netherlands) and National Natural Science Foundation of China (31925005 and 31870293), and Fudan University.
Conflict of interest statement. The authors declare that they have no competing interests.
X.L. and Y.W. conceived and designed the experiments; X.L., M.Y., D.N., and J.Z. performed the experiments; X.L., D.N., and Y.W. analyzed the data; X.L., H.M., P.BV., and Y.W. wrote and revised the manuscript; all authors read and approved the final version of the manuscript.
The author responsible for distribution of materials integral to the findings presented in this article in accordance with the policy described in the Instructions for Authors (https://dbpia.nl.go.kr/plphys/pages/general-instructions) is: Yingxiang Wang ([email protected]).
References
Author notes
Senior author.