-
PDF
- Split View
-
Views
-
Cite
Cite
Cyril Charbonnel, Oleh Rymarenko, Olivier Da Ines, Fatiha Benyahya, Charles I. White, Falk Butter, Simon Amiard, The Linker Histone GH1-HMGA1 Is Involved in Telomere Stability and DNA Damage Repair, Plant Physiology, Volume 177, Issue 1, May 2018, Pages 311–327, https://doi.org/10.1104/pp.17.01789
- Share Icon Share
Abstract
Despite intensive searches, few proteins involved in telomere homeostasis have been identified in plants. Here, we used pull-down assays to identify potential telomeric interactors in the model plant species Arabidopsis (Arabidopsis thaliana). We identified the candidate protein GH1-HMGA1 (also known as HON4), an uncharacterized linker histone protein of the High Mobility Group Protein A (HMGA) family in plants. HMGAs are architectural transcription factors and have been suggested to function in DNA damage repair, but their precise biological roles remain unclear. Here, we show that GH1-HMGA1 is required for efficient DNA damage repair and telomere integrity in Arabidopsis. GH1-HMGA1 mutants exhibit developmental and growth defects, accompanied by ploidy defects, increased telomere dysfunction-induced foci, mitotic anaphase bridges, and degraded telomeres. Furthermore, mutants have a higher sensitivity to genotoxic agents such as mitomycin C and γ-irradiation. Our work also suggests that GH1-HMGA1 is involved directly in the repair process by allowing the completion of homologous recombination.
Eukaryotic chromosomes are linear and thus harbor DNA ends. If left unprotected, the cell recognizes these ends as DNA double-strand breaks (DSBs) and activates the DNA-damage response (DDR), resulting in chromosome fusions and, eventually, cancer or cell death. Telomeres are repetitive sequences at the ends of chromosomes important for their protection. Although minor differences in telomeric DNA sequence exist between species, telomeric DNA is composed of several kilobases of G-rich double-stranded telomeric repeats ending with a 3′ single-strand overhang. However, telomeric proteins are more diverse. In mammals, telomere protection is provided mainly by shelterin, a complex of six core subunits: Telomeric Repeat Binding Factor1 (TRF1), TRF2, Protection of Telomere1 (POT1), TERF1-Interacting Nuclear Factor2, POT1-Interacting Protein1, and Repressor/Activator Protein1, that prevents inappropriate recombination and fusion between telomeres. The complex also plays an important role in telomere replication and length regulation (de Lange, 2009). TRF1 and TRF2 bind the double-stranded DNA region of the telomere through a single myb-like DNA-binding domain that contains the telobox motif required for specific telomeric sequence recognition, while POT1 binds to the 3′ overhang via two oligonucleotide/oligosaccharide-binding fold domains. In contrast, in budding yeast (Saccharomyces cerevisiae), the Rap1-Rif1-Rif2 complex binds the double-stranded DNA and the Cdc13-Stn1-Ten1 (CST) complex recognizes the single-strand overhang (Wellinger and Zakian, 2012).
Despite intensive search for proteins involved in telomere end protection in plants, none with a similar functionality to the shelterin complex has been reported (Amiard et al., 2011b, 2013; Procházková Schrumpfová et al., 2016). Structure prediction has identified 15 TRF-like proteins in Arabidopsis (Arabidopsis thaliana) with a single telobox Myb domain at the C or N terminus (Pe¡ka et al., 2011), and recently, one of these candidates, Telomere Repeat Binding1 (TRB1), was shown to colocalize with telomeres (Schrumpfová et al., 2014). TRB1 mutants, however, show no obvious telomeric defects, except a slight decrease in telomere length. Another putative shelterin-like candidate protein, Telomeric DNA Binding Protein1, was characterized in Arabidopsis and shown to play a role in telomere length regulation (Hwang and Cho, 2007).
The only characterized Arabidopsis proteins acting directly in telomere end protection are the homologs of the yeast and mammalian CST complex. Mutation in any Arabidopsis CST subunit (CTC1, STN1, or TEN1) leads to severe morphological defects and is accompanied by massive genomic instability, telomere length decrease, elongated single-strand G overhangs, telomeric fusions, and the appearance of extrachromosomal telomeric circles (Song et al., 2008; Surovtseva et al., 2009; Leehy et al., 2013). In plant cells, the CST complex directly represses the ATR-dependent DDR pathway (Amiard et al., 2011a; Boltz et al., 2012). This mode of protection is restricted to single-strand overhangs, which are recognized by the CST complex. However, half of the Arabidopsis telomeres are not resected by EXO1 and, therefore, are blunt ended. In these cases, it was proposed that telomere end protection is achieved via binding of the heterodimer KU70/80. These two mechanisms seem to balance: in the absence of KU (ku mutant), the extremities are resected by EXO1 and then can be protected by the CST complex (Kazda et al., 2012; Nelson and Shippen, 2012). Both the CST complex and the KU70/80 heterodimer are not solely telomeric but also function in general genome maintenance, with CST being necessary for the completion of replication of challenging DNA regions, including telomeres, in human (Homo sapiens) and Arabidopsis (Chen and Lingner, 2013; Derboven et al., 2014).
With the aim of identifying putative Arabidopsis telomeric interactors, we performed a telomere pull-down combined with label-free quantitative mass spectrometry. One of the identified proteins was the as yet uncharacterized High Mobility Group Protein A (HMGA) protein HON4, recently proposed to be renamed GH1-HMGA1 (Kotlinski et al., 2017). Three families of HMG proteins exist, HMGA, HMGB, and HMGN, according to the presence of a specific functional domain. In mammals, the HMGA family is composed of two proteins: HMGA1 and HMGA2. Both proteins are expressed in embryonic tissues and embryonic stem cells, are absent in most somatic adult cells, and, interestingly, are highly abundant in tumorigenic cells (Ozturk et al., 2014). They usually harbor three or four AT-hook motifs required for their binding to AT-rich DNA sequences. However, their binding depends more on structural components than on the exact DNA sequence. It has been reported that HMGA proteins compete with histone H1 for binding to linker DNA, inducing the loosening of chromatin structure (Catez and Hock, 2010; Ozturk et al., 2014). Thus, they are believed to play a role in transcription by promoting the joining of regulatory elements and have been shown to have a clear role in development: HMGA1 knockout mice (Mus musculus) are smaller and suffer from cardiac hypertrophy, have lesser ability of cells to differentiate, and are sterile, whereas transgenic mice overexpressing a truncated version of HMGA2 are giant, obese, and develop lymphomas (Ozturk et al., 2014).
Another property of these proteins has emerged recently: their DNA protective function in cancer cells or in response to genotoxic treatment. Very recent work places HMGA1 in a complex with DNA-PK to enhance ligase IV activity (Pellarin et al., 2016). In cells overexpressing HMGA2, the presence of DNA-PKcs at these DSB sites is prolonged and alters the binding of KU70/80. This results in the alteration of DNA repair dynamics and an increase in spontaneous chromosome aberrations (Li et al., 2009). Furthermore, HMGA2 may be involved in DNA base excision repair (Summer et al., 2009) and has been reported to be a replication fork chaperone at ongoing and stalled forks (Yu et al., 2014). Both HMGA1 and HMGA2 have dRP/AP lyase activity, are substrates of ataxia telangiectasia mutated (ATM; Pentimalli et al., 2008; Palmieri et al., 2011), and positively regulate ATM expression via a positive feedback loop in response to DNA damage. Consequently, down-regulation of HMGA proteins reduces ATM expression and enhances cancer cell sensitivity to DNA damage. These two high mobility group proteins are frequently overexpressed in cancer cell lines, and there are some indications of links to telomeres. Our work has indicated a role for HMGA1 in TERRA localization to telomeres (Scheibe et al., 2013). HMGA2 positively regulates the transcription of the catalytic subunit of the telomerase in HeLa cells (Li et al., 2011) and increases telomere stability in cancer cells (Natarajan et al., 2016).
Plant HMGA proteins are expressed ubiquitously and have a GH1 domain (histone H1 nucleosome-binding globular domain) not found in their animal homologs (Jerzmanowski et al., 2000; Kotlinski et al., 2017). The maize (Zea mays) HMGA protein binds AT-rich sequences in vitro and in vivo and localizes to the nucleolus (Zhao et al., 2009). AtHMGA (recently renamed GH1-HMGA3) is located in the nucleus and excluded from the nucleolus (Launholt et al., 2006). In Arabidopsis, several uncharacterized HMGA proteins are present, including GH1-HMGA1 (Kotlinski et al., 2017). Here, we show that GH1-HMGA1 is a chromatin protein important for correct plant development and is required for DNA repair and telomere stability.
RESULTS
Identification of GH1-HMGA1 as a Telomeric Binding Factor
To identify Arabidopsis telomeric proteins, we performed a label-free quantitative proteomics analysis of a telomere pull-down (Casas-Vila et al., 2015; Kappei et al., 2017) with either the Arabidopsis TTTAGGG repeat sequence or a shuffled DNA control. We identified several candidate proteins, including TRB1, TRB3, and GH1-HMGA1 (At3g18035) enriched with the telomeric bait (Fig. 1, A and B). GH1-HMGA1 together with GH1-HMGA2 and GH1-HMGA3 belong to the HMGA-like protein family in plants (Kotlinski et al., 2017), which, in contrast to the mammalian HMGA proteins, features an additional H1/H5 linker domain usually present in histone H1 (Supplemental Fig. S1). Among them, GH1-HMGA3 is considered as the canonical HMGA protein, with four well-defined AT-hook domains. GH1-HMGA1 and GH1-HMGA2 are more than double in length compared with GH1-HMGA3, share 50% identity (BLAST e-value, 2e-106), and contain six AT-hooks (Supplemental Fig. S1; Kotlinski et al., 2017).
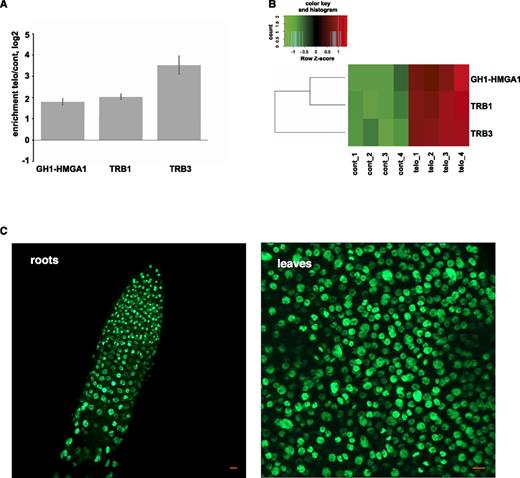
GH1-HMGA1 associates with chromatin in vivo. A, Pull-down enrichment with the telomeric probe compared with the scrambled control sequence probe. The average fold enrichment for each protein was determined from replicates (n = 4). Error bars represent sd. B, Z-scored LFQ values of the enrichment of the three proteins between the quadruplicates. C, Live imaging of a root tip and of a section of a rosette leaf of Arabidopsis plants transformed with the GH1-HMGA1-GFP fusion construct. Bars = 10 μm.
GH1-HMGA1 Associates with Chromatin in Vivo
To check the localization of GH1-HMGA1 in vivo, we created transgenic lines expressing a GFP-tagged GH1-HMGA1 protein under the control of its own promoter. In three independent lines, the GH1-HMGA1-GFP fusion protein was expressed abundantly in the nuclei of roots and leaves (Fig. 1C), matching previous transcript-based evidence from publicly available Arabidopsis RNA sequencing data sets revealing an abundant expression in all tissues and all developmental stages (Supplemental Fig. S2).
To evaluate more precisely the nuclear localization of GH1-HMGA1-GFP, we performed coimmunofluorescence studies with the centromeric CENH3 histone variant (Fig. 2A) and immuno-FISH (for fluorescence in situ hybridization) using telomeric probes (Fig. 2B). These experiments revealed that GH1-HMGA1 is highly and broadly expressed both in mitotic (Fig. 2A) and interphase (Fig. 2B) nuclei. In Figure 2A, we observed that the GFP signal seems to be excluded from the CENH3 signal. This exclusion is confirmed in Figure 2B, with the GFP signal that is excluded from the chromocenters (yellow arrows) and from the nucleoli (green arrows). We next wondered whether GH1-HMGA1 colocalized with telomeric DNA. Using the colocalization module of the ZEN software, we analyzed 92 and 83 telomeric foci (from two independent experiments [10 nuclei in each]) and calculated the Pearson’s correlation coefficient for each focus (Supplemental Fig. S3). The results indicated that around 30% (33.7% and 29.9%) of the telomeric signals are devoid of GH1-HMGA1 proteins. The remainder is divided into two categories, with around half of the signal between 0 and 0.5 (considered as partially colocalized) and half between 0.5 and 1 (considered as fully colocalized). This experiment indicates that GH1-HMGA1 can be present at some DNA extremities but is not associated exclusively with the telomeres.
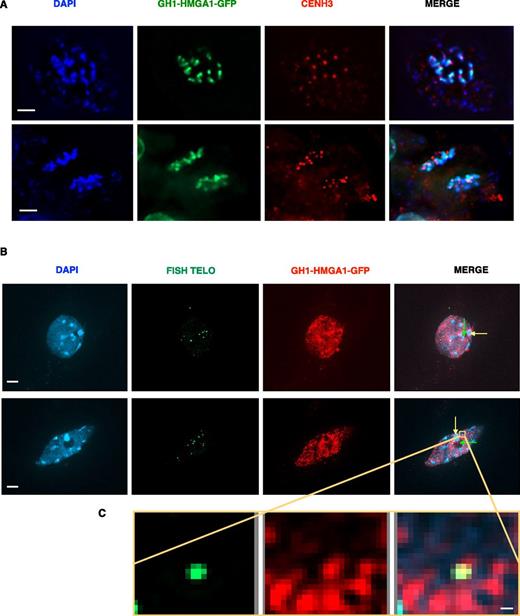
GH1-HMGA1 is a chromatin protein. A, Double immunostaining of a mitotic root tip nucleus of a GH1-HMGA1-GFP transformant with anti-GFP and anti-CENH3 antibodies. DNA is stained with 4′,6-diamino-phenylindole (DAPI; blue), GFP signal is colored in green, and CENH3 signal is colored in red. Bars = 2 μm. B, GFP immunostaining and telomeric FISH labeling of leaf nuclei of GH1-HMGA1-GFP plants. Nuclei were stained with DAPI (blue), FISH signals are colored in green, and GH1-HMGA1-GFP is colored in red. Images are collapsed Z-stack projections. Yellow arrows indicate examples of chromocenters without GH1-HMGA1 signal, and green arrows indicate examples of nucleoli devoid of GH1-HMGA1 signal. Bars = 2 μm. C, Closeup view showing colocalization between GH1-HMGA1 and one telomere. See also Supplemental Figure S3. Bar = 0.1 μm.
gh1-hmga1 Mutants Exhibit Developmental Growth Defects
To examine the functional role of GH1-HMGA1 in vivo, two independent T-DNA insertion mutant lines were characterized. The gh1-hmga1-1 line (SAIL_215_D04) has a T-DNA insertion in the second exon and no detectable full-length transcripts, while in the gh1-hmga1-2 line (SALK_ 099887C), the T-DNA insertion is in the last exon and produces a truncated transcript (Supplemental Fig. S4).
After self-fertilization of heterozygous gh1-hmga1-1 plants, only 4.3% (32 of 743) of the progeny were homozygous mutants, rather than the 25% expected from Mendelian segregation. All of these gh1-hmga1-1 mutant plants exhibited impaired growth, short roots, small and sharp leaves, short inflorescences, and total sterility (Fig. 3, A and D), demonstrating an important role of GH1-HMGA1 in plant development. The gh1-hmga1-2 mutants showed a similar developmental phenotype to gh1-hmga1-1 mutants (Fig. 3B), but the alleles segregate at the expected Mendelian ratio: one-fourth of progeny from selfed heterozygous parents were homozygous gh1-hmga1-2 mutants. Due to the sterility of gh1-hmga1-1 plants, we performed most of the further in-depth characterization with the gh1-hmga1-2 line.
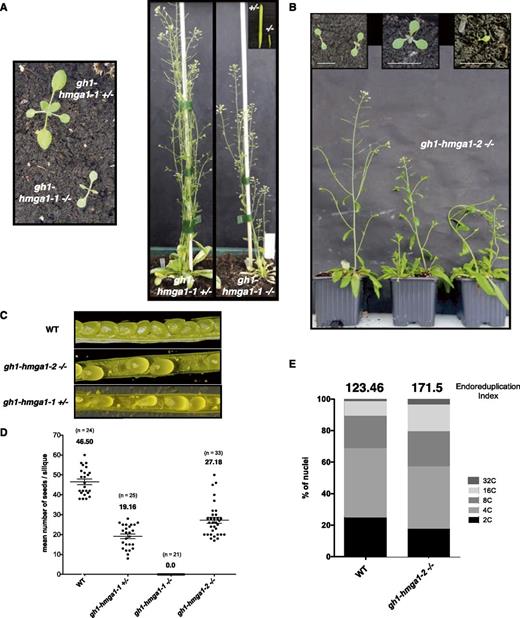
Characterization of gh1-hmga1 T-DNA lines. A and B, Images representing the developmental growth of gh1-hmga1-1 (A) and gh1-hmga1-2 (B) mutants. C, Images comparing the siliques from the wild type (WT) and gh1-hmga1-1 and gh1-hmga1-2 mutants. D, Number of seeds per silique. Black circles represent raw data, and horizontal lines represent means with error bars (±se); n = the number of analyzed siliques. E, Distribution of mitotic nuclear DNA contents in 1-week-old seedlings of wild-type and gh1-hmga1-2 mutant plants determined by flow cytometry. The endoreduplication index (EI) represents the average number of endocycles undergone by a typical nucleus (EI = 1*4C+2*8C+3*16C; Takahashi et al., 2008).
Measurement of ploidy levels by flow cytometry showed a higher percentage of nuclei with 16C or 32C content in the gh1-hmga1-2 mutants, characteristic of genomic instability (Fig. 3E). While, in contrast to gh1-hmga1-1, gh1-hmga1-2 plants were not sterile, they showed decreased fertility, manifested by approximately half the number of seeds per silique compared with wild-type plants (Fig. 3, C and D). We also observed a low percentage of defective cotyledon phenotypes, including monocotyledonous seedlings, seedlings with partially fused cotyledons, and tricots (Fig. 3B).
GH1-HMGA1 Participates in DDR
Previous studies in mammals have described a role for HMGA proteins in the DDR process (Reeves, 2015). In order to determine whether the Arabidopsis GH1-HMGA1 also is involved in DDR, we performed DNA damage sensitivity assays with the gh1-hmga1-2 mutants. Seven-day-old seedlings were irradiated at 100 Gray (Gy), and the number of sensitive plants was counted 7 d after irradiation. As illustrated in Figure 4A, gh1-hmga1-2 mutants were hypersensitive to γ-irradiation, with less than 20% of plants showing resistance, a level equivalent to our positive control, a triple knockout of three major DNA repair proteins (ku80 xrcc1 xpf; Charbonnel et al., 2011). gh1-hmga1-2 mutants also were hypersensitive to mitomycin C (MMC), a DNA cross-linking agent (Fig. 4, B and C). However, the absence of GH1-HMGA1 did not influence sensitivity to treatment with hydroxyurea, a powerful agent affecting DNA replication (Fig. 4D).
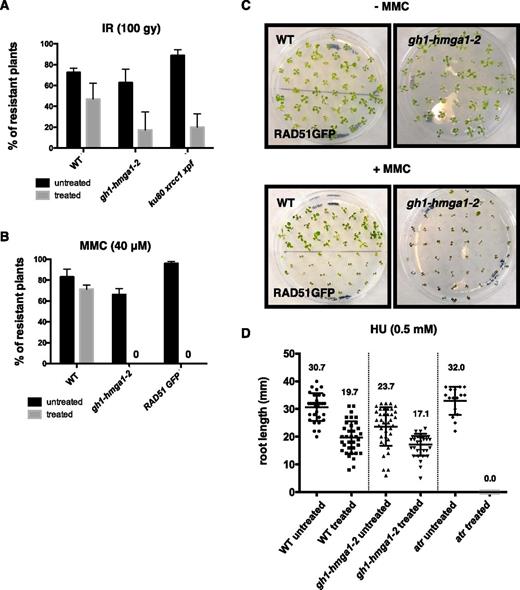
GH1-HMGA1 is involved in DNA damage repair. A, DNA damage sensitivity assay with seedlings treated with 100 Gy irradiation (IR). The DNA damage-sensitive mutant ku80 xrcc1 xpf was used as a control. Error bars indicate the se of three biological replicates with 50 seedlings each. B, DNA damage sensitivity assay with seedlings treated with 40 µm MMC. The RAD51-GFP line was used as a control (Da Ines et al., 2013). Error bars indicate the se of three biological replicates with 50 seedlings each. C, Representative plates showing control plants and plants grown 14 d in the presence of 40 µm MMC. D, DNA damage sensitivity assay of seedlings treated with 0.5 mm hydroxyurea (HU). Quantification is shown for the root length of plants grown on control medium or on medium supplemented with 0.5 mm hydroxyurea (n = 30). Black circles represent raw data. Error bars indicate the se. The atr mutant line was used as a positive control for hydroxyurea sensitivity. WT, Wild type.
To extend this analysis of GH1-HMGA1 in DNA DSB repair, we performed a time-course analysis of γ-H2AX foci after 25 Gy irradiation (Fig. 5, A–C). Ten minutes after irradiation, we counted an equivalent number of γ-H2AX foci in mutant and wild-type plants, revealing that GH1-HMGA1 does not seem to be required for the initial DSB signaling step (Fig. 5B). However, 1 h after irradiation, we observed that approximately 75% of DNA DSBs were repaired in the wild type but less than 40% were repaired in the gh1-hmga1-2 mutants (5.2 foci remaining in the wild type compared with 13.8 in gh1-hmga1-2; Fig. 5C). We can conclude from these experiments that the repair of DNA DSB is less efficient in absence of GH1-HMGA1.
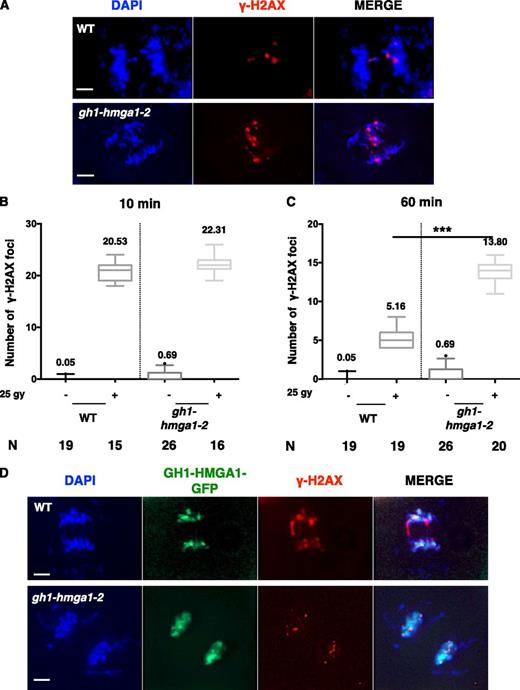
Time-course analyses of DNA damage signaling in the gh1-hmga1-2 mutant. A, Images of γ-H2AX immunostaining on root rip nuclei of the wild type (WT) and the gh1-hmga1-2 mutant 60 min after irradiation with 25 Gy. DNA is stained with DAPI (blue), and γ-H2AX signal is colored in red. Bars = 2 µm. B, Box plots of γ-H2AX foci counted on mitotic nuclei of root tips extracted from plants 10 min after irradiation with 25 Gy. The number (N) of nuclei analyzed is indicated. C, Box plots of γ-H2AX foci counted on mitotic nuclei of root tips extracted from plants 60 min after irradiation with 25 Gy. The number of nuclei analyzed is indicated. The difference in the number of foci 60 min after irradiation between the wild type and the mutant is significant, as determined by unpaired Student’s t test (***, P < 0.001). D, Double immunostaining with anti-GFP and anti-γ-H2AX antibodies of mitotic root tip nuclei of the wild type and a GH1-HMGA1-GFP transformant 10 min after irradiation with 25 Gy. DNA is stained with DAPI (blue), GFP signal is colored in green, and γ-H2AX signal is colored in red. Bars = 2 µm.
Using immunostaining, we next investigated whether GH1-HMGA1-GFP colocalizes with γ-H2AX foci after irradiation. As expected, GH1-HMGA1 displayed a diffuse localization on mitotic figures of untreated plants, but we did not observe any enrichment of GH1-HMGA1 at DSB sites in the mutant (Fig. 5D).
Homologous Recombination Is Impaired in gh1-hmga1 Mutants
Considering that the early phase of DNA repair was not affected (Fig. 5B), we assumed that the DNA repair defect observed in the absence of GH1-HMGA1 may originate from a defect in homologous recombination (HR), known to be activated at a later stage compared with the immediate action of nonhomologous end joining (Charbonnel et al., 2011). To test this hypothesis, we crossed our mutants with the recombination tester line IU-GUS (Orel et al., 2003) and counted the number of blue sectors (recombination events) in 150 F2 plants in three independent replicates. Figure 6A (and Supplemental Fig. S5) showed that the absence of full-length GH1-HMGA1 leads to a severe decrease in HR efficiency, with a decrease of roughly 10-fold in gh1-hmga1-2 mutants (4.59 in the wild type compared with 0.46 in gh1-hmga1-2).
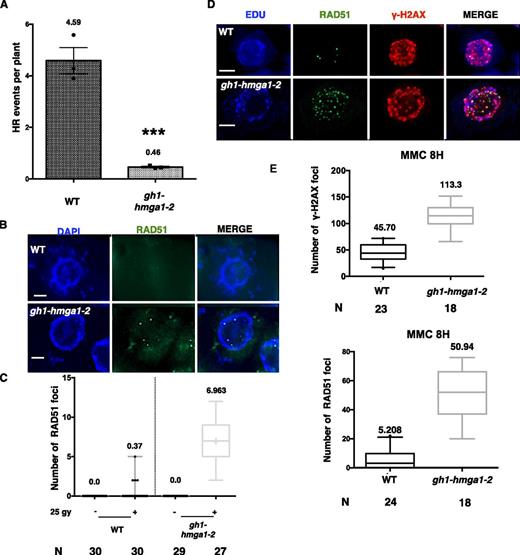
HR in the absence of GH1-HMGA1 proteins. A, Spontaneous somatic HR frequencies counted on three independent experiments (50 plants in each). Mean values are shown. Error bars represent se. ***, P < 0.0001 (Mann-Whitney t test). B, Immunostaining with anti-RAD51 antibody of mitotic root tip nuclei 60 min after irradiation with 25 Gy. DNA is stained with DAPI (blue), and RAD51 signal is colored in green. Bars = 2 µm. C, Box plots of RAD51 foci counted on S- and G2-phase nuclei of root tips extracted from plants 60 min after irradiation with 25 Gy. The number (N) of nuclei analyzed is indicated. D, Double immunostaining with anti-RAD51 and anti-γ-H2AX antibodies of mitotic root tip nuclei after 8 h of MMC treatment. S- and G2-phase nuclei are stained with EdU (blue), RAD51 signal is colored in green, and γ-H2AX signal is colored in red. Bars = 2 µm. E, Box plots of RAD51 and γ-H2AX foci counted on S/G2 nuclei of root tips extracted from plants after 8 h of treatment with MMC. The number of nuclei analyzed is indicated. WT, Wild type.
The decrease in HR activity could be due to inhibition of the major DNA repair genes, as reported in mammals, where HMGA1 inhibits BRCA1 transcription in breast cancer cells (Baldassarre et al., 2003). Therefore, we checked the expression of several genes encoding important components of HR. AtRAD51, AtPARP1, and AtBRCA1 transcript levels were not altered in nontreated or DNA damage-induced conditions in the gh1-hmga1-2 line (Supplemental Fig. S6). We further checked for a direct effect on RAD51 recruitment by counting nuclear RAD51 foci in irradiated or MMC-treated mutant plants compared with the wild type. The gh1-hmga1-2 plants had higher numbers of DNA damage-induced RAD51 foci than the wild-type controls. Thus, the reduced HR in gh1-hmga1-2 plants was not due to less recruitment of RAD51 in the absence of functional GH1-HMGA1 (Fig. 6, B–E). These observations argue for a role of GH1-HMGA1 in HR independent of RAD51 loading.
Investigations in yeast and mammals argue that HR is facilitated in the absence of histone H1 (Downs et al., 2003; Murga et al., 2007), and recent in vitro studies in mammalian cells show that eviction of H1 from chromatin is essential for efficient HR (Machida et al., 2014). Thus, the observed decrease in HR efficiency might result from a greater incorporation of H1 on chromatin. To test this, we performed an immunolabeling directed against histone H1 and evaluated the quantity of H1 on chromatin (Fig. 7). While the ratio between DAPI and H1 signals was 1.03 in the wild type, in the mutant, it increased to 1.44, corresponding to an increase of more than 40% in nuclei of the mutant compared with the wild type. This result suggests a higher incorporation of H1 in chromatin in the absence of GH1-HMGA1.
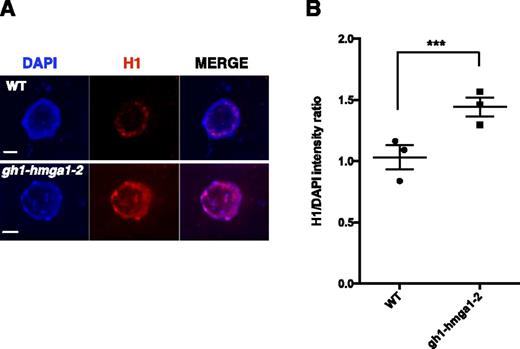
H1 fluorescence intensity in the gh1-hmga1-2 mutant. A, Immunostaining with anti-H1 antibody of root tip nuclei. DNA is stained with DAPI (blue), and H1 signal is colored in red. Bars = 2 µm. B, Box plots representing the H1-DAPI fluorescence intensity ratio measured from root tip nuclei in the gh1-hmga1-2 mutant compared with the wild type (WT). The asterisks indicate significant differences (unpaired Student’s t test, ***, P < 0.001). Data are from three independent experiments calculating the intensity of 25 S- and G2-phase nuclei coming from five different fields in each experiment (75 nuclei in total).
gh1-hmga1 Mutants Show Telomeric Deficiencies
Since we identified GH1-HMGA1 as a putative telomeric interactor (Fig. 1; Supplemental Fig. S3), we investigated whether its absence impacts telomere regulation and/or stability. Telomere restriction fragment (TRF) analysis revealed a telomere length decrease in the second mutant generation (G2) of both gh1-hmga1-1 and gh1-hmga1-2 mutants. However, the length of the shortened telomeres appeared to stabilize in the following generations (Fig. 8A; Supplemental Fig. S7), in contrast to the phenotype of tert mutants, for example.
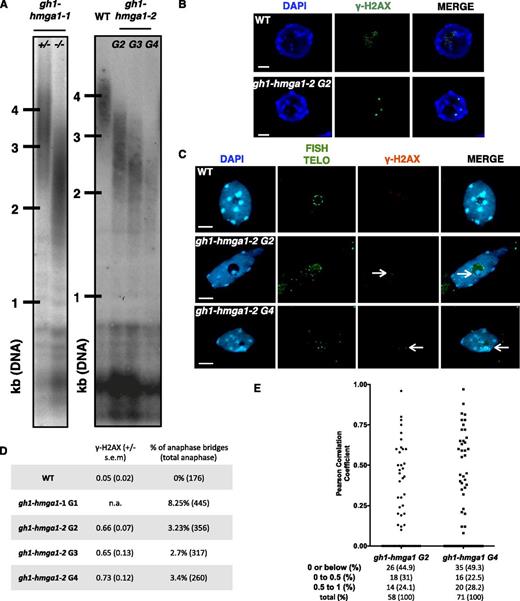
Telomere instability in the gh1-hmga1 mutants. A, TRF analyses of bulk telomere length in genomic DNA using telomeric repeat probes. For gh1-hmga1-1, DNA was extracted from either heterozygous (+/−) or mutant (−/−) G1 buds. For gh1-hmga1-2, DNA was extracted from seedlings at the second (G2), third (G3), and fourth (G4) generations. B, γ-H2AX immunofluorescence staining of root nuclei of the gh1-hmga1-2 mutant. Nuclei were stained with DAPI (blue), and γ-H2AX foci are colored in green. Images are collapsed Z-stack projections of a deconvolved three-dimensional image stack. Bars = 2 μm. C, Immuno-FISH staining: γ-H2AX immunostaining and telomeric FISH labeling of root tip nuclei of GH1-HMGA1-GFP plants. Nuclei were stained with DAPI (blue), FISH signals are colored in green, and γ-H2AX foci are colored in red. Images are collapsed Z-stack projections. Arrows indicate telomere-induced foci. Bars = 2 μm. D, Table presenting the mean number of γ-H2AX from five apices (100 nuclei in total) and the percentage of anaphase bridges. E, Range of the Pearson correlation coefficient of γ-H2AX/telomeres calculated with the colocalization module of the ZEN software using Z-stack files. The numbers of analyzed foci are indicated. Foci with coefficients below 0 are considered as noncolocalized, foci with coefficients between 0 and 0.5 are considered as partially colocalized, and foci with coefficients between 0.5 and 1 are considered as fully colocalized. WT, Wild type.
The effect of the absence of GH1-HMGA1 on telomeric stability was confirmed with cytological experiments. We first counted the number of γ-H2AX foci in root tip nuclei of the gh1-hmga1-2 mutant and found that, in contrast to wild-type plants, in which almost no γ-H2AX foci were observed, the gh1-hmga1-2 mutant harbored 0.66 γ-H2AX foci per nucleus (100 nuclei) at G2. Interestingly, and in concordance with the TRF analyses (above), this value did not increase in subsequent generations (0.65 in generation 3 and 0.73 in generation 4; Fig. 8, B and D). To evaluate the number of telomere-induced foci, we performed immuno-FISH experiments to analyze the coincidence of the γ-H2AX foci and telomeric DNA. We found that 44.9% of the γ-H2AX foci in G2 and 49.3% in G4 had a Pearson’s correlation coefficient below 0 (Fig. 8E), indicating that around half of the foci were not associated with telomeres. These foci may originate from deprotected telomeres too short to be detected by FISH or may have a nontelomeric origin, such as replication defects (Yu et al., 2014). We also detected 31% of foci in G2 and 22.5% in G4 with a Pearson’s correlation coefficient between 0 and 0.5 (partially colocalized) and 24.1% in G2 and 28.2% in G4 with a coefficient between 0.5 and 1 (considered as colocalized; Zinchuk and Zinchuk, 2008). Thus, at least one-quarter of the examined γ-H2AX foci may be formed at deprotected telomeres.
The presence of deprotected telomeres is expected to lead to chromosome fusions, forming dicentric bridges observable during anaphase. In the first generation of the gh1-hmga1-1 mutant, 8.25% of mitotic anaphase nuclei had visible anaphase bridges, and in gh1-hmga1-2 plants, 3.23% of mitoses had anaphase bridges in G2, compared with wild-type plants, where anaphase bridges were rarely seen (Fig. 8D). As seen with TRF analyses and numbers of γ-H2AX foci, we did not observe a further increase in the number of telomeric fusions in generations 3 and 4.
To analyze whether the decrease in telomeric length depended on telomerase, we crossed the gh1-hmga1-2 line with the tert line (mutated for the catalytic subunit of telomerase). A dramatic increase in developmental growth defects was seen in generation 4 (Supplemental Fig. S8, A and B) of the double mutant tert gh1-hmga1, with only 6.6% fertile plants (Fig. 9A), a level usually seen in generation 7 tert plants (Riha et al., 2001). This was accompanied by high levels of dead cells in root meristems (Fig. 9B; Supplemental Fig. S8C) and an increase in the number of both γ-H2AX foci and telomeric fusions in the fourth generation of the double mutant lines compared with the single mutants (Fig. 9, C and D). Finally, the analysis of telomeric DNA length confirmed a greater telomere loss in tert gh1-hmga1 double mutants compared with the tert and gh1-hmga1 plants (Fig. 9E), indicating that GH1-HMGA1 and telomerase are involved in two different pathways to regulate telomeric elongation.
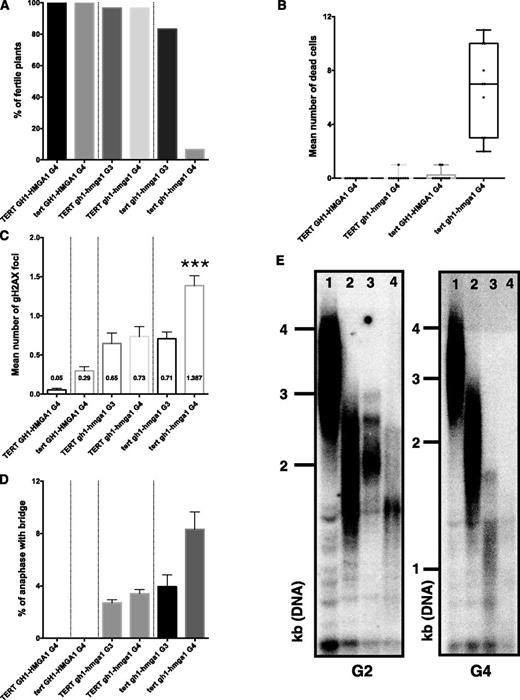
Telomeric insufficiencies are independent of telomerase. A, Percentage of fertile plants (n = 60 plants) in the fourth generation (G4) of TERT GH1-HMGA1 and tert GH1-HMGA1 mutants and in the third (G3) and fourth generations of TERT gh1-hmga1 and tert gh1-hmga1 mutants. B, Graphic representing the number of dead cells counted in 10 root tips stained with propidium iodide. C, Mean number (and se) of γ-H2AX foci from five apices (100 nuclei in total). The asterisks indicate a significant difference between TERT gh1-hmga1 and tert gh1-hmga1 mutants in G4 (unpaired Student’s t test, ***, P < 0.001). D, Mean percentage (and se) of anaphases presenting at least one bridge from at least 50 anaphases from three different experiments in each case. E, TRF analyses of bulk telomere length in genomic DNA using telomeric repeat probes. DNA was extracted from seedlings at the second (G2) and fourth generations. Telomeric profiles are presented for GH1-HMGA1 TERT G2 (lane 1), gh1-hmga1 TERT G2 (lane 2), GH1-HMGA1 tert G2 (lane 3), and gh1-hmga1 tert G2 (lane 4).
Overall, these observations clearly show a role of GH1-HMGA1 in telomere regulation, affecting both telomere protection and length regulation.
DISCUSSION
We observed an enrichment of GH1-HMGA1 in our telomere pull-down aiming to identify new telomeric regulators in Arabidopsis. GH1-HMGA1 belongs to the HMGA protein family in plants (Kotlinski et al., 2017). However, its domain architecture is distinct from that of human HMGA, as it consists of an additional N-terminal histone GH1 domain, likely providing additional chromatin-binding properties. Both the linker histone H1 and the HMG proteins are abundant, associate with chromatin, and are involved in many fundamental DNA-related processes: chromatin compaction, transcription, cell cycle progression, and DNA repair (Cleynen and Van de Ven, 2008; Over and Michaels, 2014; Reeves, 2015). HMG proteins compete with histone H1 for DNA binding (Catez and Hock, 2010; Reeves, 2010), and their binding promotes chromatin decompaction, allowing the regulation of chromatin accessibility in interplay with H1. Interestingly, all mammalian HMG proteins compete with H1 and members of a given HMG family compete with one another for chromatin-binding sites, but members of different HMG families do not cross-compete, indicating that there are distinct, family-specific chromatin-binding sites (Catez et al., 2004).
In Arabidopsis (and plants in general), very few studies have been performed to characterize the functional role of HMG proteins. Both maize and Arabidopsis HMGA are located in the nucleus (Launholt et al., 2006; Zhao et al., 2009), and fluorescence recovery after photobleaching studies with AtHMGA (GH1-HMGA3) have shown a fast-moving protein in plant cell nuclei like their mammalian counterparts. In contrast to mammalian HMGA1 and HMGA2, GH1-HMGA1 was relatively abundant and expressed ubiquitously in Arabidopsis (Figs. 1 and 2). In support of this, the absence of GH1-HMGA1 led to severe morphological defects associated with genomic instability, including telomeric deprotection and inefficient DNA repair.
Interestingly, while GH1-HMGA3 is not associated with condensed chromosomes in mitosis (Launholt et al., 2006), our work showed that its family member GH1-HMGA1 is present on mitotic chromosomes. Thus, the localization of GH1-HMGA1 seems to be more reminiscent of mammalian HMGA proteins that are abundantly present at metaphase chromosomes (Disney et al., 1989). The localization on specific DNA loci is another fundamental aspect of HMG proteins. In our study, even as GH1-HMGA1 binding seemed to be more widespread, we observed an exclusion from centromeres and an apparent enrichment at pericentromeric regions. More work has to be done to validate these results and to precisely define the exact localization of GH1-HMGA1 on chromatin.
This study and reports from the literature argue for an active role of these proteins in the DNA repair process, especially in HR, which may be conserved in animals and fungi. For instance, mammalian HMGA overexpression sensitizes cancer cell lines to UV light, cisplatin, and methyl-methanesulfonate. In our work, the expression of three major genes involved in DNA repair processes was not affected in gh1-hmga1 mutants. Additionally, in these mutants, early DNA damage repair appeared unaffected, but 1 h after irradiation treatment, the efficiency of repair was impaired greatly, favoring a transcription-independent role for GH1-HMGA1 in the process. In support of this idea, mammalian HMGA and GH1-HMGA1 are both phosphorylated by ATM kinase after DNA damage (Palmieri et al., 2011; Roitinger et al., 2015) and HMGA1a in wheat (Triticum aestivum) and mammals has a strong affinity for Holliday junctions (Hill and Reeves, 1997; Zhang et al., 2003). Furthermore, it was noted recently that HMGA2 is recruited directly to stalled replication forks and acts as a replication fork chaperone (Yu et al., 2014). All these observations suggest a direct role in the DNA damage repair process.
What could be the role of GH1-HMGA1 in DSB repair by HR? One suggestion comes from studies revealing that the amount of histone H1 in chromatin has a direct impact on HR efficiency, with the absence of H1 correlating with a greater ability to repair DSB in yeast and mice (Downs et al., 2003; Murga et al., 2007). In yeast, the absence of H1 promotes HR and makes telomeres more prone to recombination, increasing the number of survivors in the absence of functional telomerase (Downs et al., 2003). In accordance with this suggestion that higher order chromatin organization imposed by H1 inhibits HR, the histone chaperone NAP1 is recruited via RAD54 to DSB sites to remove H1, stimulating the chromatin-remodeling activity of RAD54 required for correct chromosome pairing during HR (Machida et al., 2014). Interestingly, it has been shown that Arabidopsis NAP1 is important for somatic HR (Gao et al., 2012). Thus, the presence of the GH1-HMGA1 protein might limit the amount of H1 on DNA at DSB sites, favoring HR repair. In the absence of GH1-HMGA1, higher amounts of H1 would impair chromatin remodeling by RAD54 and strand invasion by RAD51. Our immunocytological data showing an enrichment of H1 on chromatin in gh1-hmga1 agreed with this scenario.
The importance of a functional GH1-HMGA1 in telomere homeostasis was seen in the phenotypes of the mutant plants. The knockout mutant (gh1-hmga1-1) had pleiotropic growth defects accompanied by deprotected and degraded telomeres, showing a role of this protein in telomere stability. That only 4.3% of mutant plants were found in the progeny of selfed heterozygotes (25% was expected) and the severe developmental phenotype and sterility of the surviving mutant plants underline the importance of this role. So, what could be the function of GH1-HMGA1 at plant telomeres? Other HMG family proteins, such as plant and mammalian HMGB, are involved in telomere homeostasis (Schrumpfová et al., 2011; Polanská et al., 2012), and mammalian mutants for these proteins display TERT-independent decreased telomere length. Interestingly, in Arabidopsis, hmgb mutants have increased levels of telomeric repeats containing RNA (TERRA) transcripts, but a mechanistic link is still missing (Schrumpfová et al., 2011). Thus, although no genomic instability has yet been reported due to the absence of HMGBs in mammals, these proteins are clearly linked to telomere maintenance. Importantly, a recent report shows that the mammalian HMGA2 protein is implicated directly in telomere protection in cancer cells (Natarajan et al., 2016). This demonstrates that HMGA2 increases TRF2 retention at telomeres by preventing the phosphorylation of TRF2 (TRF2T188-p) by ATM and that decreased HMGA2 expression leads to TRF2 removal from telomeres and telomere instability.
Our work shows that GH1-HMGA1 is important for telomere length regulation and for telomere protection. The acceleration of telomeric loss in the double tert gh1-hmga1 mutants (Fig. 9) and the fact that the length of shortened telomeres of gh1-hmga1 mutant plants appears to stabilize (Fig. 8; Supplemental Fig. S7) indicate that GH1-HMGA1 acts independently of telomerase in telomere length homeostasis. Different, but not mutually exclusive, scenarios can be considered to explain the role of GH1-HMGA1 at telomeres. First, GH1-HMGA1 could be required for correct telomere replication, with its absence leading to replication defects and telomeric loss that are compensated by telomerase. Second, given its role in HR, GH1-HMGA1 could be required for telomere length regulation in a parallel pathway to telomerase. Such an alternative mechanism in telomere elongation has been suspected in Arabidopsis (Růčková et al., 2008); however, no molecular mechanism has been described. Finally, we speculate that, as in mammals, GH1-HMGA1 is important for telomere protection through stabilization of the plant shelterin complex. Although no such proteins have yet been described in plants, the TRB proteins are candidates to play a role in this mechanism (Schrumpfová et al., 2014), and we can hypothesize an interaction between TRBs and GH1-HMGA1, through their respective GH1 domains, forming a stable complex important for telomere homeostasis. As stated above, these hypotheses are not necessarily mutually exclusive, and more work will be required for full understanding of how GH1-HMGA1 participates in telomere length regulation and protection.
In conclusion, we have identified GH1-HMGA1 as a factor involved in DNA damage repair in Arabidopsis and propose that it acts by influencing chromatin organization via competition with linker histones. This DNA repair function seems to be widely conserved in eukaryotes. Affecting Arabidopsis telomere length regulation and telomere end protection, GH1-HMGA1 is thus a telomere regulator in plants. More work is required to elucidate the other roles of this multifunctional protein.
MATERIALS AND METHODS
Telomere Pull-Down
Telomere pull-downs were conducted as described (Kappei et al., 2017). Arabidopsis (Arabidopsis thaliana) whole-cell lysate was incubated for 2 h at 4°C under slight agitation in PBB buffer with immobilized (Dynabeads C1; Thermo) concatamerized, biotinylated oligonucleotides of either the Arabidopsis telomeric TTTAGGG repeat or its shuffled control sequence (GTGTATG). The beads were washed three times with PBB buffer and heated subsequently in 30 µL of 1× LDS buffer to 70°C (Thermo). The proteins were separated on a NuPage Novex 4% to 12% gradient gel. Each lane was minced, and mass spectrometry sample preparation was done according to the in-gel protocol (Shevchenko et al., 2006). Peptides were desalted and stored on StageTips prior to measurement.
Mass Spectrometry and Data Analysis
Peptides were analyzed by nanoflow HPLC on an EASY-nLC 1000 system (Thermo) coupled online to a Q Exactive Plus mass spectrometer (Thermo). Peptides were separated on a C18 reverse-phase capillary (25 cm long, 75 μm i.d., packed in house with ReproSil-Pur C18-AQ 1.9 μm resin; Dr Maisch). A 208-min gradient from 2% to 60% acetonitrile in 0.5% formic acid at a flow of 200 nL min−1 was used. The Q Exactive Plus was operated with a Top10 tandem mass spectrometry spectra acquisition method per mass spectrometry full scan. The raw files were processed with MaxQuant version 1.5.0.25 (Cox and Mann, 2008) against the Uniprot Arabidopsis database (33,382 entries). Carbamidomethylation was set as a fixed modification, while Met oxidation and protein N-acetylation were considered as variable modifications. The search was performed with an initial mass tolerance of 7 ppm mass accuracy for the precursor ion and 20 ppm for the tandem mass spectrometry spectra in the HCD fragmentation mode. Search results were processed with MaxQuant and filtered with a false discovery rate of 0.01. The match between run option and the LFQ quantitation were activated. Missing values were imputed by a downshifted, compressed normal distribution. Replicates were averaged, and their P values were calculated by Student’s t test. Plots were created using R.
Bioinformatics Analysis
Phylogeny
Amino acid sequences of HsHMGA1a (NP_665906.1), HsHMGA1b (NP_002122.1), HsHMGA1c (NP_001306009.1), HsHMGA2 (NP_003474.1), HsH1.1 (NP_005316.1), AtHMGA (GH1-HMGA3; NP_172943.1), AtHON4 (GH1-HMGA1; NP_188431.3), AtHON5 (GH1-HMGA2; NP_175295.1), AtH1.1 (NP_172161.1), AtH1.2 (NP_180620.1), and AtH1.3 (NP_179396.1) proteins were aligned with Clustal omega (www.ebi.ac.uk/tools/msa/clustalo/). The phylogenic tree was made using Mega7 software with the following settings: Maximum Likehood (StatisticalMethod), 1000 Bootstraps, Wag model, uniform rates, Complete Deletion (Gap/Missing Data Treatment), Nearest-Neighbor-Interchange (ML Heuristic Method).
Domain Analysis
The presence of conserved domains in GH1-HMGA1 amino acid sequences was determined using Motif Scan (http://myhits.isb-sib.ch/cgi-bin/motif_scan) and CD-search (https://www.ncbi.nlm.nih.gov/Structure/cdd/wrpsb.cgi).
Plant Material and Growth Conditions
Seeds were surface sterilized and sown on petri plates on 1× Murashige and Skoog medium including vitamins and MES buffer (#M0255; Duchefa Biochimie), plus 1% Suc (Duchefa Biochimie), solidified with 0.8% agar (Bacto-Agar; DIFCO Laboratories). Petri dishes were placed at 4°C for 48 h and transferred to a growth chamber (16 h of light and 8 h of dark) at 23°C.
GH1-HMGA1 T-DNA insertion lines (SAIL_215_D04 and SALK_099887C) were obtained from the Arabidopsis Biological Resource Center. The tert T-DNA line has been described previously (Fitzgerald, 1999). The double mutant gh1-hmga1 tert line was produced by crossing a TERT/tert heterozygote with a gh1-hmga1 homozygote using standard techniques.
Sensitivity Assay
For the MMC assay, medium was supplemented with 40 μm MMC (Sigma) and plants were then grown for 2 weeks. For γ-irradiation, 7-d-old seedlings were irradiated in a cabinet x-ray irradiator (X-RAD 320), returned to standard growth conditions, and grown further for 7 d. Sensitivity was then analyzed in 2-week-old seedlings by counting the number of true leaves as described previously (Bleuyard and White, 2004). Plants with more than three true leaves were considered resistant. For the hydroxyurea sensitivity assay, seeds were germinated on vertical plates containing medium supplemented with 0.5 mm hydroxyurea and allowed to grow for 10 d. Sensitivity was analyzed by measuring root length at day 10. In each case, the number of leaves was counted on at least 50 seedlings in three independent experiments.
Mutant Characterization
The GH1-HMGA1 gene identification locus is At3g18035. The gh1-hmga1-1 (SAIL_215_D04) mutant was genotyped using primers P1 and P2 to detect the wild-type loci and primers P1 and SAIL LB2 (SAIL T-DNA left border-specific primer) to detect the T-DNA insertion allele. For the gh1-hmga1-2 mutant (SALK_099887), genotyping was performed using primers P3 and P4 to detect the wild-type loci and primers P3 and Lba1 (SALK T-DNA left border-specific primer) to detect the T-DNA allele. Sequences of the primers used for genotyping are listed in Supplemental Table S1.
Reverse Transcription-PCR Analyses
For reverse transcription-PCR, total RNA was extracted from rosette leaves using Trizol Reagent (Thermo-Fisher Scientific) following the manufacturer’s instructions. After treatment with RQ1 RNase-free DNase (Promega) and reverse transcription using M-MLV reverse transcriptase (Promega) according to the manufacturer’s instructions, 80 ng was used for each PCR amplification. Primers used for PCR are indicated in the corresponding figures, and their sequences are listed in Supplemental Table S1.
Cloning and Transformation
For GFP-tagged lines, the DNA fragment encompassing the full GH1-HMGA1 genomic coding sequence (including introns) and 1 kb of upstream sequence was amplified by PCR (forward primer P5 and reverse primer P6; Supplemental Table S1) using Arabidopsis Columbia-0 wild-type genomic DNA as a template. The PCR product was inserted into pDONR (Invitrogen) and verified by sequencing. The complete genomic fragment was then transferred to the Gateway destination vector pB7FWG2, from which the promoter was removed with SacI/SpeI digestion. Eventually, the plasmid was transformed into Agrobacterium tumefaciens, which was used subsequently to transform Columbia-0 wild-type plants by the floral dip method (Clough and Bent, 1998). Seeds from the A. tumefaciens-treated plants were sown on soil, and transformants were selected for BASTA resistance. The presence of the constructs and the genotypes of BASTA-resistant plantlets were verified by PCR. Plants used in this study were selected as homozygous for the presence of the construct.
Immunostaining
Five days after germination, root tips were prepared as described previously (Amiard et al., 2014). Slides were incubated overnight at 4°C with 50 μL of primary antibody in fresh blocking buffer (3% BSA and 0.05% Tween 20 [Sigma] in 1× PBS), washed 3× 5 min in 1× PBS solution, and then incubated 2 to 3 h at room temperature in 50 μL of blocking buffer of secondary antibodies. Finally, slides were washed 3× 5 min in 1× PBS and mounted in Vectashield mounting medium with 1.5 μg mL−1 DAPI (Vector Laboratories). Antibodies and dilutions used in this study are reported in Supplemental Table S2.
Immuno-FISH
After fixation, leaves were chopped directly in the enzyme cocktail mix (1% cellulase, 1% pectolyase, and 0.5% cytohelicase in 1× PME) and incubated 15 min at room temperature. Nuclei suspensions were then transferred to poly-Lys-coated slides. One volume of 1% lipsol in 1× PME was added to the mixture and spread on the slide. Then, 1 volume of 4% PFA in 1× PME was mixed, and slides were dried at room temperature.
Immunodetection was conducted with an appropriate antibody coupled with biotin (Supplemental Table S2). Before the FISH step, slides were postfixed with a 4% PFA PBS solution. FISH was carried out as described previously (Vannier et al., 2009) using a telomeric probe labeled by PCR [(95°C 1’, 55°C 40’’, and 72°C 2’)*5 (94°C 1’, 60°C 40’’, and 72°C 2’)*25] with digoxigenin-11-dUTP using specific telomere primers (5′-TTTAGGG-3′). For the detection of the digoxigenin-labeled probe, mouse anti-digoxigenin (1:125) antibody followed by rabbit anti-mouse FITC (1:500) and goat Alexa 488 anti-rabbit IgG (1:100) antibodies were used. Finally, the target protein was detected with a tertiary fluorescent Cy3 anti-biotin (1:1,000) antibody (C5585; Sigma), and slides were mounted using Vectashield (Vector Laboratories) mounting medium containing 1.5 µg mL−1 DAPI.
DAPI Staining of Mitoses and Meiosis
Whole inflorescences were collected and squashed on a slide (Ross et al., 1996; Caryl et al., 2000). Slides were mounted using Vectashield (Vector Laboratories) mounting medium containing 1.5 µg mL−1 DAPI.
Microscopy
Images of immunostaining were acquired on the Zeiss Axioimager.Z1 microscope using ZEN software. Measurements and image enhancement were performed using the same software. Image stacks were captured in three dimensions (x, y, and z) and were deconvoluted with the deconvolution module (theoretical point spread function, iterative algorithm) of the ZEN software. For presentation, the images were collapsed Z-stack projections obtained using the extended-focus module (projection method) of the ZEN software.
For live microscopy and immuno-FISH experiments, acquisitions were performed on a structured illumination (pseudo-confocal) imaging system (ApoTome AxioImager M2; Zeiss) and were deconvoluted using the deconvolution module (regularized inverse filter algorithm). The fluorescence colocalization was analyzed via the colocalization module of the ZEN software using the uncollapsed Z-stack files.
Relative Fluorescence Analysis
Images of nuclei were acquired with DAPI (Set49) and DsRed (Set43HE) filters with 50 and 200 ms of exposure, respectively. The intensity of each channel was calculated using the intensity sum projections module of the ZEN software, and the ratio between DAPI and DsRed signal was calculated. This was performed on three independent experiments, calculating in each case the intensity of 25 S/G2 nuclei (75 nuclei in total).
TRF Analyses
TRF analysis of telomere length in HinfI-digested genomic DNA was conducted as described previously (Gallego and White, 2001).
Analyses of DNA Content with Flow Cytometry
Nuclei were prepared with the Cystain UV Precise P kit (Partec) according to the manufacturer’s protocol. Briefly, 20 1-week-old seedlings were chopped with a sharp razor blade in 200 mL of Cystain UV Precise P nuclei extraction buffer supplemented with 800 mL of Cystain UV Precise P staining buffer. Samples were filtered through a 30-μm nylon mesh, and supernatants were analyzed using an Attune Acoustic Focusing Cytometer (Life Technologies) following the manufacturer’s protocol. Results were analyzed using the Attune Cytometric Software version 1.2.5.
Histochemical Staining of GUS Expression
Fourteen-day-old seedlings grown under standard conditions were harvested and incubated in staining buffer (0.2% Triton X-100, 50 mm sodium phosphate buffer [pH 7.2], and 2 mm 5-bromo-4-chloro-3-indolyl-β-d-GlcA [Biosynth], dissolved in N,N-dimethyl formamide). Plants were vacuum infiltrated for 15 min and incubated at 37°C overnight. The staining solution was then replaced with 70% ethanol to remove leaf pigments, and the blue spots were counted with a binocular microscope.
Accession Numbers
Accession numbers are as follows: AtGH1-HMGA1, At3g18035; and AtTERT, AT5G16850.
Supplemental Data
The following supplemental materials are available.
Supplemental Figure S1. Phylogenetic tree of HMGA-like family members.
Supplemental Figure S2. Previous GH1-HMGA1 expression profiles.
Supplemental Figure S3. GH1-HMGA1 colocalization with telomeres.
Supplemental Figure S4. Intron-exon organization of GH1-HMGA1 and T-DNA insertion sites.
Supplemental Figure S5. Raw data used for Figure 6A.
Supplemental Figure S6. Semiquantitative transcriptomic analysis of DNA repair genes.
Supplemental Figure S7. Analysis of telomeric length over multiple generations.
Supplemental Figure S8. Phenotypic characterization of the tert gh1-hmga1 mutants.
Supplemental Table S1. Primers used in this study.
Supplemental Table S2. List of antibodies used in this study.
ACKNOWLEDGMENTS
We thank the members of the recombination group (particularly Maria E. Gallego) for their help and discussions. We thank Julio Saez Vazquez for providing the anti-H1 antibody, Peter Schlögelhofer for providing the anti-RAD51 antibody, and Steve Henikoff for providing the anti-CENH3 antibody. We thank Alina Bluhm and Nuria Casas-Vila for providing training to O.R.
LITERATURE CITED
Author notes
This work was supported by a grant from the Region Auvergne to S.A. (no. 105060), the Centre National de la Recherche Scientifique, the Université Clermont Auvergne, and the Institut National de la Santé et la Recherche Medicale. The work in the Butter group was supported by Rhineland Palatinate Forschungsschwerpunkt Gene Regulation in Evolution and Development (GeneRED) and Deutsche Forschungsgemeinschaft (BU 2996/1).
Current address: Department of Cellular Logistics, Am Fassberg 11, 37077 Goettingen, Germany.
Current address: Institut National de la Recherche Agronomique 5, Chemin de Beaulieu, 63000 Clermont-Ferrand, France.
Address correspondence to [email protected] and [email protected].
The author responsible for distribution of materials integral to the findings presented in this article in accordance with the policy described in the Instructions for Authors (www.plantphysiol.org) is: Simon Amiard ([email protected]).
C.I.W., F.B., and S.A. conceived and supervised the research; C.C., O.R., O.D.I., F.B., and S.A. performed experiments; S.A. and F.B. wrote the article with contributions from C.C. and C.I.W.