-
PDF
- Split View
-
Views
-
Cite
Cite
Lae-Hyeon Cho, Jinmi Yoon, Richa Pasriga, Gynheung An, Homodimerization of Ehd1 Is Required to Induce Flowering in Rice , Plant Physiology, Volume 170, Issue 4, April 2016, Pages 2159–2171, https://doi.org/10.1104/pp.15.01723
- Share Icon Share
Abstract
In plants, flowering time is elaborately controlled by various environment factors. Ultimately, florigens such as FLOWERING LOCUS T (FT) or FT-like molecules induce flowering. In rice (Oryza sativa), Early heading date 1 (Ehd1) is a major inducer of florigen gene expression. Although Ehd1 is highly homologous to the type-B response regulator (RR) family in the cytokinin signaling pathway, its precise molecular mechanism is not well understood. In this study, we showed that the C-terminal portion of the protein containing the GARP DNA-binding (G) domain can promote flowering when overexpressed. We also observed that the N-terminal portion of Ehd1, carrying the receiver (R) domain, delays flowering by inhibiting endogenous Ehd1 activity. Ehd1 protein forms a homomer via a 16-amino acid region in the inter domain between R and G. From the site-directed mutagenesis analyses, we demonstrated that phosphorylation of the Asp-63 residue within the R domain induces the homomerization of Ehd1, which is crucial for Ehd1 activity. A type-A RR, OsRR1, physically interacts with Ehd1 to form a heterodimer. In addition, OsRR1-overexpressing plants show a late-flowering phenotype. Based on these observations, we conclude that OsRR1 inhibits Ehd1 activity by binding to form an inactive complex.
Flowering is one of the most important biological processes in plant reproduction. One critical factor, timing, ensures that plants flower under favorable conditions and that sufficient time is allowed for seed development (Kim et al., 2009a). The molecular mechanisms of flowering have been extensively studied in the long-day (LD) plant Arabidopsis (Arabidopsis thaliana) and in short-day (SD) plant rice (Oryza sativa; Imaizumi and Kay, 2006; Tsuji et al., 2011). These plant systems share conserved mechanisms such as the GIGANTEA-CONSTANS-FLOWERING LOCUS T (FT) pathway (Park et al., 1999; Samach et al., 2000; Kojima et al., 2002; Hayama et al., 2003; Corbesier et al., 2007; Tamaki et al., 2007). However, several major flowering regulatory genes in rice are not present in Arabidopsis (Brambilla and Fornara, 2013; Lee and An, 2015).
Early heading date 1 (Ehd1) is a unique gene that induces the expression of Heading date 3a (Hd3a) and Rice Flowering locus T1 (RFT1), two florigens in rice (Doi et al., 2004; Zhao et al., 2015). Ehd1 merges various upstream flowering signals and acts as a signal integrator. CONSTANS-like (COL) proteins such as Heading date 1 (Hd1), Grain yield and heading date 7, and COL4 suppress Ehd1 expression under nonpermissive LD conditions (Yano et al., 2000; Xue et al., 2008; Lee et al., 2010). The APETALA 2 genes SUPERNUMERARY BRACT (SNB) and OsINDETERMINATE SPIKELET 1 also inhibit Ehd1 and delay flowering (Lee et al., 2014). Under permissive SD conditions, Hd1, OsINDETERMINATE 1, and OsMADS51 induce Ehd1 to enhance flowering (Kim et al., 2007; Matsubara et al., 2008; Park et al., 2008; Wu et al., 2008).
Ehd1 belongs to the response regulator (RR) protein family in cytokinin signaling pathway. Two types of RRs mediate cytokinin signaling. Type-A RRs are the negative components that turn off this signal by forming feedback regulatory loops (Hwang and Sheen, 2001; Hwang et al., 2002, 2012; To et al., 2004). This type of RR is thought to bind and inhibit type-B RRs, which are transcription activators (Kim, 2008). Whereas type-B RRs have a receiver (R) domain and GARP DNA-binding (G) domain, type-A RRs contain only the former (Makino et al., 2000; Hwang et al., 2002; Schaller et al., 2007). Cytokinin signaling is a sequential phosphorelay similar to bacterial two-component response systems. Both RR types are activated by phosphorylation of the middle Asp (D) in the conserved Asp-Asp-Lys (d-d-K) motif within the R domain (West and Stock, 2001; Hwang et al., 2002). This phosphorylation either increases or decreases the stability of RR proteins (To et al., 2007; Kim et al., 2012; Liang et al., 2012) and also induces the homomerization of RRs through conformational changes (Fiedler and Weiss, 1995; Birck et al., 1999; Da Re et al., 1999; Anand et al., 2000; Veerabagu et al., 2012). Cytokinin strongly induces type-A RR genes and stabilizes their proteins (Brandstatter and Kieber, 1998; D'Agostino et al., 2000; To et al., 2007; Tsai et al., 2012). By contrast, type-B RR genes are not induced by cytokinin, but their proteins have transcriptional activity that is enhanced by cytokinin-stimulated phosphorylation of the R domain (Hass et al., 2004; Kim et al., 2006; Veerabagu et al., 2012). In addition, the subcellular localization of RR proteins is not affected by phosphorylation (Sakai et al., 2000; Tsai et al., 2012).
Like other type-B RRs, Ehd1 has conserved DNA-binding G domain and R domain (Doi et al., 2004). However, phylogenetic analyses have revealed that Ehd1 is diversified from other RRs of that type, and its ortholog is found only in members of the grass family (Colasanti and Coneva, 2009; Murphy et al., 2011; Coelho et al., 2013). In addition, there has been no report that RR proteins are involved in flowering time regulation. Thus, Ehd1 appears to have evolved in monocot plants to function as a unique component in the flowering pathway.
Although Ehd1 and the florigen genes Hd3a and RFT1 have a similar diurnal rhythm with a morning peak, Ehd1 peaks just prior to both Hd3a and RFT1 (Doi et al., 2004; Ryu et al., 2009; Lee et al., 2010; Gao et al., 2013; Yang et al., 2013). This suggests that Ehd1 functions immediately upstream of those florigen genes. Ehd1 protein has DNA-binding ability and may bind to the promoter regions of Hd3a and RFT1 to activate those genes (Doi et al., 2004). In addition to transcriptional regulation, posttranslational modifications of Ehd1 play a critical role in promoting Hd3a and RFT1. Activity of Ehd1 protein is reduced under nonpermissive LD conditions and is repressed by OsPhyA (Itoh et al., 2010; Osugi et al., 2011; Itoh and Izawa, 2013). However, the precise molecular mechanism how Ehd1 regulates downstream genes is largely unknown.
Here, we report that the Asp residue in the d-d-k motif is needed for dimerization of Ehd1 and that this dimerization is essential for Ehd1 functioning. We also show that OsRR1, a type-A RR, suppresses flowering by interacting with Ehd1. Our results suggest that RR proteins have antagonistic roles in regulating flowering time in rice.
RESULTS
The C-Terminal Stretch of Ehd1 Can Activate Transcription and the GARP DNA-Binding Domain Has a Nuclear Localization Signal
Ehd1 protein has an R domain between the 11th and 123rd residues and a G domain between the 197th and 255th residues. An inter (I) domain is present between the R domain and the G domain, while a C-terminal stretch (C) is located behind the G domain (Fig. 1A). Our examination of transcriptional activation that utilized a yeast system indicated that Ehd1, fused to the GAL4 DNA binding domain, activates the reporter gene (Fig. 1B). Analyses of various truncated fragments of the protein also showed that all constructs containing that C region can activate the reporter gene (Fig. 1B). When the R or RI region is deleted, activation is reduced. However, the C region alone has greater activity when compared with the full-length protein, suggesting that the upstream region represses the effector domain of Ehd1. Therefore, our observations demonstrate that the C-terminal region of Ehd1 functions as a transcriptional activator, similar to that observed for other type-B RR proteins in Arabidopsis (Hwang et al., 2002; Grefen and Harter, 2004).
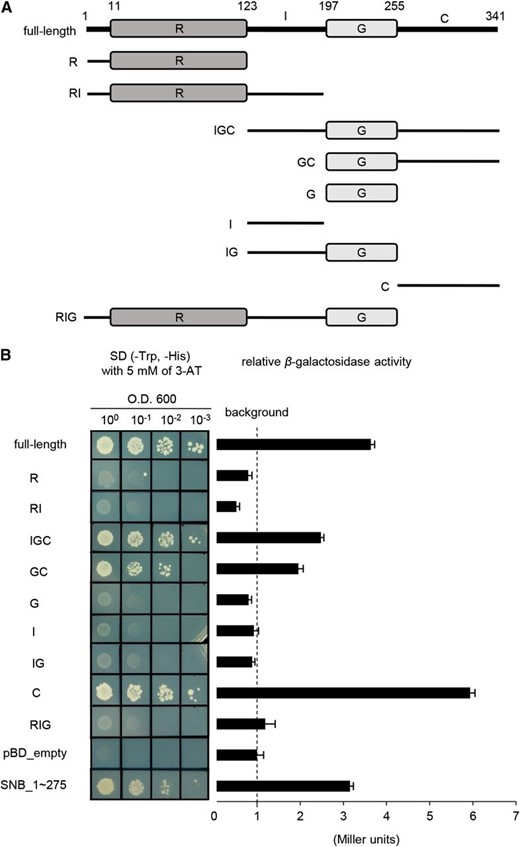
Analyses of transactivation of Ehd1. A, Schematic diagrams of Ehd1 and its truncated molecules used for assay of transactivation. Numbers represent positions of amino acids. R, receiver domain; I, inter domain; G, GARP DNA-binding domain; C, C-terminal region. B, Analyses of transactivation. Full-length or truncated Ehd1 cDNA was inserted into pBD_GAL4 Cam vector containing GAL4 DNA-binding domain, then transferred into yeast cells. Empty vector (pBD_empty) and SNB_1 ∼ 275 were used as negative and positive control, respectively. Values calculated for β-galactosidase activity are averages of three independent experiments. Bars represent standard deviations.
Analyses of the subcellular localization of Ehd1 using rice protoplasts suggested that the protein is found in the nucleus (Supplemental Fig. S1B). Of the four domains, only the G domain is localized to the nucleus (Supplemental Fig. S1, C–F).
The GARP Domain Region Induces Flowering, Whereas the N-Terminal Region Containing the Receiver Domain Represses Flowering
To continue our study of the molecular function of Ehd1 domains, we generated two deletion mutants. The first construct (IGC) lacked the N-terminal 123 residues (1st to 123rd) containing the R domain (Fig. 2A), while the second construct (RI) lacked the C-terminal 145 amino acid residues (197th-341st) that contain the G domain and C region (Fig. 2A). These were placed under the control of the maize (Zea mays) Ubiquitin (Ubi1) promoter and nos terminator in a binary vector, and the chimeric molecules were stably transferred into rice by Agrobacterium-mediated cocultivation. From the 20 independently transformed plants obtained for each construct, we selected two lines that highly expressed the introduced gene. The T1 progeny were grown under SD conditions and heading dates were recorded. The Ehd1 full-length overexpression (OX) plants flowered 17 to 18 d earlier than the wild type (Fig. 2, B and C). The Ehd1 OX plants also showed this phenotype under LD, flowering 40 ∼ 43 d earlier than the wild type (Supplemental Fig. S2). This observation is similar to one previously reporting that over-expression of Ehd1 induces flowering (Osugi et al., 2011).
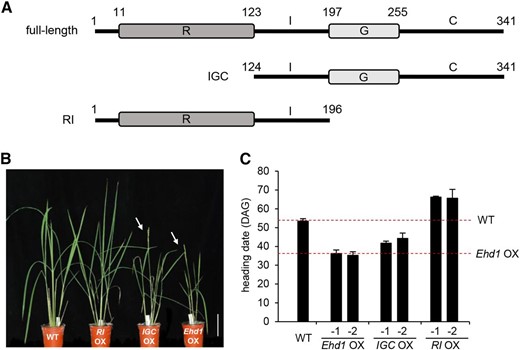
Phenotypes of transgenic plants over-expressing full-length or truncated Ehd1. A, Schematic diagrams of full-length and truncated forms of Ehd1 used for generation of transgenic plants. Top, full-length protein; middle, truncated Ehd1 with IGC domain; bottom, truncated Ehd1 with RI domain. B, Phenotypes of transgenic plants over-expressing full-length Ehd1 (Ehd1 OX) and truncated Ehd1 (RI OX and IGC OX) grown under SD conditions. Arrows indicate bolted panicles. Scale bar = 10 cm. C, Heading dates under SD conditions. Two independently transformed plants that expressed introduced gene at high levels were analyzed. Date were recorded when first panicle bolted. Heading dates of wild-type plants and transgenics over-expressing full-length Ehd1 are indicated as dashed lines. Error bars show standard deviations. n = 8 or more. WT, wild type.
We found it interesting that the IGC OX plants also flowered early when compared with the wild type (Fig. 2, B and C). This indicated that the R domain is not required for Ehd1 to function in inducing flowering. Although the OX plants flowered earlier than the wild type, they flowered later than the full-length Ehd1-OX plants. This was probably due to a reduction in transactivation when the R domain was deleted (Fig. 1B). By contrast, RI OX plants, generated via the second construct, flowered 12 ∼ 13 d later than the wild type (Fig. 2, B and C), thereby indicating that the N-terminal region containing the R domain acts as a flowering repressor.
Ehd1 Forms a Homomer through the Inter Domain
Because RR forms homomers or heterodimers (Dortay et al., 2006; Veerabagu et al., 2012), we proposed that the RI fragment might bind to intrinsic Ehd1 to form an inactive heterodimer that led to the delayed flowering phenotype. To investigate the possibility of protein dimerization, we performed coimmunoprecipitation (co-IP) analyses. We first examined whether the Ehd1 full-length protein forms a homomer. Ehd1 fused to the HA tag (Ehd1-HA) and Ehd1 fused to the Myc tag (Ehd1-Myc) were coexpressed in rice root-derived callus suspension (Oc) cell protoplasts. After immunoprecipitation by anti-Myc antibodies, protein interactions were detected with anti-HA antibodies. This experiment indicated that Ehd1 protein forms a homomer (Fig. 3A). We also observed that the RI and IGC fragments form homodimers (Fig. 3, B and C). Finally, we examined potential dimerization between RI and the full-length protein. As we had expected, RI-HA interacted with the full-length Ehd1-Myc, and IGC-Myc also interacted with the full-length Ehd1-HA (Fig. 3C). We confirmed this homomerization of the RI fragment by yeast two-hybrid (Y2H) assays (Fig. 3E) and bimolecular fluorescence complementation (BiFC) experiments (Supplemental Fig. S3).
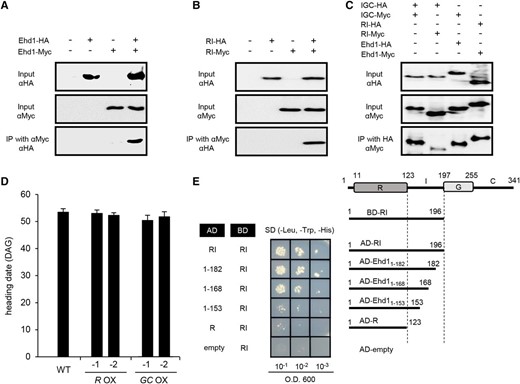
Interaction analyses of Ehd1 and truncated Ehd1. A, Homerization analysis of Ehd1. coIP analyses performed between Ehd1-HA and Ehd-Myc. B, Homerization analysis of truncated Ehd1 containing R and I regions. coIP analyses were performed between Ehd1 RI fragment tagged with HA (RI-HA) and Ehd1 RI fragment tagged with Myc (RI-Myc). C, Interaction analyses among full-length Ehd1 and truncated Ehd1. Truncated Ehd1 containing I, G, and C regions was tagged with HA (IGC-HA) or Myc (IGC-Myc). Homomerization of fragment as well as interaction with RI fragment or full-length Ehd1 were examined. Tagged proteins were expressed in rice suspension cells after transformation of tagged constructs. Expressed proteins were immunoprecipitated with anti-Myc antibodies or anti-HA antibodies, then separated by sodium dodecyl sulfate polyacrylamide gel electrophoresis (SDS-PAGE). Signals were detected by HRP-conjugated anti-HA antibodies or anti-Myc antibodies. Inclusion or exclusion of construct is shown by plus or minus. D, Heading date of SD-grown transgenic plants over-expressing truncated Ehd1 with R domain (R OX) or GC domain (GC OX). Error bars indicate standard deviations. n = 8 or more. Scale bar = 10 cm. WT, wild type. E, Y2H assays of interaction between Ehd1 RI fragment (BD-RI) with deletion derivatives of I region. AD-empty and BD-RI was used as a negative control.
To identify the region responsible for the dimerization, we generated additional constructs and examined their interactions by Y2H. In particular, we investigated whether the I region is needed for dimerization by testing the interaction between the RI fragment (aa 1–196) and the R domain (aa 1–123) alone. This analysis showed that R does not bind to RI (Fig. 3E). We confirmed this result by BiFC experiments (Supplemental Fig. S3), which suggested that the I region (aa 124-196) is necessary for dimerization. Furthermore, removal of 14 aa (183–196) or 28 aa (169–196) of the I region did not decrease this interaction with RI (Fig. 3E; Supplemental Fig. S3). However, a further deletion (aa 154–196) led to significantly diminished interactions, thereby implying that the 15-aa region between the 154th and 168th positions is involved in this dimerization.
We confirmed these results with the IGC fragment (aa 124–341) and found that it binds to RI, based on BiFC analysis (Supplemental Fig. S3). This outcome confirmed our conclusions from the co-IP experiment (Fig. 3C). However, the IGC fragment did not interact with GC (aa 197–341), confirming that the I region is required for dimerization (Supplemental Fig. S3). Removing 30 aa (124–153) from the IGC fragment did not reduce its ability to bind to IGC. However, deletion of an additional 15 aa (154–168) or 29 aa (154–182) resulted in a significant loss of binding ability (Supplemental Fig. S3). These findings supported our conclusion that the region between the 154th and 168th positions within the I region is needed for dimerization.
We then monitored flowering time in deletion mutants, using transgenic plants containing those fragments. Plants expressing the R region flowered at 52 to 53 d after germination (DAG), which was nearly the same as when flowering was recorded for the wild type (Fig. 3D). We also observed that this timing was not influenced in plants expressing the GC region (Fig. 3D). Transcripts levels of Hd3a and RFT1 were not significantly changed in these transgenic plants when compared with the wild type (Supplemental Fig. S4). This was evidence that R alone or the GC region do not affect flowering time, probably because the capacity for dimerization is lacking for the intrinsic Ehd1.
Phosphorylation of Asp-63 Is Essential for Ehd1 Activity
The R domain of Ehd1 contains the conserved Asp-Asp-Lys (d-d-K) residues found in all RR proteins, while the middle Asp at the 63rd residue (Asp-63) is a putative site for phosphorylation (Supplemental Fig. S5; Hwang and Sheen, 2001; Hwang et al., 2002). In bacteria, transcriptional activity by RRs depends upon phosphorylation of the R domain (Lewis et al., 2002; Gao et al., 2007). Similarly, R-domain phosphorylation is crucial for activating RR proteins in Arabidopsis and rice (Hwang and Sheen, 2001; Hass et al., 2004; Hirose et al., 2007).
Direct analyses of phosphorylated Asp are difficult to conduct, because this acylphosphate bond is easily hydrolyzed and is both acid and base labile (Koshland, 1952). Moreover, no antibodies toward phospho-Asp are commercially available. However, those problems can be overcome through mutational approaches. While the Asp to Glu (d-to-E) substitution mimics a constitutive phosphorylated state, the Asp to Asn (d-to-N) substitution partially inactivates that state (Hwang and Sheen, 2001; Hass et al., 2004; Kim et al., 2006; Lee et al., 2008). Here, we replaced Asp at the 63rd residue of Ehd1 with either Glu (D63E) or Asn (D63N; Fig. 4A). Transgenic rice plants expressing this mutated Ehd1 were generated, and their flowering time was compared with that of plants overexpressing the wild-type Ehd1. We were interested to learn that the d-to-E substitution caused extremely early flowering, i.e. 3 d earlier under SD and 21 d earlier under LD when compared with the wild-type Ehd1 OX plants (Fig. 4, B–D). In contrast, the d-to-N plants flowered later than wild-type Ehd1 OX plants, being delayed by 11 d under SD and 10 d under LD. Levels of transcripts for the florigen genes Hd3a and RFT1 were higher in the D63E plants and lower in D63N plants when compared with Ehd1-wild-type OX plants (Fig. 4, E and F). These observations indicated that the Asp-63 in Ehd1 plays an important role in controlling flowering time. Because the constitutively active form, D63E, is more active than the parent Ehd1 while the partially active form, D63N, has less activity, phosphorylation of the Asp appears to be necessary for Ehd1 function. Although the D63N plants flowered later than wild-type Ehd1 OX plants, the former flowered earlier than plants without exogenous Ehd1 expression. Florigen gene expression was enhanced in the D63N plants, whereas those genes were not expressed in the wild type (Fig. 4, E and F). This suggested that the d-to-N substitution did not completely inactivate Ehd1 functioning.
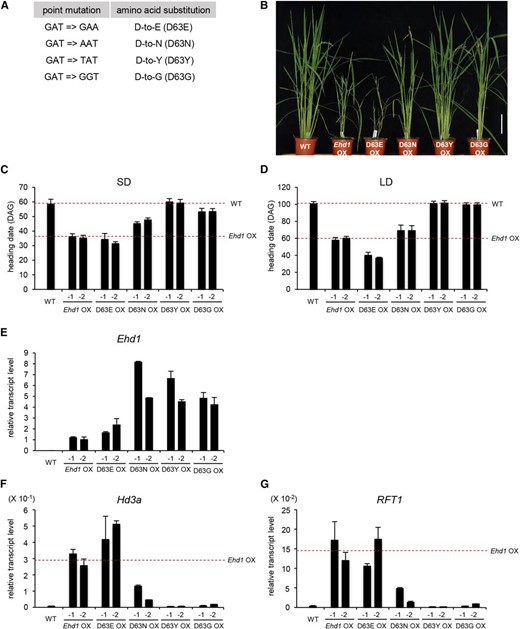
Flowering phenotypes of transgenic plants over-expressing Asp-63 mutants. A, Point mutations and aa substitutions in 63rd Asp residue of Ehd1. B, Phenotypes of SD-grown transgenic plants expressing mutated Ehd1. Bar = 10 cm. C and D, Heading date of transgenic plants expressing mutated Ehd1 grown under SD (C) or LD (D) conditions. Two independently transformed plants that expressed introduced gene wild-type plants and transgenics over-expressing wild-type Ehd1 are indicated as dashed lines. Error bars show standard deviations. n = 6 or more. E and G, Quantitative real-time PCR analyses of Ehd1 (E), Hd3a (F), and RFT1 (G) expression in plants over-expressing mutated Ehd1. Leaf blades were harvested at ZT 4 h from 21-DAG plants grown under SD conditions. The y axis shows the relative transcript level compared with rice Ubi1 expression. Transcript levels for florigen genes in transgenic plants over-expressing wild-type Ehd1 are indicated as dashed lines. Scale bar = 10 cm. Error bars show standard deviations. n = 4 or more.
We generated additional Ehd1 mutants by substituting the 63rd Asp with either Tyr (D63Y) or Gly (D63G). Flowering was not accelerated in transgenic rice plants expressing D63Y Ehd1 or D63G Ehd1 and they instead flowered at the same time as the wild type, thereby suggesting that those substitutions made the Ehd1 protein into a completely inactive form. Expression of Hd3a and RFT1 was not increased in those plants (Fig. 4, E and F), further supporting our conclusion that phosphorylation of the Asp at the 63th residue is essential for Ehd1 functioning to induce florigen genes.
Phosphorylation of Asp at the 63rd Residue Is Required for Homomerization of Ehd1
Homomerization of RRs depends upon the middle Asp being phosphorylated in the conserved d-d-K motif (Fiedler and Weiss, 1995; Da Re et al., 1999; Perron-Savard et al., 2005; Veerabagu et al., 2012). Therefore, we tested the homomerization capability of Ehd1 mutants by Y2H assays (Fig. 5). Because the C-terminal region of Ehd1 is self-activated, we used RI fragments. Those fragments with the D63E substitution formed a homodimer, as observed from the wild-type RI. Based on measurements of β-galactosidase activity, binding strength was greater in the D63E fragment than in the wild-type counterpart. In contrast, the RI fragment with the D63N substitution showed a weak interaction, and neither the D63Y nor the D63G substitution formed a homodimer. These results suggested that mutations of the Asp-63 affect the dimerization of Ehd1, possibly by structural changes.
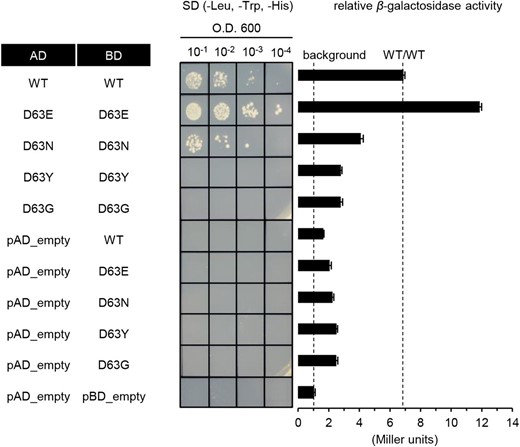
Y2H assays for homomerization of mutated Ehd1. Homomerization analyses by Y2H experiments. Wild-type Ehd1 and Asp-63 mutants were inserted into pGAD424 or pBD_GAL4 cam vector, and their interaction was analyzed by testing growth on minimal medium. Transformants were grown in liquid medium without Leu and Trp to achieve optical density A600 = 1. Afterward, 10 μL of undiluted or diluted yeast cell suspension was dropped onto minimal medium plates supplemented with 5 mm 3-amino-1,2,4-triazole but without Leu, Trp, and His, and held for 3 d. Values for relative β-galactosidase activity are averages from 3 independent assays. Error bars indicate standard deviations.
We performed BiFC experiments to confirm this capacity for dimerization. Mutants or wild-type Ehd1 were fused to the N terminus or C terminus of Venus. These fusion molecules were then coexpressed in rice Oc cell protoplasts, and their interactions were monitored under a fluorescence microscope (Supplemental Fig. S6). As observed from our Y2H assays, the D63E substitution showed the strongest florescence, while the D63N substitution displayed weak signals. Neither D63Y nor D63G produced any signal above the background level.
To test whether homomerization of Ehd1 is phosphorylation dependent, we carried out co-IP experiments after calf intestinal alkaline phosphatase (CIP) treatment. When the CIP was treated before immuno-precipitation, the homomerization capacity of Ehd1 was greatly reduced (Fig. 6). This indicated that homomerization of Ehd1 is dependent on phosphorylation levels.
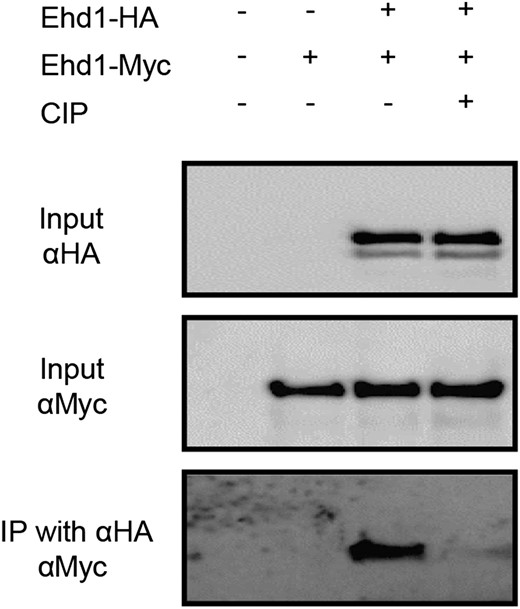
Phosphorylation-dependent homomerization of Ehd1. co-IP analyses were performed between Ehd1-HA and Ehd1-Myc after CIP treatment. Vectors were transformed into protoplasts prepared from Oc cells. Before immunoprecipitation with anti-HA antibodies, the protein extracts were incubated with or without CIP for 2 h at 37°C. Immunoprecipitated proteins were separated by SDS-PAGE, and signals were detected by HRP-conjugated anti-Myc antibodies.
Therefore, the results of our in planta experiments supported our conclusion that homomerization of Ehd1 is dependent upon phosphorylation of Asp-63.
Ehd1 Interacts with OsRR1, a Type-A RR
We showed that the RI fragment of Ehd1 delays flowering by binding to Ehd1. The RI is similar to type-A RRs that have an R domain but lack a G domain and C-terminal stretch. Because such RRs act as negative regulators (Hwang and Sheen, 2001; To et al., 2004; Hwang et al., 2012), we suspected that there is a type-A RR that binds to Ehd1 and inhibits flowering. Here, we conducted co-IP experiments to investigate the interaction between Ehd1 and rice type-A RRs and found that Ehd1 binds specifically to OsRR1 (Fig. 7A). This specific interaction was confirmed by BiFC analysis. Strong florescence was observed in the nucleus of Oc cell protoplasts when NV-OsRR1 and CV-Ehd1 constructs were cotransformed (Supplemental Fig. S7).

Characterization of OsRR1. A, Interaction analyses between Ehd1 and type-A RRs of rice by co-IP experiments. Full-length cDNAs of Ehd1 and rice type-A RRs were tagged with Myc and placed under control of Ubi1 promoter. Constructs, together with Ehd1-HA tag molecule, were introduced into protoplasts prepared from Oc cells, and expressed proteins were immuno-precipitated with anti-HA antibody. After proteins were separated via SDS-PAGE, signals were detected by HRP-conjugated anti-Myc antibody. B, Levels of OsRR1 transcripts in over-expressing plants. Two independently transformed plants that expressed introduced gene at high levels were analyzed. C, Heading dates for OsRR1 OX transgenic plants and wild-type controls grown under SD conditions. Bar = 10 cm. Error bars indicate standard deviations. n = 6 or more.
To obtain further evidence that OsRR1 is involved in controlling flowering time, we generated transgenic plants over-expressing OsRR1 (Fig. 7B). From the 15 independently transformed plants, we selected two lines that highly expressed the introduced gene. Examination of their T1 progeny showed that they flowered 8 to 13 d later than the wild type (Fig. 6C). The extent of this delayed-flowering phenotype was similar to that of plants over-expressing the RI fragment (Fig. 2C).These findings suggested that OsRR1 might inhibit Ehd1 by forming an inactive heterodimer that causes late flowering (Fig. 8).
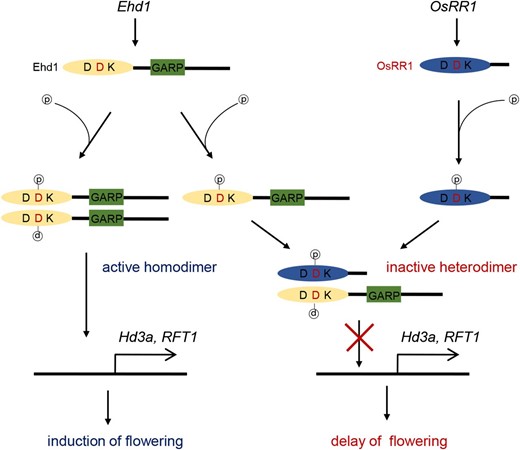
A putative model for flowering inhibition by OsRR1. Ehd1 is expressed during period of flowering induction. Ehd1 protein is activated by phosphorylation of middle D in R domain. Phosphorylation induces dimerization of Ehd1 by structural changes, which stimulates transcription of target genes Hd3a and RFT1. OsRR1 binds to Ehd1 to interfere with homomerization of Ehd1. This results in suppression of target gene expression, thereby delaying flowering.
DISCUSSION
Ehd1 Functions As a Dimer
The comparison of amino acid sequences among rice and Arabidopsis type-B RRs showed that the R and G domains are highly conserved, while the I and C-terminal regions are variable. The average lengths of such RRs of rice and Arabidopsis are 567 and 563 residues, respectively. However, Ehd1 is only 341 residues long. The extra 200 aa present in those other type-B RRs might mean that they have additional functions not associated with Ehd1. Nevertheless, although its C-terminal region is shorter, Ehd1 has transactivation ability in yeast, suggesting that Ehd1 is also a transcriptional activator like the others (Sakai et al., 2000; Kim et al., 2006; Veerabagu et al., 2012).
Arabidopsis has two type-B RRs—ARR1 and ARR2—for which their transactivation ability is inhibited by the R domain (Sakai et al., 2000). Transgenic plants over-expressing ARR1 that lacks the R region show severe phenotypes when compared with plants that over-express the full-length gene (Sakai et al., 2001). This suggests that the R domain has a repressive function. We found here that deletion of the R domain as well as the I and G regions, i.e. leaving only the C-terminal region, led to increased transactivation, whereas removal of either the R or RI region reduced that activity. Our findings implied, therefore, that the G region blocks transactivation but the R domain improves that ability.
We showed that over-expression of Ehd1 enhanced flowering, and deletion of the R region did not lose the enhancing ability. This is similar to previous observations that ARR1 is functional without the R domain in Arabidopsis (Sakai et al., 2001; Imamura et al., 2003). Therefore, the R region is not needed for protein functioning. When the I region was removed in our experiments, however, the ability of Ehd1 was diminished. Thus, because the I region is involved in homomerization of the protein, it appears that Ehd1 functions as a homodimer.
We also demonstrated that the N-terminal fragment containing R and I regions repressed flowering but that R-alone did not inhibit that process. This indicated that dimerization of the protein is necessary for such inhibition. Imamura et al. (2003) have reported that Arabidopsis plants over-expressing the R domain of ARR11 develop normally. Because the construct used in that study did not include most of the I region, this lack of inhibitory functioning was probably due to its inability to form a dimer. Again, we could postulate that the RI fragment forms a dimer with endogenous Ehd1, thereby blocking the formation of an Ehd1 homodimer.
From site-directed mutagenesis, we obtained evidence that the 63rd Asp residue in the R domain is the phosphorylation site and that this phosphorylation is crucial for activating Ehd1. We also obtained data that could be used to demonstrate that phosphorylation of the Asp residue is required for homomerization of Ehd1 and that the phosphorylated homomer is an active state of this protein. The phosphorylation may induce conformational changes of Ehd1 and expose the I region to enhance homomerization. These results are similar to those described for ARR18, an Arabidopsis type-B RR that forms a homomer in a phosphorylation-dependent manner (Veerabagu et al., 2012). In bacteria, homomerization of RRs is also mediated by the phosphorylation of R domains (Da Re et al., 1999; Lewis et al., 2002).
OsRR1 Delays Flowering by Forming a Heterodimer with Ehd1
Although homomerization of type-B RRs and physical interactions among different type-B RRs have been described (Dortay et al., 2006; Veerabagu et al., 2012), no heteromeric interaction has been reported between type-B and type-A RRs. Although the latter are considered negative regulators of the cytokinin response, the mechanism by which type-A inhibits type-B has not been fully elucidated, especially at the protein level (Pacifici et al., 2015). Although no direct experimental evidence has been revealed, researchers have proposed that type-A RR proteins may inhibit the activity of type-B RR proteins either by competing for phosphorylation from upstream His-containing phosphotransferases or by forming inactive type-A/B heterodimers (Kim, 2008; Kieber and Schaller, 2014).
In our current study, we showed that the type-A RR OsRR1 binds to the type-B RR Ehd1. In addition, we noted that over-expression of OsRR1 caused late flowering, suggesting that OsRR1 functions antagonistically to Ehd1. Based on that, we developed a putative model proposing that type-A RRs may inhibit type-B RRs through direct binding to form an inactive dimer. Further study is needed to confirm the interaction. For example, generation of OsRR1 OX transgenic plants in ehd1 will clarify roles of the gene in controlling flowering time.
OsRR1 is most highly expressed in roots, mature leaves, and flowers and is induced by cytokinin (Ito and Kurata, 2006; Jain et al., 2006). Transgenic plants over-expressing OsRR1 produce a higher frequency of crown root initiation in rice (Kitomi et al., 2011). These reports together with our results indicate that OsRR1 has multiple functions.
Components of the Cytokinin Signaling Pathway Are Involved in Flowering Time Regulation in Rice
Among their diverse roles, cytokinins are involved in the floral transition (Müller and Sheen, 2007; Argueso et al., 2009, Perilli et al., 2010; Turnbull, 2011; Hwang et al., 2012). However, the molecular mechanism by which they regulate flowering time is largely unknown. In Arabidopsis, cytokinin promotes flowering by direct action in the shoot apical meristem through transcriptional activation of TWIN SISTER OF FT (D’Aloia et al., 2011). In Sinapis alba, cytokinin induces mitotic activation in the shoot apical meristem during the floral transition (Bernier et al., 1977). Classical experiments suggest that the role of cytokinin in flowering is complicated (Kinet et al., 1993). Although cytokinin is needed for normal seedling development, premature flowering must be blocked in young plants. Therefore, cytokinin does not simply induce flowering. Instead, its functions might vary by tissue type and developmental stage for individual plants. To elucidate the regulatory role of cytokinin, the flowering phenotype of various cytokinin biosynthesis or signaling mutants is necessary. For example, the rice cytokinin oxidase/dehydrogenase4 activation mutant flowers later than the wild type (Gao et al., 2014). Other investigations are required to measure of the contents of active cytokinins both spatially and temporally.
Cytokinin responses are mediated by RR proteins. The rice genome encodes 13 type-A RRs and 13 type-B RRs (Schaller et al., 2007). However, the role of RR proteins in flowering time control is still largely unknown. In this study, we determined that Ehd1, a typical type-B RR, functions in the flowering of rice. Ehd1 orthologs are found only in members of the grass family, implying that Ehd1 has evolved to regulate flowering time only in such plants (Colasanti and Coneva, 2009; Murphy et al., 2011; Coelho et al., 2013). In addition, we examined the role of OsRR1, a component of the cytokinin signaling pathway that influences flowering time. Our findings provide new insight into how the flowering signal and cytokinin functioning have become diversified in higher plants.
MATERIALS AND METHODS
Plant Materials and Growth Conditions
Japonica rice (Oryza saliva cv Dongjin) plants were raised in controlled growth rooms under either SD conditions (12 h light at 28°C/12 h darkness at 22°C, humidity 50%) or LD conditions (14.5 h light at 28°C/9.5 h darkness at 22°C, humidity 50%). Seeds were germinated either on a 1/2 Murashige and Skoog medium containing 3% Suc or in soil as previously reported (Yi and An, 2013). We selected two T0 lines that expressed the introduced genes highly. T1 progeny were grown on 1/2 Murashige and Skoog medium containing 50 μg mL−1 hygromycin. Because all the progeny showed similar flowering-time phenotypes, we investigated heading dates of the transgenic plants without determining copy numbers. For RNA isolations, leaf blades were harvested at 21 or 28 DAG from SD plants and at 49 or 56 DAG from LD plants.
Vector Construction and Rice Transformation
The full-length Ehd1 cDNA, deletion mutants of the cDNA, and type-A RR cDNA clones were isolated by PCR using the primer sets listed in Supplemental Table S1. Point-mutant forms of Ehd1 were generated via site-directed mutagenesis by the overlap extension PCR method (Urban et al., 1997). The PCR products were cloned into binary vector pGA3428 or pGA3438, which contained the maize (Zea mays) ubiquitin 1 promoter, nopaline synthase (nos) terminator, and BsrGI and SpeI sites (Kim et al., 2009b). The Ehd1 promoter–GUS vector was constructed by inserting the 2.4-kb promoter region (−2,444 ∼ −4 bp from ATG start codon) into the pGA3519 binary vector, using HpaI and BamHI. Likewise, the Hd3a promoter–GUS vector was constructed by inserting the 2.2-kb promoter region (−2,226 ∼ +153 bp from ATG start codon) into the binary vector using KpnI and BamHI. After these constructs were transformed into Agrobacterium tumefaciens LBA4404 (An et al., 1989), transgenic rice plants were generated through stable transformation of the transfer DNA via Agrobacterium-mediated cocultivation, as previously reported (Lee et al., 1999).
RNA Isolation and RT-PCR and Quantitative RT-PCR Analyses
Total RNA was isolated from the leaf blades using RNAiso Plus (TaKaRa, Shiga, Japan). The first cDNA was synthesized with 2 μg of total RNA, using Moloney murine leukemia virus reverse transcriptase (Promega, Madison, WI) with 10 ng of the oligo(dT)18 primer and 2.5 mm deoxyribonucleotide triphosphate. Synthesized cDNAs were used as templates for quantitative real-time RT-PCR with SYBR Premix Ex Taq II (TaKaRa) and the Rotor-Gene 6000 instrument system (Corbett Research, Sydney, Australia). Rice Ubi1 was used as an internal control. All experiments were conducted at least three times, with four or more samples taken at each point. To ensure primer specificity, we performed the experiments when the melting curve showed a single sharp peak. PCR products were sequenced to verify the specificity of the reaction (Ryu et al., 2009; Yang et al., 2013; Yoon et al., 2014). All primers used for studying gene expression are listed in Supplemental Table S1.
Protoplast Isolation and Transformation
Protoplasts were isolated from Oc cells as described previously (Choi et al., 2014) but with minor modifications. Briefly, the Oc suspension solution was collected by centrifugation and the supernatant was removed. The cells were then incubated in a solution (2% cellulose RS, 1% macerozyme, 0.4 m mannitol, 0.1% MES, pH 5.7, and 0.1% CaCl2) for 4 h with gentle shaking. After an equal volume of a solution (117 mm potassium chloride, 82 mm magnesium chloride, and 85 mm calcium chloride) was added and the cells were harvested, their protoplasts were resuspended in a solution (0.4 m mannitol, 70 mm potassium chloride, 5 mm magnesium chloride, and 0.1% MES, pH 5.7) to achieve a density of 3 ×106 cells mL−1, as quantified with a hemocytometer. Isolated protoplasts were electroporated with 20 μg of plasmid DNA, utilizing a Gene Pulser Xcell (Bio-Rad, Hercules, CA) at 300 V and 450 mF (Ryu et al., 2009). The transfected protoplasts were incubated overnight at 28°C in the dark to allow for protein expression.
co-IP Assays
Myc-tag vector pGA3697 was constructed using the maize Ubi1 promoter and 6× Myc coding region. Restriction enzyme sites HpaI and KpnI were placed behind the Ubi1 promoter so that an inserted coding sequence could be fused to the Myc tag. The HA-tag vector pGA3698, carrying 3× HA, was similarly constructed. Full-length or truncated Ehd1 cDNA and type-A RR full-length cDNA were amplified without the stop codon using the primer sets listed in Supplemental Table S1. Amplified cDNA was inserted into the tag vectors and transferred into the Oc cell protoplasts. After incubation for 14 h at 28°C, the protoplasts were harvested and used for co-IP analysis as previously reported (Ryu et al., 2009; Choi et al., 2014). Briefly, the protoplasts were resuspended in Immunoprecipitation (IP) IP buffer (75 mm NaCl, 50 mm Tris-HCl, pH 7.5, 5 mm EDTA, 1% Triton X-100, 1 mm dithiothreitol (DTT), 1 mm phenylmethanesulfonylfluoride, 2 mm NaF, 20 μM MG132, and an adequate amount of Protease inhibitor cocktail; Roche, Mannheim, Germany). For CIP (New England Biolabs, Ipswich, MA) treatment, protein extracts were incubated in another IP buffer (100 mm NaCl, 100 mm MgCl2, 50 mm Tris-HCl, pH 7.5, 1 mm EDTA, 1% Triton X-100, 1 mm DTT, 5 mm phenylmethanesulfonylfluoride, 5 mm NaF, 40 μM MG132, and an adequate amount of Protease inhibitor cocktail) for 2 h at 37°C. Following brief vortexing, the samples were centrifuged for 5 min at 12,000 rpm. Afterward, 10 μL each of proteins A and G, conjugated to agarose beads (Millipore, Billerica, MA), were added to the supernatant and incubated for 1 h to prevent nonspecific binding. Approximately 10% of the precleared extract was saved as an input control while the remainder was incubated for 6 h with either an anti-HA mouse monoclonal antibody (12CA5; Roche) or an anti-Myc antibody (#2276; Cell Signaling, Beverly, MA) that was combined with 10 μL each of protein A- and G-conjugated agarose beads. After washing five times with buffer (75 mm KCl, 50 mm Tris-HCl, pH 7.5, 5 mm EDTA, 0.1% Triton X-100, 1 mm DTT, and 1mM phenylmethanesulfonylfluoride), the proteins bound to the beads were eluted with 20 μL of the IP buffer. The elutes were electrophoresed on a 10% SDS-polyacrylamide gel, then transferred to a polyvinylidene difluoride membrane (Millipore). The blot was incubated in a solution containing either horseradish peroxidase (HRP)-conjugated anti-Myc monoclonal antibody (#2040; Cell Signaling) or anti-HA monoclonal antibody (#2999; Cell Signaling). The bound proteins were observed by using an ECL prime western-blotting detection reagent (RPN2232; GE Healthcare, Buckinghamshire, UK) in LAS-4000 (GE Healthcare).
Subcellular Localization of Ehd1
Full-length or truncated fragments of Ehd1 cDNA were inserted into the pGA3452 vector that contained the maize Ubi1 promoter, synthetic GFP coding region, and the nos terminator, through SmaI and SpeI sites (Kim et al., 2009b). The NLS-mRFP driven by the 35S promoter was used as a nuclear marker. The constructs were cotransformed into protoplasts isolated from Oc cells by electroporation, and the transformants were incubated overnight at 28°C in the dark for 12 to 16 h. Fluorescence was observed with a filter-equipped microscope (BX61; Olympus, Tokyo, Japan) under a bright field, GFP channel, and red fluorescent protein channel.
Y2H Analyses
Y2H analysis was performed as described previously (Moon et al., 1999; Ryu et al., 2009). The haploid Saccharomyces cerevisiae strain YRG-2 was transformed by a modified lithium acetate protocol, using pBD_GAL4 and pGAD424 vectors that expressed Ehd1 or Ehd1 deletion derivatives. Transformants were grown on a minimal medium (i.e. a yeast nitrogen base without amino acids, Glc, essential amino acids, and agar) that lacked Leu, Trp, and His with or without 5 mm 3-amino-1,2,4-triazole.
Quantitative Assays of β-Galactosidase Activity
Plasmids containing the GAL4 DNA binding domain fused with Ehd1 full-length or deletion derivatives were transformed into yeast strain YRG-2. The empty vector (pBD_GAL4_empty) and SNB_1 ∼ 275 were used as the negative and positive control, respectively. Assays for β-galactosidase activity were performed as previously reported (Moon et al., 1999). Exponential-phase yeast cells (A600 = 0.6 ∼ 0.8) were collected and resuspended in Z buffer (60 mm Na2HPO4⋅7H2O, 40 mm NaH2PO4⋅7H2O, 10 mm KCl, and 0.1 mm MgSO4⋅7H2O, pH 7.0). Enzyme activity was measured in the harvested cells by using O-nitrophenyl β-d-galactopyranoside (Sigma-Aldrich, St Louis, MO) as a substrate. Activity, based on Miller units, was calculated by the following formula: 1000 × OD420 × the volume of cells (mL)−1 × incubation time (min)−1 × OD600−1.
BiFC Assays
The full-length cDNA or truncated fragments of Ehd1 and full-length OsRR1 cDNA were inserted into the pGA3815 or pGA3816 vectors that contained the maize ubiquitin 1 promoter, the nos terminator, and either the fragmented C terminus of Venus (aa 155–238) or the N terminus of Venus (aa 1–172), through the BamHI and MluI sites. The constructs were transformed into protoplasts isolated from the Oc cells, and the transformants were incubated overnight at 28°C in the dark. Fluorescence was observed with a filter-equipped microscope (BX61; Olympus) under a bright field or Yellow Fluorescent Protein channel.
Supplemental Data
The following supplemental materials are available.
Supplemental Figure S1. Subcellular localization of Ehd1.
Supplemental Figure S2. Heading date of Ehd1 OX plants under LD conditions.
Supplemental Figure S3. BiFC assays of RI fragment and IGC fragments, and serial deletion derivatives of RI and IGC.
Supplemental Figure S4. Analyses of transcripts levels of Ehd1 and truncated derivatives of Ehd1 over-expressing plants.
Supplemental Figure S5. Amino acid sequence alignment of R domain from Ehd1 and 13 type-A RRs in rice.
Supplemental Figure S6. Analyses of homomerization of wild-type or mutant Ehd1 by BiFC assays.
Supplemental Figure S7. Analyses of interaction between Ehd1 and OsRR1 by BiFC assays.
Supplemental Table S1. Primers used in this study.
ACKNOWLEDGMENTS
We thank Kyungsook An for generating the transgenic lines and handling the seed stock and Priscilla Licht for editing the English composition of the article.
Glossary
- BiFC
bimolecular fluorescence complementation
- C
C-terminal stretch
- CIP
calf intestinal alkaline phosphatase
- co-IP
coimmunoprecipitation
- DAG
days after germination
- DTT
dithiothreitol
- Ehd1-HA
Ehd1 fused to the HA tag
- Ehd1-Myc
Ehd1 fused to the Myc tag
- G
GARP DNA-binding
- HRP
horseradish peroxidase
- I
inter domain
- LD
long-day
- Oc
rice root-derived callus suspension
- OX
over-expression
- R
receiver
- RR
response regulator
- SD
short-day
- Y2H
yeast two-hybrid
LITERATURE CITED
An G, Ebert PR, Mitra A, Ha SB (1989) Binary vectors. In Plant Molecular Biology Manual. Kluwer Academic Publisher, Dordrecht, pp 1–19
Kieber JJ, Schaller GE (2014) Cytokinins the Arabidopsis Book. American Society of Plant Biologists 12
Author notes
This work was supported in part by grants from the Cooperative Research Program for Agriculture Science & Technology Development (Project No. PJ01108001), Rural Development Administration, Republic of Korea; the Republic of Korea Basic Research Promotion Fund (Grant No. NRF-2007-0093862); and Kyung Hee University (20130214) to G.A.
Address correspondence to [email protected]
The author responsible for distribution of materials integral to the findings presented in this article in accordance with the policy described in the Instructions for Authors (www.plantphysiol.org) is: Gynheung An ([email protected]).G.A. organized the entire of this research; L.-H.C. designed the experiment and analyzed the experimental data; L.-H.C., J.Y., and R.P. performed the experiments; L.-H.C. wrote the manuscript; and G.A. helped with the organization and editing it.
Articles can be viewed without a subscription.