-
PDF
- Split View
-
Views
-
Cite
Cite
Yu-Ling Hung, Syuan-Fei Hong, Wei-Lun Wei, Shiuan Cheng, Jia-Zhen Yu, Veny Tjita, Qian-Yuan Yong, Ryuichi Nishihama, Takayuki Kohchi, John L Bowman, Yuan-Chi Chien, Yen-Hsin Chiu, Ho-Chun Yang, Mei-Yeh Jade Lu, Zhao-Jun Pan, Chun-Neng Wang, Shih-Shun Lin, Dual Regulation of Cytochrome P450 Gene Expression by Two Distinct Small RNAs, a Novel tasiRNA and miRNA, in Marchantia polymorpha, Plant and Cell Physiology, Volume 65, Issue 7, July 2024, Pages 1115–1134, https://doi.org/10.1093/pcp/pcae029
- Share Icon Share
Abstract
The miR390-derived TAS3 trans-acting short-interfering RNAs (tasiRNAs) module represents a conserved RNA silencing pathway in the plant kingdom; however, its characterization in the bryophyte Marchantia polymorpha is limited. This study elucidated that MpDCL4 processes MpTAS3 double-stranded RNA (dsRNA) to generate tasiRNAs, primarily from the 5ʹ- and 3ʹ-ends of dsRNA. Notably, we discovered a novel tasiRNA, tasi78A, which can negatively regulate a cytochrome P450 gene, MpCYP78A101. Additionally, tasi78A was abundant in MpAGO1, and transient expression assays underscored the role of tasi78A in repressing MpCYP78A101. A microRNA, miR11700, also regulates MpCYP78A101 expression. This coordinate regulation suggests a role in modulating auxin signaling at apical notches of gemma, influencing the growth and sexual organ development of M. polymorpha and emphasizing the significance of RNA silencing in MpCYP78A101 regulation. However, phylogenetic analysis identified another paralog of the CYP78 family, Mp1g14150, which may have a redundant role with MpCYP78A101, explaining the absence of noticeable morphological changes in loss-of-function plants. Taken together, our findings provide new insights into the combined regulatory roles of miR390/MpTAS3/miR11700 in controlling MpCYP78A101 and expand our knowledge about the biogenesis and regulation of tasiRNAs in M. polymorpha.
Introduction
Trans-acting short-interfering RNA (tasiRNA) is a unique RNA silencing mechanism in the plant kingdom. In Arabidopsis, the TAS transcript undergoes cleavage by a microRNA (miRNA)–AGO1 complex. Subsequently, RNA-DEPENDENT RNA POLYMERASE 6 (AtRDR6) was used to transcribe the cleaved TAS fragments into double-stranded RNA (dsRNA). The dsRNAs are then processed by DICER-LIKE 4 (AtDCL4), leading to the generation of 21-nucleotide (nt) tasiRNAs in a head-to-tail arrangement (Allen et al. 2005, Yoshikawa et al. 2005). In particular, TAS3 is conserved throughout land plants (Xia et al. 2017). Studies in Arabidopsis indicate that the main components involved in TAS3 tasiRNA biogenesis include microRNA390 (miR390), AGRONAUTE 7 (AtAGO7), AtDCL4, HUA ENHANCER1 (AtHEN1) and AtRDR6 (Allen et al. 2005, Pegler et al. 2019). The TAS3 transcript is characterized by the presence of two miR390 target sites located at its 5ʹ- and 3ʹ-ends, a configuration described in the ‘two-hit’ model (Xia et al. 2017). This unique arrangement of miR390 target sites on the TAS3 transcript suggested that, over the course of evolution, TAS3 loci might have developed a bidirectional processing mechanism involving these two sites (Allen et al. 2005, Axtell et al. 2006). Studies have shown that the miR390–AtAGO7 complex binds to the 5ʹ-end target site without cleavage, while the 3ʹ-end target site is bound and cleaved by the complex (Allen et al. 2005, Montgomery et al. 2008a). Both binding to these target sites and the 3ʹ-end cleavage must be specifically carried out by AtAGO7 to initiate subsequent tasiRNA production (Allen et al. 2005, Montgomery et al. 2008a). The combination of miR390–AtAGO7 for TAS3 tasiRNA production cannot be replaced by other miRNAs or AtAGO1 (Allen et al. 2005, Montgomery et al. 2008a).
In Arabidopsis, the TAS1 and TAS2 transcripts are activated by miR173 cleavage, while the TAS4 transcripts are activated by miR828 cleavage (Allen et al. 2005, Rajagopalan et al. 2006, Montgomery et al. 2008b, Cuperus et al. 2010, Luo et al. 2012). Both miR173 and miR828 are 22 nt in length miRNAs and are loaded into AtAGO1 to initiate tasiRNA biogenesis. In contrast, miR390 is 21 nt in length, indicating that the mechanism of TAS3 tasiRNA production differs from that of other TASs. The genome of Marchantia polymorpha, a bryophyte, encodes miR390 but does not contain AGO7, raising the question of whether MpAGO1 carries miR390. Additionally, degradome data revealed evidence of cleavage at both the 5ʹ- and 3ʹ-ends of the miR390 cleavage sites of MpTAS3 (Xia et al. 2017, Lin and Bowman 2018), suggesting that the generation of tasiRNAs from MpTAS3 might adopt a two-cleave strategy.
In angiosperms, TAS3-derived tasiARF targets the clade B AUXIN RESPONSIVE FACTOR (ARF) family (tasiARF/ARF module), which plays a pivotal role in downregulating the auxin signaling pathway (Allen et al. 2005, Axtell et al. 2006, Garcia et al. 2006, Douglas et al. 2010, Yan et al. 2010, Xia et al. 2017). Additionally, the tasiARF/ARF module enhances the auxin response in Physcomitrium patens, leading to increase sensitive and stable expression of auxin-responsive genes (Plavskin et al. 2016). However, in M. polymorpha, the role of MpTAS3-derived tasiARF in MpARF2 regulation is currently unclear.
Instead of the tasiARF/ARF module regulating auxin signaling in angiosperms, Jiang et al. (2021) reported that a cytochrome P450 monooxygenase (CYP) superfamily member, CYP78A, can activate cytokinin signaling to promote the activation of auxin biosynthesis genes, increasing auxin content. In Arabidopsis, there are six members of the CYP78A subfamily (Bak et al. 2011). In bryophytes, P. patens has two CYP78As (PpCYP78A27 and PpCYP78A28), both of which have redundant functions affecting colony size, gametophore formation and hormone signaling during protonema growth and gametophore development (Katsumata et al. 2011). Marchantia polymorpha also contains two proteins, MpCYP78A101 (Mp3g23930) and Mp1g14150, but their functions remain unclear (Nelson 2018). In angiosperms, the regulation of CYP genes by miRNAs plays a pivotal role. For instance, in Jatropha curcas, CYP86A8 and CYP94A2 are regulated by Jcu-miR156f, while in wheat, CYP450 is under the regulatory control of miR9773 (Li et al. 2017, Yang et al. 2019). These results indicate the importance of miRNA-mediated modulation in maintaining the stability of CYP expression.
The genome of M. polymorpha encodes genes involved in RNA silencing based on sequence similarity (Bowman et al. 2017). Given the haploid chromosome set of M. polymorpha, mutations in essential genes could lead to lethal mutant strains, complicating functional studies. Additionally, due to the regulatory role of miR390, overexpression of the TAS3 transcript may not necessarily increase the yield of tasiRNAs. Moreover, a gain-of-function in the TAS3 transcript might result in an overproduction of tasiRNAs, which poses challenges for the specific analysis of individual tasiRNAs. All these factors make studying TAS3 tasiRNAs in M. polymorpha particularly challenging. Therefore, studies related to RNA silencing component genes have not been reported. In this study, we successfully knocked out the MpDCL4 and MIR390 genes using gene editing technology, confirming that MpDCL4 and miR390 are involved in M. polymorpha TAS3 tasiRNA production. We also identified a highly expressed tasiRNA, tasi78A, and demonstrated that tasi78A coordinately regulates MpCYP78A101 with miR11700 via the artificial miRNA (amiRNA) approach. Through gain-of-function and loss-of-function studies, we demonstrated that the miR390/tasi78A/miR11700 module regulates the expression of MpCYP78A101, affecting the sensitivity of M. polymorpha to auxin and controlling plant development.
Results
miR390/TAS3/MpARF2 pathway in M. polymorpha
We investigated whether the conserved regulatory mechanism of the miR390/MpTAS3 module also exists in M. polymorpha. Degradome profiling from the vegetative stage was employed to verify the specific sites where miRNA targets are cleaved in the RNA molecules (Lin et al. 2016). Two degradome-supported processing sites were previously assigned to MpTAS3 (Fig. 1A) (Lin et al. 2016). Fifty-nine degradome reads (2.09 RPM) were present at the 5ʹ-end of the miR390 target site of MpTAS3 (Fig. 1B), while only nine degradome reads (0.32 RPM) were observed at the 3ʹ-end of the target site (Fig. 1C). Additionally, we identified a target site of tasiARF at 136 nt from the start codon of MpARF2, with five degradome reads (0.18 RPM) at the cleavage site (Fig. 1D).
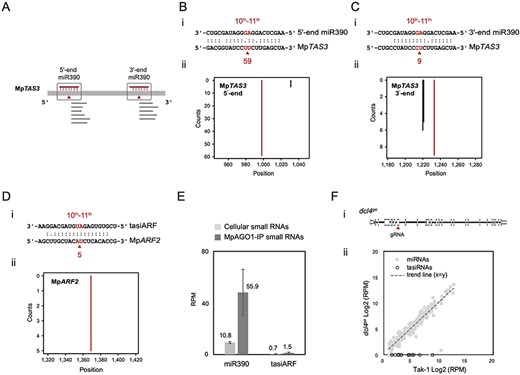
Target prediction of miR390 and tasiARF and the role of MpDCL4 in tasiRNA biogenesis in M. polymorpha. (A) Illustration of the two-hit configuration of miR390 to MpTAS3. (B) The 5ʹ-end target site of MpTAS3 paired with miR390. (C) The 3ʹ-end target site of MpTAS3 paired with miR390. (D) tasiARF and MpARF2. The alignments between the representative tasiRNAs or miRNAs and their target transcripts are shown below the corresponding t-plots. The arrowheads and the numbers below the arrowheads correspond to the positions of red lines and the read abundances, respectively. The x-axis of the t-plot is the position on the transcript starting from the transcription start site, and the y-axis is the number of reads detected from a position. Red lines indicate the signatures produced by tasiRNA- or miRNA-directed cleavage. (E) Bar plot of miR390 and tasiARF RPM values from the cellular and MpAGO1-IP profiles. (F) miRNAs and tasiRNAs in the dcl4ge mutant. Site of gene editing on the MpDCL4 genomic DNA (i). The scatter plot shows the RPM values of miRNAs (gray dots) and tasiRNAs (white dots) in Tak-1 plants and dcl4ge mutants (ii).
To verify the loading of miRNAs and tasiRNAs into MpAGO1, we conducted an immunoprecipitation experiment using α-MpAGO1 antibodies and sequenced the small RNAs bound to MpAGO1. Then, we compared the abundance of these small RNAs between the cellular small RNA-Seq profile (cellular profile) and the MpAGO1-immunoprecipitated small RNA-Seq profile (MpAGO1-IP profile) from the vegetative stage. The cellular and MpAGO1-IP profiles exhibited 10.8 RPM and 55.9 RPM of miR390, respectively (Fig. 1E), indicating enrichment of miR390 in MpAGO1. In contrast, we observed low RPM values for tasiARF (1.5 RPM) in the MpAGO-IP profile (Fig. 1E).
In Arabidopsis, AtDCL4 is the major component involved in tasiRNA processing. We investigated the role of MpDCL4 by performing CRISPR/Cas9 editing of the MpDCL4 genome (Fig. 1F) and obtained five dcl4ge mutants, all of which exhibited INDELs located on exon 7 of the MpDCL4 genome sequence (Supplementary Fig. S1A, B). Specifically, we used the male dcl4ge mutant line #7-10, which carries an insertion causing a frameshift mutation (Supplementary Fig. S1C, D), for small RNA analysis. We compared the composition of TAS3 tasiRNAs in Tak-1 plants and dcl4ge mutants. Our results showed the presence of tasiRNAs in Tak-1 plants but not in dcl4ge mutants (Fig. 1F). Furthermore, the RPM values of miRNAs in both Tak-1 plants and dcl4ge mutants were similar (Fig. 1F), indicating that MpDCL4 specifically affects the generation of tasiRNAs and functions like AtDCL4 in tasiRNA biogenesis. Overall, these findings provide evidence for the conserved regulatory mechanism of the miR390/MpTAS3 module in M. polymorpha, highlighting the role of MpAGO1 and MpDCL4 in small RNA processing and target cleavage.
MpTAS3-derived tasiRNAs are mainly processed from the 3ʹ-end phasing positions 1 and 2 of MpTAS3 transcripts
To further explore the biogenesis of MpTAS3-derived tasiRNAs, we mapped 21-nt small RNAs from the cellular and MpAGO1-IP profiles to MpTAS3 transcripts. Our analysis revealed that MpTAS3-derived phased 21-nt tasiRNAs could be initiated from different positions of MpTAS3 in a phased pattern, indicating the influence of MpDCL4 on tasiRNA production. However, whether MpDCL4 can cleave both directions of double-strand MpTAS3 remains unknown. Thus, the directionality of MpDCL4, whether it starts from the 5ʹ-end and/or 3ʹ-end, still requires further investigation. Given that AtAGO7–miR390 does not cleave the 5ʹ-end of AtTAS3, the generation of tasiRNAs in Arabidopsis initiates from the 3ʹ-end of the AtTAS3 transcript. To maintain a uniform comparative basis between M. polymorpha and Arabidopsis, the 3ʹ-end of the MpTAS3 transcript has also been selected as the initiation point for tasiRNA production.
To define the potential phasing positions, we assigned the 10th nt of the 3ʹ-end of the miR390 cleavage site as position 1, with subsequent positions having a 1-nt shift toward the 5ʹ-end, denoted as position 2, position 3 and so on (Fig. 2A). In the different positions analyzed, the tasiRNAs initiated from the 3ʹ-end at position 2 (3ʹ-end P2), which are equivalent to those initiated from the 5ʹ-end at position 4 (5ʹ-end P4), were identified as the most abundant among the MpTAS3-derived tasiRNAs (Fig. 2B, panel i; Supplementary Fig. S2). Overall, position 2 exhibited the highest proportion of tasiRNAs, accounting for more than 60% of the tasiRNAs in both the cellular and MpAGO-IP profiles (Fig. 2B, panel ii; Supplementary Fig. S2), indicating that tasiRNAs derived from MpTAS3 are predominantly generated from phasing position 2 and efficiently loaded into MpAGO1. Additionally, approximately 5–15% of tasiRNAs can also be loaded into MpAGO1 from phasing position 1 (3ʹ-end P1 or 5ʹ-end P5) (Fig. 2B, panel ii; Supplementary Fig. S2). The results indicate that the tasiRNAs loaded into MpAGO1 primarily originate from phasing positions 1 and 2. In Arabidopsis, tasiRNAs are also primarily produced and loaded into AtAGO1 from phasing positions 1 and 2 (Fig. 2B, panels iii and iv). These observations highlight the similarity in tasiRNA biogenesis mediated by MpDCL4 in M. polymorpha compared to Arabidopsis.
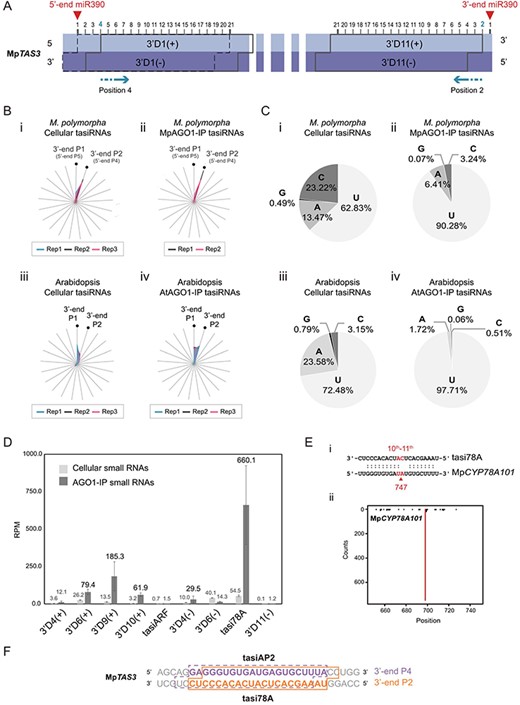
MpTAS3-derived phased 21- nt tasiRNAs and their corresponding targets in M. polymorpha. (A) Illustration of the positions and orientations of the predicted DICER processing cleavage sites on MpTAS3. Red arrowheads indicate the 5ʹ-end and 3ʹ- end miR390 cleavage sites. The MpTAS3-derived tasiRNAs were named the number-phasing position. The symbols of (+) and (−) represent the tasiRNA fragments generated from the sense and antisense strands, respectively. (B) Phasing position analysis of TAS3-derived 21-nt tasiRNAs from cellular and AGO1-IP profiles in M. polymorpha and Arabidopsis. The radar plots represent the ratio of tasiRNAs from each of the 21 phasing positions. Rep-number represents the number of biological replicates. (C) The 5ʹ-end 1st nucleotide of the tasiRNAs from the cellular and AGO1-IP profiles in M. polymorpha and Arabidopsis. (D) Bar plot of the RPM values of tasiRNAs from 3ʹ-end phasing position 2 in the cellular and MpAGO1-IP profiles. (E) Alignments and target plots of tasi78A and MpCYP78A101. (F) Sequences of tasi78A and tasiAP2 in M. polymorpha.
Moreover, we analyzed the 5ʹ-end nucleotide composition of the tasiRNAs derived from the phasing positions 1 and 2. Notably, most of these tasiRNAs (62.83%) had a 5ʹ-end 1st-U (Fig. 2C, panel i). When tasiRNAs were loaded into MpAGO1, the paired strands of MpTAS3-derived tasiRNAs exhibited unequal amounts. Consequently, 90.28% of the MpAGO1s loaded with MpTAS3-derived tasiRNAs from phasing positions 1 and 2 were 5ʹ-end 1st-U, 6.41% were 5ʹ-end 1st-A, 3.24% were 5ʹ-end 1st-C, and 0.07% were 5ʹ-end 1st-G (Fig. 2C, panel ii). In Arabidopsis, the 5ʹ-end nucleotides of tasiRNAs are primarily composed of U (72.48%) and A (23.58%) (Fig. 2C, panel iii), but when they are loaded into AtAGO1, they are mainly composed of U (97.71%) (Fig. 2C, panel iv). These findings provide valuable insights into the preferential loading and potential regulatory role of specific tasiRNAs mediated by MpAGO1 and AtAGO1.
A novel tasi78A regulates MpCYP78A101
To further understand the role of tasiRNAs in RNA silencing, we examined the abundance of MpTAS3-derived tasiRNAs from the 3ʹ-end phasing position 2 in the MpAGO1-IP profile. Our analysis revealed that a novel tasiRNA, tasi78A (660.1 RPM), was significantly enriched (Fig. 2D). Moreover, the degradome data revealed 747 degradome reads (26.89 RPM) at the target site (138 nt) in the MpCYP78A101 transcript (Fig. 2E), indicating that MpCYP78A101 is the primary target of tasi78A. Based on small RNA target identification, tasi78A exhibited 14 putative target genes; however, the other genes displayed only a few degradome reads (fewer than 5 reads, Supplementary Fig. S3), further supporting the primary target role of tasi78A on MpCYP78A101. Furthermore, we observed enrichment of four other MpTAS3-derived tasiRNAs, namely tasi2-3ʹD6(+) (79.4 RPM), tasi2-3ʹD9(+) (185.3 RPM), tasi2-3ʹD10(+) (61.9 RPM) and tasi2-3ʹD4(−) (29.5 RPM) (Fig. 2D). However, none of these tasiRNAs cleave putative target transcripts based on the prediction and degradome profiles (Supplementary Fig. S4). Notably, the very weak degradation of MpARF2 (Fig. 1D) was supported by the low abundance of tasiARF (0.7 RPM in the cellular profile and 1.5 RPM in the MpAGO1-IP profile) (Fig. 2D). Additionally, tasi78A, the reverse and complementary sequence to tasiAP2, originated from the phasing position 4 (Fig. 2F). A previous study suggested that the tasiAP2 sequence is unique to non-vascular plants (Morozov et al. 2018). Therefore, it is reasonable to infer that the reverse complemented tasi78A may also be conserved in non-vascular plants.
miR390 leads to the production of tasiRNAs in M. polymorpha
To further investigate the miR390/MpTAS3 regulatory pathway, we generated transgenic lines overexpressing the MIR390 gene under the control of the EF1α promoter (MIR390OE plant) and created MIR390 knockout mutants (mir390ge mutant). In the mir390ge mutants, the structure of the miR390 precursor showed a conformational change in either the miR390 mature strand or the star stand (Fig. 3A), implying the absence of miR390 production. Northern blot analysis revealed that the levels of miR390 were significantly increased in the MIR390OE plants than in the Tak-1 plants; however, the levels of tasiARF and tasi78A only show slightly increased (Fig. 3B). The small RNA profile also confirmed the expression of miR390, tasiARF, and tasi78A in the MIR390OE plants (Fig. 3C). In contrast, no detectable miR390 was detected in the four mutants when we checked their small RNA-Seq profiles (Fig. 3C). Furthermore, tasiARF and tasi78A were not detected in the mir390ge mutants, indicating the loss of MpTAS3-derived tasiRNA generation in these mutants (Fig. 3C). Notably, we unexpectedly detected a 24-nt variant of miR390 (5ʹ-ACAAAGCUCAGGAGGGAUAGCGUC-3ʹ), in the MIR390OE plants, the reason for which remains unknown (Fig. 3B, C).
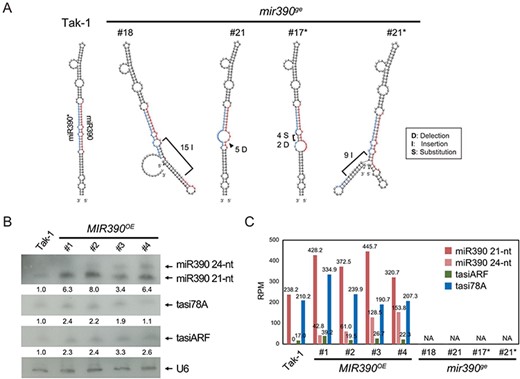
Small RNA expression patterns of miR390, tasiARF and tasi78A in the mir390ge mutants and MIR390OE plants. (A) Secondary structure prediction of the wild type and edited miR390 precursors. The mature sequence is highlighted in red, and the star sequence is highlighted in blue. (B) Northern blotting of miR390, tasiARF and tasi78A in the MIR390OE plants. U6 was used as a loading control. (C) Small RNA profiles of miR390, tasiARF and tasi78A in the MIR390OE plants and mir390ge mutants.
MpCYP78A101 was regulated by both tasi78A and miR11700
In addition to tasi78A, we identified a miRNA target site on MpCYP78A101 (Fig. 4A). The target site is at position 237 nt and is targeted by miR11700, which exhibited 338 degradome reads (12.17 RPM) with a prominent peak at the targeting position (Fig. 4A). Moreover, tasi78A (660.1 RPM) and miR11700 (354.7 RPM) were significantly enriched in the MpAGO1-IP profile (Figs. 2D, 4B).
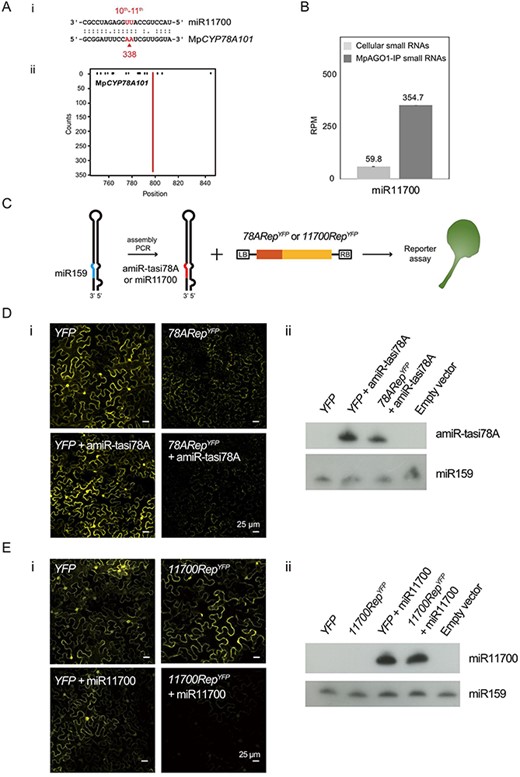
Target prediction of mi11700 and the reporter assays of amiR-tasi78A, miR11700 and MpCYP78A101 in N. benthamiana. (A) Alignment and target plot of miR11700 and MpCYP78A101. The alignments between the representative miRNAs and their target transcripts are shown above the t-plots. The arrowheads and the numbers below the arrowheads correspond to the positions of red lines and the read abundances, respectively. The x-axis of the t-plot is the position on the transcript starting from the transcription start site, and the y-axis is the number of reads detected from a position. Red lines indicate the signature produced by miRNA-directed cleavage. (B) Bar plot of the RPM values of miR11700 from the cellular and MpAGO1-IP profiles. (C) Illustration of the transient expression in N. benthamiana for assessing the activity of amiR-tasi78A or miR11700 alongside their reporters, 78ARepYFP and 11700RepYFP. (D) Transient reporter assays of amiR-tasi78A and 78ARepYFP (78ARepYFP + amiR-tasi78A) in the leaves of N. benthamiana. The constructs expressing YFP and amiR-tasi78A (YFP + amiR-tasi78A) were co-expressed in N. benthamiana as a negative control (i). Northern blotting of amiR-tasi78A in infiltrated plants. The miR159 was used as a loading control (ii). (E) Transient reporter assays of miR11700 and 11700RepYFP (11700RepYFP + miR11700) in leaves of N. benthamiana. The constructs expressing YFP and miR11700 (YFP + miR11700) were co-expressed in N. benthamiana as a negative control (i). Northern blotting of miR11700 in infiltrated plants. The miR159 was used as a loading control (ii).
To further examine the regulatory relationship between small RNAs (tasi78A and miR11700) and MpCYP78A101, transient expression reporter assays were performed in Nicotiana benthamiana. To ectopically express tasi78A, we constructed an amiRNA for tasi78A (amiR-tasi78A) (Fig. 4C). The chimeric construct of YELLOW FLUORESCENT PROTEIN (YFP) fused with the tasi78A target site of MpCYP78A101 (78ARepYFP) exhibited fluorescent signals in the cytoplasm (Fig. 4D, panel i). Interestingly, co-expressing 78ARepYFP with amiR-tasi78A (78ARepYFP + amiR-tasi78A) reduced the YFP fluorescence (Fig. 4D, panel i). In contrast, there was no difference in YFP fluorescence between YFP alone and YFP co-expressing amiR-tasi78A (YFP + amiR-tasi78A) (Fig. 4D, panel i). Moreover, the presence of amiR-tasi78A was confirmed by northern blotting in the samples co-expressing amiR-tasi78A (Fig. 4D, panel ii). These data suggested that amiR-tasi78A can cleave the RNA substrate containing the tasi78A target site within MpCYP78A101.
Similarly, when the substrate 11700RepYFP was co-expressed with miR11700, a decrease in YFP fluorescence was observed compared to that of the YFP control (Fig. 4E, panel i). However, no difference in YFP fluorescence was detected between samples expressing YFP alone and those co-expressing YFP and miR11700 (Fig. 4E, panel i). Northern blotting confirmed the high expression of miR11700 in the samples expressing miR11700 (Fig. 4E, panel ii). In addition, all YFP fluorescence intensities were quantified (Supplementary Fig. S5). The results indicated that the expression of both 78ARepYFP and 11700RepYFP decreased by less than 0.2-fold in the amiR-tasi78A and miR11700 samples, respectively (Supplementary Fig. S5). These findings indicated that both tasi78A and miR11700 can target MpCYP78A101.
Promoter assay for MIR390, MpTAS3, MIR11700 and MpCYP78A101
A promoter assay was conducted to investigate the expression patterns of MIR390, MpTAS3, MIR11700 and MpCYP78A101. Robust signals of MIR390pro:Citrine-nuclear localization signal (NLS) and MpCYP78A101pro:Citrine-NLS signals were detected at the apical notch, the site of the apical meristem, in gemma (Fig. 5A, B). Additionally, signals of MpTAS3pro:Citrine-NLS and MIR11700pro:Citrine-NLS signals were also observed at the apical notch of the gemma, although the signals were relatively weak (Fig. 5C, D). The overlapping expression patterns observed in the promoter assays suggested that the miR390/MpTAS3 module and MIR11700 may regulate MpCYP78A101 at the apical notch area in the gemma. Furthermore, signals of MIR390pro:Citrine-NLS and MpTAS3pro:Citrine-NLS were also detected in the gemma cup (Fig. 5A, C), indicating that the miR390/MpTAS3 module may influence the development of the gemma cup. Notably, Tak-1 plants served as a negative control in the promoter assay, where no citrine fluorescence was observed (Fig. 5E).
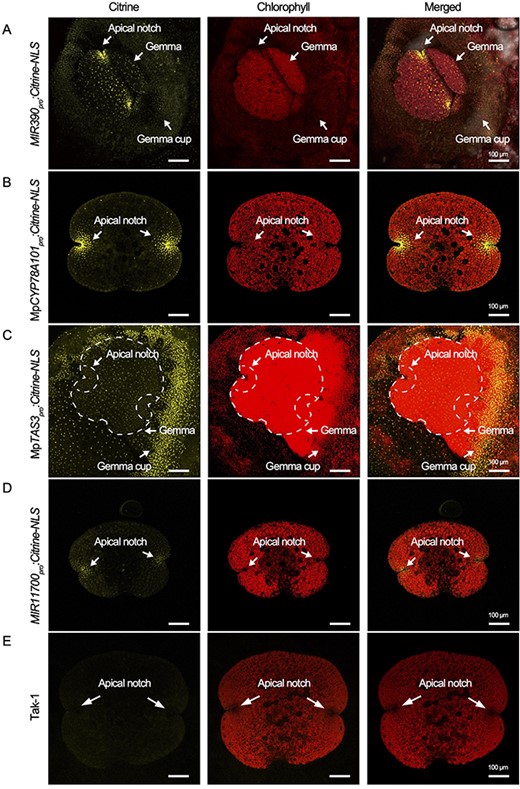
Expression patterns of the MIR390/MpTAS3 module, MIR11700, and MpCYP78A101 in M. polymorpha. Confocal images of the MIR390pro:Citrine-NLS (A), MpCYP78A101pro:Citrine-NLS (B), MpTAS3pro:Citrine-NLS (C) and MIR11700pro:Citrine-NLS (D) transgenic plants during gemma development. (E) Tak-1 plant was used as a negative control.
tasi78A negatively regulates MpCYP78A101 in M. polymorpha
Our observations revealed that MIR390OE plants and mir390ge mutants exhibited no noticeable phenotypic differences from Tak-1 plants, including differences in gemma cup formation, thallus morphology and sexual organ development (Supplementary Fig. S6A, B). Notably, upregulation of MpCYP78A101 was observed exclusively in the mir390ge mutants (Supplementary Fig. S6C). However, overexpression of miR390 in MIR390OE plants did not significantly reduce the expression of MpCYP78A101 only because of the slightly increased tasi78A (Fig. 3B, C; Supplementary Fig. S6D, E). Therefore, to investigate the silencing effect of tasi78A, we generated a transgenic M. polymorpha expressing an amiR-TASI78A gene (amiR-TASI78AOE plant) (Fig. 6A). However, no significant phenotypic alterations were observed in amiR-TASI78AOE plants (Fig. 6A). High expression levels of amiR-tasi78A (33- to 35.8-fold) were detected by northern blotting (Fig. 6B), and the expression of MpCYP78A101 was downregulated (Fig. 6C). Consistent results were obtained when 78ARepYFP was transiently expressed in amiR-TASI78AOE plants (Fig. 6D) by agropenetration, providing further support for the regulatory role of tasi78A in modulating the expression of MpCYP78A101.
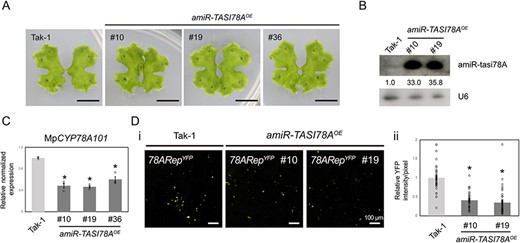
Phenotypic observations and reporter assays of 78ARepYFP in the amiR-TASI78AOE plants. (A) Vegetative growth of the 18-day-old amiR-TASI78AOE plant G3 lines. Scale bars, 1 cm. (B) Northern blotting of amiR-tasi78A in amiR-TASI78AOE plants. U6 served as a loading control. (C) qRT-PCR of MpCYP78A101 in the amiR-TASI78AOE plants. MpEF1-alpha was used as a reference gene. Error bars represent the standard error from three biological replicates. (D) YFP transient reporter assays of 78ARepYFP in the amiR-TASI78AOE plants (i). The quantified relative YFP intensity of the data was calculated from more than 30 images captured by a fluorescence microscope (ii). Tak-1 plants were used as control. Error bars represent the standard error from three biological replicates.
miR11700 may regulate MpCYP78A101 at the reproductive stage in M. polymorpha
To confirm the function of miR11700, we generated mir11700ge mutants and transgenic M. polymorpha expressing the MIR11700 gene (MIR11700OE plants). In the mutants, changes in the secondary structure of the miR11700 precursor suggested that the generation of miR11700 was impaired (Fig. 7A). In various individual mir11700ge mutants, the expression of miR11700 was undetectable (Fig. 7B). Notably, in the mir11700ge mutant lines #2 and #7, an approximately 1.8-fold increase in the expression of MpCYP78A101 was detected (Fig. 7C). The lack of MpCYP78A101 upregulation in all the mir11700ge mutants might be attributed to the persistent control of MpCYP78A101 by endogenous tasi78A. In the vegetative stage, the thallus phenotype of the mir11700ge mutants was similar to that of Tak-1 plants (Fig. 7D). However, in the reproductive stage, the mutants exhibited a noticeable increase in thallus width compared to that of Tak-1 plants (Fig. 7D and Supplementary Fig. S7A).
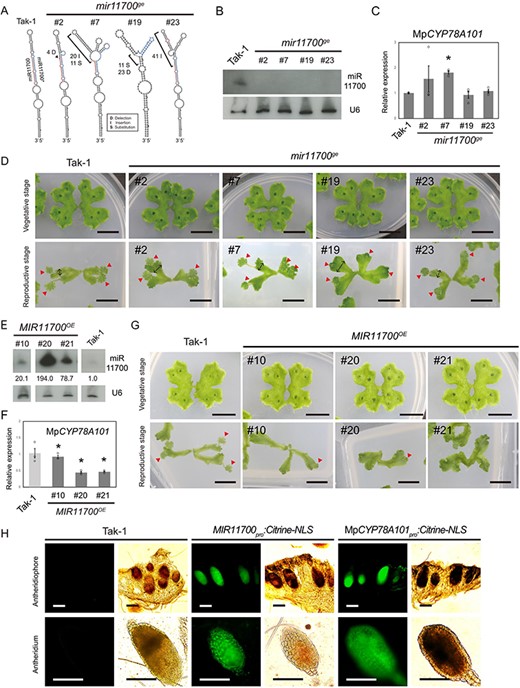
Phenotypic observations and expression analysis of the mir11700ge mutants and MIR11700OE plants. (A) Secondary structure prediction of the wild type and edited miR11700 precursors. The mature and star sequences of miRNAs are highlighted in red and blue, respectively. (B) Northern blotting of miR11700 in the mir11700ge mutants. (C) qRT-PCR of MpCYP78A101 in the mir11700ge mutants. (D) Plant growth of the mir11700ge mutants. (E) Northern blotting of miR11700 in the MIR11700OE plants. (F) qRT-PCR of MpCYP78A101 in the MIR11700OE plants. (G) Plant growth of the MIR11700OE plants. U6 served as a loading control. EF1-alpha was used as a reference gene. Error bars represent the standard error from three biological replicates. The plants in the vegetative and reproductive stages were 2.5-week-old and 3.5-week-old plants, respectively. Scale bar, 1 cm. The bidirectional arrow indicates the width of the thallus, while the red arrowhead indicates the male sexual organs. (H) Expression patterns of MIR11700 and MpCYP78A101 in the male sexual organs of M. polymorpha. Confocal images of the antheridiophore (upper panel) and antheridium (lower panel) of the MIR11700pro:Citrine-NLS and MpCYP78A101pro:Citrine-NLS plants were obtained at the reproductive stage. Tak-1 plant was used as a negative control. Scale bars, 200 μm.
Moreover, three distinct MIR11700OE plants exhibited significant variation in the expression of miR11700 (20.1- to 194-fold) (Fig. 7E). Correspondingly, the expression of endogenous MpCYP78A101 decreased, showing variations that matched the differing overexpression levels of miR11700. These data suggested that miR11700 effectively reduces the expression of MpCYP78A101 (Fig. 7E, F). Additionally, these MIR11700OE plants exhibited vegetative growth morphology similar to that of Tak-1 plants (Fig. 7G). However, at the reproductive stage, the thalli of the mir11700ge mutants appeared more expansive than those of the Tak-1 plants, while the number of sexual organs remained unchanged (Fig. 7D, arrowheads). In contrast, in the MIR11700OE plants, the number of sexual organs was reduced (Fig. 7G, arrowheads; and Supplementary Fig. S7B). Using promoter-reporter assays, we found that the fluorescence of citrine-NLS expressed under the control of both the MIR11700OE and MpCYP78A101 promoters was uniformly distributed throughout the entire antheridium in the transgenic plants (Fig. 7H). These data suggested that miR11700 may regulate MpCYP78A101, specifically during the reproductive stage in M. polymorpha.
Evaluation of the regulatory relationships between tasi78A, miR11700 and MpCYP78A101 in M. polymorpha
Due to the lack of phenotypic alterations in the MIR390OE plants and mir390ge mutants (Supplementary Fig. S6A, B), we generated mir390/11700ge mutants and double transgenic M. polymorpha plants expressing the MIR390 and MIR11700 genes (MIR390/11700OE plants) (Fig. 8). In loss-of-function mutants, the altered secondary structure of the miR390 and miR11700 precursors suggested that the production of miR390 and miR11700 was disrupted (Fig. 8A). Northern blot analysis revealed that in the mir390/11700ge mutants, both the miR390 and miR11700 levels were undetectable. Conversely, MIR390/11700OE plants demonstrated substantial overexpression of these miRNAs, ranging from 1.6- to 8.8-fold for miR390 and miR11700 (Fig. 8B, C). No obvious phenotype was observed in the mir390/11700ge mutants (Fig. 8D). This absence of a phenotype could be attributed to the slight increase in MpCYP78A101 expression in gemmae, while its expression in thalli remains nearly unchanged (Fig. 8E and Supplementary Fig. S8). These MIR390/11700OE plants appeared to have normal thalli, but the number of gemma cups varied (Fig. 8F). Notably, MpCYP78A101 expression was significantly lower in these MIR390/11700OE plants (Fig. 8G, panel i), while MpARF2 expression remained unchanged (Fig. 8G, panel ii). These data suggested that the disrupted development of gemma cups could be due to reduced MpCYP78A101 when miR390 and miR11700 are overexpressed. To further confirm the regulatory relationship between miR11700 and MpCYP78A101, we conducted a reporter assay by transiently expressing 11700RepYFP in mir390/11700ge mutants and MIR390/11700OE plants. The results indicated that YFP fluorescence was greater in the mir390/11700ge mutants and significantly decreased in the MIR390/11700OE plants (Fig. 8H), supporting the regulated role of miR11700 in altering the expression of MpCYP78A101. Considering the role of tasi78A in regulating MpCYP78A101 (Fig. 6D), we suggested that tasi78A and miR11700 coordinate in the RNA silencing-mediated regulation of MpCYP78A101. Moreover, without NAA (1-naphthaleneacetic acid) treatment, the epidermal cell sizes of dcl4ge and mir390/11700ge mutants were approximately 1.2-fold (Fig. 8I; Supplementary Fig. S9E). In contrast, the cell sizes of mir390ge mutants and amiR-TASI78AOE plants were comparable to those in Tak-1 plants (Fig. 8I; Supplementary Fig. S9E).
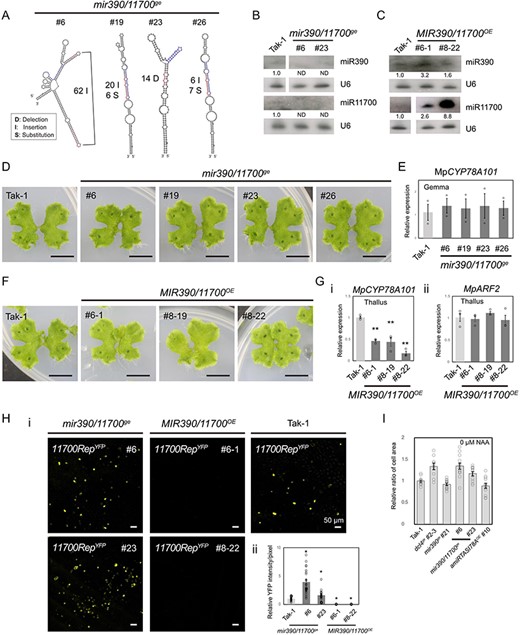
Phenotypic observations and reporter assays of 11700RepYFP in the mir390/11700ge mutants and MIR390/11700OE plants. (A) Secondary structure prediction of the wild type and edited miR11700 precursors. The mature sequence is highlighted in red and the star sequence is highlighted in blue. The mir390ge mutant was used as the genetic background to mutate miR11700. Northern blotting of miR390 and miR11700 in the mir390/11700ge mutants (B) and MIR390/11700OE plants (C), respectively. (D) Vegetative growth of the 18-day-old mir390/11700ge mutants. Scale bars, 1 cm. (E) qRT-PCR analysis of MpCYP78A101 in the gemmae of the mir390/11700ge mutants. (F) Vegetative growth of the 18-day-old MIR390/11700OE plants. Scale bars, 1 cm. (G) qRT-PCR analysis of MpCYP78A101 (i) and MpARF2 (ii) in the thallus of MIR390/11700OE plants. (H) YFP-transient reporter assays of 11700RepYFP in the mir390/11700ge mutants and MIR390/11700OE plants (i). The YFP intensity was quantified using more than 20 images captured by a fluorescence microscope (ii). Tak-1 was used as a control. Error bars represent the standard error from three biological replicates. (I) Relative cell area ratios of various plants in response to Tak-1 plants without NAA treatment. Epidermal cells in the central region of gemma were used for area calculation.
The dcl4ge, mir390ge and mir390/11700ge mutants display an NAA-less sensitive phenotype
The presence of tasiARF in miR390-mediated tasiRNAs is known to negatively regulate ARFs in Arabidopsis, thus influencing the auxin response. In this study, to elucidate the effects of tasiRNAs on the auxin response, plant growth and development, we treated dcl4ge, mir390ge, mir390/11700ge and amiR-TASI78A plants, as well as Tak-1 plants, with external NAA. Compared with other concentrations of NAA, 0.5 μM NAA significantly inhibited thallus growth in gemmalings derived from different plants (Fig. 9A; Supplementary Fig. S9A–D). Conversely, in the absence of NAA, these plants did not exhibit any notable difference in thallus size (Fig. 9A, B). The mean relative thallus areas of the dcl4ge and mir390/11700ge mutants were greater than that of Tak-1 plants, whereas those of the amiR-TASI78AOE plants were smaller (Fig. 9A, B).
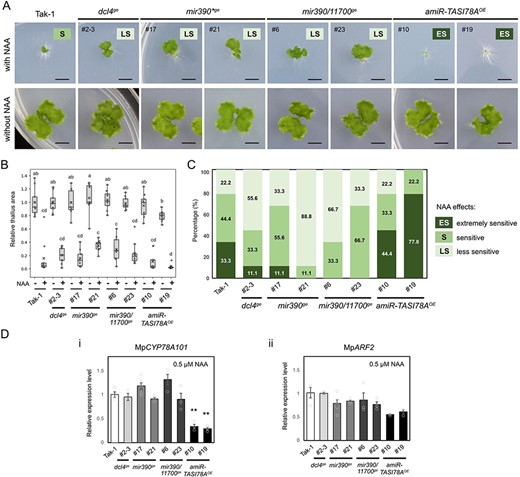
NAA treatment of dcl4ge, mir390ge, mir390/11700ge mutants and amiR-TASI78AOE plants. (A) Comparative phenotypes of Tak-1 plants, dcl4ge, mir390ge, mir390/11700ge mutants and amiR-TASI78AOE plants (n = 36). These images were captured 14 days post-gemma germination on the media supplemented with 0.5 µM NAA (upper panel) and without NAA (lower panel). ‘ES’ represents an extremely sensitive phenotype; ‘S’ represents a sensitive phenotype; ‘LS’ represents a less sensitive phenotype. Scale bar, 5 mm. (B) A boxplot illustrating the relative thallus area within each box. The horizontal band denotes the median, and the cross indicates the mean. The lower and upper edges of the box represent the first and third quartiles, respectively. Individual data points derived from a minimum of nine independent plants are shown as dots. Statistical significance was assessed using Tukey’s honestly significant difference test, with letters above the boxplots denoting significant differences (P-value < .05). (C) The frequency distribution of NAA effects was classified across various plants. An area ratio (with NAA/without NAA) less than 0.025 indicated an extremely sensitive phenotype, an area ratio between 0.025 and 0.2 indicated a sensitive phenotype and an area ratio greater than 0.2 indicated a less sensitive phenotype. (D) qRT-PCR analysis of MpARF2 (i) and MpCYP78A101 (ii), using MpEF1-alpha as the internal control. Error bars represent the standard error (SE) from three biological replicates.
To more accurately delineate NAA sensitivity, we classified plants with a thallus area ratio (with NAA/without NAA) less than 0.025 as extremely sensitive phenotypes, those with a ratio ranging from 0.025 to 0.2 as sensitive phenotypes, and those with a ratio exceeding 0.2 as less sensitive phenotypes (Fig. 9A). According to these categories, approximately 33.3% of Tak-1 gemmalings exhibited highly sensitivity to NAA, 44.4% showed sensitivity and 22.2% presented less sensitivity (Fig. 9C). Among the various knockout mutants, only 11.1% of the gemmalings of the dcl4ge mutant and mir390ge mutant showed an extremely sensitive phenotype to NAA; however, most of them were classified as sensitive (11.1–55.6%) or less sensitive (33.3–88.8%) phenotypes (Fig. 9C). In contrast, a significant proportion of amiR-TASI78AOE gemmalings #10 and #19 exhibited extremely sensitive (44.4% and 77.8%) and sensitive (22.2% and 33.3%) phenotypes, respectively (Fig. 9C). Additionally, gain-of-function of tasi78A in amiR-TASI78AOE plants resulted in the downregulation of MpCYP78A101 under NAA condition (Fig. 9D, panel i), suggesting that the levels of MpCYP78A101 could alter the sensitivity of gemmalings to auxin.
We assessed the MpARF2 expression in these gemmalings to investigate the morphological changes under NAA treatment. Despite a noticeable reduction in MpARF2 expression in amiR-TASI78AOE plants under NAA treatment, the decrease was not statistically significant. In contrast, other mutants exhibited no significant differences in MpARF2 expression when compared to Tak-1 plants (Fig. 9D, panel ii), suggesting that the morphological variations observed in the mutants and transgenic plants under NAA treatment may not be attributed to alterations in MpARF2 expression. Instead, they are more likely related to auxin-signaling regulation mediated by MpCYP78A101.
Target sites of tasi78A and miR11700 in CYP78 homologs during land plant evolution
By aligning the CYP78 homologs in land plants, we identified target sites for tasi78A in the bryophyte lineage (Fig. 10, labeled with an orange circle). However, no corresponding target site was found in Mp1g14150, a paralog of MpCYP78A101, or in other CYP78 homologs of vascular plants (Fig. 10; Supplementary Fig. S10). These findings suggest that tasi78A-mediated regulation may only occur in bryophytes. We also identified the targeting site for miR11700 of MpCYP78A101 within land plants. Interestingly, the target site for miR11700 is present only in MpCYP78A101 and not in other bryophytes or vascular plants (Fig. 10, labeled with a blue square). Although fewer mismatches are observed in the base pairing between miR11700 and its target site in Ceratodon purpureus (Cp3G030000), Ceratopteris richardii (Cr1Z230800) and Oryza sativa (Os08g43390), these mismatches are evenly distributed (Fig. 10, labeled with an open square; Supplementary Fig. S11). This distribution suggested that miR11700 is still incapable of cleaving its target site.
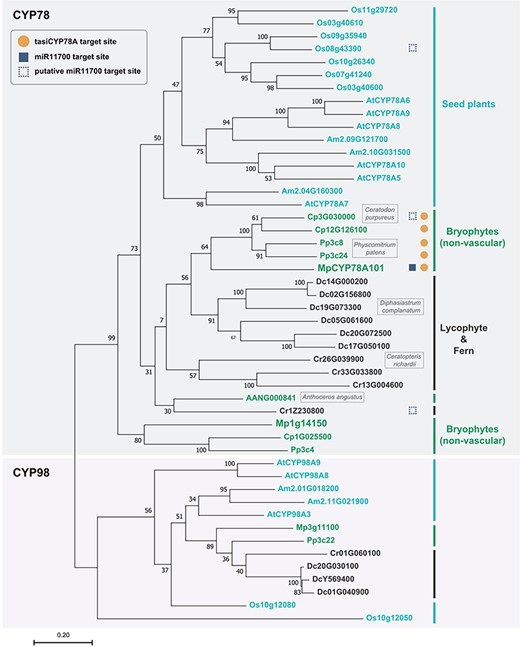
Phylogenetic tree of CYP78 homologs. CYP78 homologs in bryophytes, lycophytes, ferns and seed plants. The orange circles indicate the CYP78 homologs with the tasi78A target site. The blue squares indicate the CYP78 homologs with the miR11700 target site and the open squares indicate the CYP78 homologs with the putative miR11700 target site. Bryophytes (Anthoceros angustus, Ceratodon purpureus, Physcomitrium patens and Marchantia polymorpha), lycophytes (Diphasiastrum complanatum), ferns (Ceratopteris richardii) and seed plants (Arabidopsis thaliana, Oryza sativa, Amborella trichopoda). CYP98 homologs were used as an outgroup. The phylogenetic relationships were constructed by MEGAX using the ML criterion with 1,000 bootstrap replicates.
Discussion
In Arabidopsis, the length of a miRNA determines whether AtAGO1 mediates RNA silencing or generates tasiRNAs. The miRNA that regulates RNA silencing is primarily 21 nt in length, whereas the miRNA that generates TAS1 and TAS2 tasiRNAs is 22 nt in length (Allen et al. 2005, Rajagopalan et al. 2006, Montgomery et al. 2008b, Chen et al. 2010, Cuperus et al. 2010). In the plant kingdom, the miR390/TAS3 module is a highly conserved regulatory module, with miR390 involved in producing TAS3 tasiRNAs from bryophytes to angiosperms (Xia et al. 2017, Lin and Bowman 2018). Notably, miR390 in any plant species is 21 nt in length and not 22 nt. In Arabidopsis, TAS3 is regulated explicitly by AtAGO7 and not through AtAGO1. AtAGO7 is specialized in carrying miR390 (Montgomery et al. 2008a). However, as M. polymorpha does not have AGO7, MpAGO1 carries miR390 to participate in MpTAS3 tasiRNAs generation.
Another unique regulatory phenomenon of the miR390/TAS3 module is the ‘two-hit’ model (Allen et al. 2005, Xia et al. 2017, Lin and Bowman 2018). The TAS3 transcript has two miR390 target sites that facilitate miR390–AtAGO7 binding. In Arabidopsis, the 5ʹ-end target site only provides for miR390–AtAGO7 binding and is not cleaved, while the 3ʹ-end target site undergoes miR390–AtAGO7 binding and cleavage (Allen et al. 2005). Thus, Arabidopsis follows a ‘two-hit and one-cleave’ model to produce TAS3 tasiRNAs, with AtRDR6 and AtDCL4 generating tasiRNAs starting from the 3ʹ-end. However, both miR390 cleavage sites on TAS3 transcripts in M. polymorpha are bound and cleaved by miR390-MpAGO1, suggesting a potential ‘two-hit and two-cleave’ tasiRNA production model.
Like AtDCL4, we also confirmed that MpDCL4 is involved in tasiRNA production. TAS3 tasiRNAs were no longer produced in the dcl4ge mutant, making this mutant line valuable for future research on tasiRNAs or phasiRNAs. Through the small RNA profiles of tasiRNAs, we found that MpDCL4-produced tasiRNAs from double-stranded TAS3 transcripts at 3ʹ-end positions 2 or 1 are identical to those produced from 5ʹ-end positions 4 or 5. These few types of tasiRNAs constitute 70% of all tasiRNAs and are the main TAS3 tasiRNAs. We speculate that MpDCL4 may produce the main tasiRNAs either from 3ʹ-end positions 2 or 1 or from 5ʹ-end positions 4 or 5. According to the ‘two-hit and one-cleave’ model, the main TAS3 tasiRNAs in Arabidopsis are produced from the 3ʹ-end positions 2 or 1, which is consistent with the 3ʹ-end positions of M. polymorpha. Additionally, the observation of enlarged cell size in the dcl4ge and mir390/11700ge mutants suggested that RNA silencing plays a crucial role in cell expansion in M. polymorpha. However, besides tasi78A, whether other taisRNAs are also involved in cell expansion that needs further investigation.
MpAGO1 plays roles in both RNA silencing and tasiRNA biogenesis. This discovery prompted an interesting question: Why does only miR390-MpAGO1 lead to tasiRNA production, while other 21-nt miRNAs loaded into MpAGO1 do not induce the production of tasiRNAs from their cleaved target RNA? We propose two potential explanations. First, this might be attributed to the ‘two-hit and two-cleave’ model, as a typical miRNA-MpAGO1 cleaves at one position, whereas miR390-MpAGO1 cleaves at two positions. Second, the inherent characteristics of the TAS3 sequence, such as its secondary structure, might predispose it to tasiRNA production. Regardless of which hypothesis is correct, both M. polymorpha and Arabidopsis can serve as valuable models for further investigations in the future. In addition to the aforementioned differences, the way in which MpAGO1 accommodates small RNA differs from that of AtAGO1. AtAGO1 prefers tasiRNAs and miRNAs that have a 5ʹ-end 1st uridine (Mi et al. 2008). The insights from the MpAGO1-IP dataset indicate that other tasiRNAs incorporate other purines and pyrimidines at their 5ʹ-end 1st position. This finding that MpAGO1 offers broader and more varied abilities in small RNA selection than AtAGO1.
According to the promoter-citrine-NLS assay, miR390 was distinctly expressed in the two apical notches of gemma. MpTAS3 expression in gemma was weaker, primarily in the gemma cup tissue. Furthermore, MIR390/11700OE plants exhibited a variation in the number of gemma cups. The gemmae and gemma cup are specialized gametophytic organs of the Marchantiopsida species that are not found in angiosperms. Recent molecular research has revealed that the development of gemma and gemma cups in M. polymorpha shares regulatory modules common to other land plants (Kato et al. 2020). This finding implies that the miR390/MpTAS3/miR11700 regulatory module also plays a crucial role in developing gemma, representing a shared component of these common regulatory pathways. Additionally, we observed that MIR11700 and MpCYP78A101 were both specifically expressed in the apical notch and antheridium, indicating that the miR390-tasi78A-miR11700 regulation of MpCYP78A101 is closely related to apical notch development and reproductive processes in M. polymorpha.
The tasi78A is the most abundantly produced MpTAS3 tasiRNA and has the highest content in MpAGO1. The tasi78A belongs to the reversed and complemented sequence of tasiAP2, which is a highly conserved tasiRNA in non-vascular plants (Morozov et al. 2018). Hence, tasi78A is also a conserved tasiRNA in bryophytes. Our study showed that tasi78A can coordinately regulate MpCYP78A101 expression with miR11700. In non-vascular plants, the CYP78 family of M. polymorpha, C. purpureus and P. patens all have the tasi78A cleavage site; however, the vascular plant CYP78 gene family does not have this cleavage site, indicating that tasi78A specifically regulates the CYP78 gene family in non-vascular plants. However, miR11700 only specifically regulates MpCYP78A101, and no miR11700 target site is found in the CYP78 gene family of other non-vascular plants that show the unique dual regulation of MpCYP78A101 by tasi78A and miR11700.
To study the molecular mechanisms by which these small RNAs regulate MpCYP78A101, CRISPR gene editing and gain-of-function strategies were employed. However, evolutionary analysis revealed an unreported gene, Mp1g14150, in M. polymorpha, which is a paralog of MpCYP78A101. Therefore, morphological changes in these small RNA mutants or transgenic plants are not very pronounced, likely due to the functional redundancy of the Mp1g14150 gene, even though MpCYP78A101 expression is affected in those plants. Indeed, the CYP78A subfamily has undergone considerable duplication and expansion with functional retendering during the evolution from bryophytes to angiosperms (Ito and Meyerowitz 2000, Wang et al. 2008, Katsumata et al. 2011, Vasav and Barvkar 2019, Zhang et al. 2020). However, slight morphological changes in the thallus and sexual organs of the mir11700ge mutant and MIR11700OE plants suggest that the timing and region of miR11700 may affect genes other than MpCYP78A101.
Notably, this study used two new techniques. First, amiRNA technology was used to specifically express tasi78A (Niu et al. 2006, Flores-Sandoval et al. 2015). Second, we used transient expression by agropenetration to verify that these small RNAs regulate MpCYP78A101. By specifically expressing tasi78A using the miR160 precursor, we successfully reduced endogenous MpCYP78A101 expression, consistent with the results obtained for the MIR390/11700OE plants. In addition, through the use of agropenetration to transiently express 78ARepYFP or 11700RepYFP reporters in the young thalli of amiR-TASI78AOE and MIR390/11700OE plants, respectively, we observed reduced YFP fluorescence, which confirmed that these small RNAs are involved in the regulation of MpCYP78A101.
On the other hand, we will face some challenges while overexpressing the TAS3 locus. An excess of the TAS3 transcript might not be cleaved entirely by the limited amount of endogenous miR390-AGO1, which is a limiting factor. Moreover, the overexpression of TAS3 might result in the expression of additional tasiRNAs, possibly leading to side effects, which could obscure the precise regulatory relationship between tasi78A and MpCYP78A101. We found that agropenetration requires a young thallus as a material and works best with the Agrobacterium strain GV2260. Transient fluorescence is often limited to younger tissue near the apical notch, while the mature/basal area shows slight fluorescence. This heterogeneity poses challenges for qRT-PCR quantification, so we recommend using a confocal microscope to observe specific regions as a standard and calculating fluorescence intensity to overcome these inconsistencies. Similar issues arise when observing the loss-of-function effect of tasi78A and miR11700 on MpCYP78A101. The gemma is the preferred material for observing a slight increase in endogenous MpCYP78A101, likely due to its localized expression in the apical notch area. In summary, amiRNA and agropenetration techniques reinforce and confirm the regulatory roles of tasi78A and miR11700 in controlling the expression of MpCYP78A101.
The dual regulation of MpCYP78A101 by tasi78A and miR11700 in bryophytes is not unique. In P. patens, Ppt-tasiAP2 and Ppt-miR529 jointly regulate the expression of PpAP2 (Cho et al. 2012); Ppt-tasiARF and Ppt-miR1219 both regulate PpARF (Axtell et al. 2007). Together, Ppt-TAS3c tasiRNA and miR1217 control PpDCL1 (Arif et al. 2022). In M. polymorpha, miR11707.1 and miR11707.2 co-regulate MpAGO1 (Lin et al. 2016, Lin and Bowman 2018). These examples demonstrate that bryophytes employ a distinctive dual regulatory mechanism to control essential genes. Thus, we speculate that MpCYP78A101 is also an essential gene in M. polymorpha development that requires precise regulation.
Moreover, observations of promoter fluorescence indicate that compared with the MIR390 promoter construct, MpCYP78A101 exhibits more robust fluorescence in the apical notch, while the MIR11700 promoter has weaker fluorescence in the apical notch. Additionally, the MIR11700 promoter results in more noticeable phenotypes in developing thalli and sexual organs. Therefore, we cannot rule out the possibility that tasi78A and miR11700 might have temporal and spatial differences in regulating MpCYP78A101.
Many studies suggest that CYP78A may be involved in the accumulation and distribution of auxin. Indeed, an auxin-less sensitive phenotype was observed for the dcl4ge and mir390/11700ge mutants treated with 0.5 μM NAA. In contrast, when treated with the same concentration of NAA, amiR-TASI78AOE plants displayed an extremely sensitive phenotype to auxin, indicating that MpCYP78A101 is likely involved in the suppression of the auxin-signaling pathway. Notably, we excluded the involvement of MpARF2 in the morphological changes observed in these NAA-treated plants, as MpARF2 did not exhibit significant differential expression in these plants. Additionally, based on the degradome and MpAGO-IP profiles, the evidence for the tasiARF–MpARF regulatory relationship in the vegetative stage is insignificant and cannot be conclusively demonstrated in this study. Nevertheless, the various MpTAS3-related regulatory mutants, transgenic plants and advanced amiRNAs used in this study provide a foundation for further investigations into the tasiARF–MpARF interaction using the amiR-tasiARF approach. Interestingly, in Arabidopsis, tasiARF derived from AtTAS3 can regulate AtARF3 and AtARF4, both of which are involved in the suppression of the auxin-signaling pathway (Fig. 11A). Coincidentally, in M. polymorpha, tasi78A produced from MpTAS3 can coordinately regulate the expression of MpCYP78A101 with miR11700, thereby modulating the auxin-signaling pathway (Fig. 11B), especially in the differentiation of the apical notch. Indeed, Eklund et al. (2015) demonstrated that auxin is synthesized at the apex of the thallus through a tryptophan-dependent IPyA pathway and is transported from the apex to the base (Maravolo 1976, Gaal et al. 1982), which regulates the development of the thallus of M. polymorpha. Therefore, we hypothesize that the apical notch of gemma may also contain a high content of auxin, which affects its development.
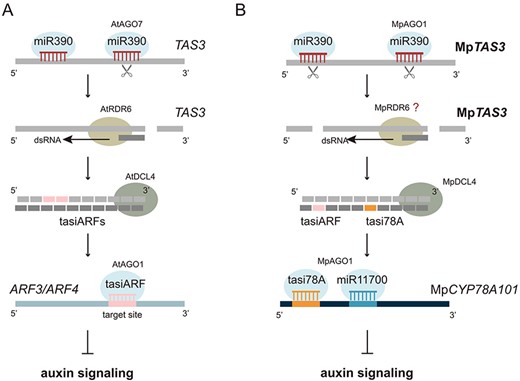
Illustration of the miR390/TAS3 regulatory module in Arabidopsis and M. polymorpha.
Conclusion
This study confirmed that miR390/MpTAS3 tasiRNA biogenesis is carried out through MpAGO1 and MpDCL4. We also discovered a new tasi78A variant that can coordinately regulate the expression of MpCYP78A101 with miR11700 in the apical notch meristem of gemma and sexual organ production. Unexpectedly, we found that MpCYP78A101 might be involved in regulating auxin signaling. In summary, miR390/MpTAS3 module-derived tasi78A coordinately regulates MpCYP78A101 with miR11700 for RNA silencing, which provides new insights into how MpCYP78A101 regulates auxin signaling and sexual organ production.
Materials and Methods
Plant materials and growth conditions
Tak-1 (male) and Tak-2 (female) M. polymorpha plants were cultured in half-strength Gamborg’s B5 medium supplemented with 1.0 ∼ 1.2% agar. The asexual growth of plants was maintained under continuous light at 50–60 μmol photons m−2 s−1 at 20°C. For reproductive growth induction, mature gemma were transferred to white light conditions and supplemented with far-red light (30 μmol photons m−2 s−1).
Gene construction
Guide RNAs (gRNAs) were designed by using the CasFinder tool of MarpolBase (https://marchantia.info/tools/casfinder/). The primer sets containing the BsaI restriction site were used for gRNA cloning and ligated to the entry vector pMpGE_En03 (Sugano et al. 2018). To overexpress the MpMIRs, the precursors of miR390 and miR11700 were amplified and cloned into pENTR/D-TOPO (Invitrogen, Carlsbad, Ca, USA). For artificial miRNA construction, we followed the standard protocol (Stemmer et al. 1995) to assemble amiR-TASI78A within the miR159 precursor of Arabidopsis (agroinfiltration in N. benthamiana) or the miR160 precursor of M. polymorpha (agropenetration in M. polymorpha). For the reporter assay, we amplified a 120-nt fragment of target DNA containing the miRNA target site (78ARepYFP, 11700RepYFP, and 11689RepYFP) and cloned it into the pENTR/D-TOPO vector (Invitrogen). The entry vector with gRNA was subsequently transformed into the pMpGE010 or pMpGE011 binary vector (Sugano et al. 2018), and the pENTR vectors with the precursor of miRNA or amiRNA were further transformed into the pMpGWB103 or pMpGWB303 (Ishizaki et al. 2015) or pBA-DC-myc binary vector via the LR Clonase (Invitrogen). The reporter clones were further subcloned into pBA-DC-YFP vector through an LR reaction. MIR390, MpTAS3, MIR11700 and MpCYP78A101 promoter-reporter constructs were constructed by amplifying 5 ∼ 5.5 kb of the sequence upstream of the transcription start site of the target gene. The promoter regions of MIR390, MpTAS3, MIR11700 and MpCYP78A101 were amplified from M. polymorpha gDNA, ligated into the entry vector pENTR1a and recombined into the pMpGWB115 (Citrine-NLS reporter) vector (Ishizaki et al. 2015). All primer sets used in gene construction can be found in Supplementary Table S1.
Plant transformation and gene knock-out
Agrobacterium-mediated transformation of M. polymorpha was conducted following the thallus transformation method (Kubota et al. 2013). The Agrobacterium strain GV2260 harboring a binary vector (pMpGE010-gRNA-miR390, miR11700 or dcl4) was used to generate the mir390ge, mir11700ge and dcl4ge mutants. The mir390ge mutant line #21 was used as a genetic background to generate the mir390/11700ge double mutant. All the gRNAs were designed based on the PAM site on the M. polymorpha genome sequence and are listed in Supplementary Table S1. In addition, a binary vector (pMpGWB103-MIR390 or MIR11700) was used to construct the MIR390OE and MIR11700OE plants. After cocultivation with Agrobacterium, the transformants were washed with sterile water three–five times and selected on medium supplemented with 100 mg/ml cefotaxime and antibiotics (10 mg/ml hygromycin or 0.5 μM chlorsulfuron) depending on the selection of the marker in the binary vector. Regenerating transformants (T1) were transferred to a new selective medium until gemmae (G1) was produced. Further asexual generation from G1 was used for all the experiments performed in this study.
Small RNA detection
Northern blot analysis was conducted for sRNA detection. Total RNA (10–20 µg) was separated on a 15% polyacrylamide/1 × TBE/8 m urea gel and transferred to a Hybond-N+ membrane. The antisense DNA probes for miRNA were end-labeled with [γ-32P] ATP using T4 polynucleotide kinase (New England Biolabs, Ipswich, MA, USA). A Mimi Quick Spin Oligo Column (Roche, Basel, Switzerland) was used to purify the free radioisotopes. The UV cross-linked membrane was prehybridized with Ambion ULTRAhyb®-Oligo hybridization buffer (Invitrogen, Carlsbad, Ca, USA) for 1 h at 42°C. Then, the labeled probe was added to the hybridization buffer to hybridize the immobilized RNA for 16 h at 42°C, and the signal was detected using X-ray film (GE Healthcare, Chicago, IL, USA) at 80°C for 16 h to 7 days, depending on the miRNA expression level.
MpAGO1-IP
For the MpAGO-IP experiment, homemade polyclonal antibodies against MpAGO1 (α-MpAGO1) were utilized at a working concentration of 1 mg/ml. Prior to conducting MpAGO1-IP, a mixture of 15 μl of IgG and 30 μl of protein A beads (Bio-Rad, Hercules, CA, USA) was mixed and pre-incubated for a minimum of 30 min. Fresh 0.5 g fresh samples were ground into a fine powder and then extracted using 500 μl of AGO1-IP buffer (composed of 50 mM Tris-HCl, pH 7.5, 150 mM NaCl, 5 mM MgCl2, 0.1% Nonidet P-40 and 10% glycerol). The tissue lysate was centrifuged at 13,000 rpm for 10 min, followed by a 2-h supernatant incubation with pre-cultured IgG beads at 4°C. The MpAGO1 precipitate was washed with AGO1-IP buffer twice and resuspended in 1× PBS buffer. The IP products were further subjected to RNA extraction using TRIzol reagent (Invitrogen) to construct a cDNA library.
Small RNA deep sequencing
Thirty-day-old thalli of the wild-type Tak-1, mir390ge mutant, MIR390OE plant and dcl4ge mutant were used for cDNA library construction. The sRNAs from 0.2 g of fresh thalli were extracted with a miRNA isolation kit (Geneaid, Taipei, Taiwan) and used for cDNA library construction following the manufacturer’s instructions for the RNA Library Prep Kit (Invitrogen Thermo Fisher Scientific). The sRNA sequencing was accomplished through single-read (1 × 50) strand-specific HiSeq sequencing (Illumina, San Diego, Ca, USA) by next-generation sequencing (NGS) at the High Throughput Genomics Core of Academia Sinica. The raw reads were trimmed to filter out the adapters and the low-quality reads by using CLC Genomic Workbench (Qiagen, Hilden, Germany). The expression of miRNAs of interest was normalized by counting reads per million (RPM) in different samples. The metadata of the cellular and MpAGO1-IP profiles were deposited in the National Center for Biotechnology Information (NCBI) under the SRA accession numbers SRR27497907, SRR27497909, SRR27497905 and SRR27497906, respectively.
Promoter-reporter assays
Sporelings (for MIR390 and MpTAS3) or thalli (for MIR11700 and MpCYP78A101) were transformed with Agrobacterium strain GV2260 harboring the binary vectors. Next, the transformants were selected with 10 mg/l hygromycin. The Critrine-NLS signal in the G2 generation of transformants was detected via a Leica TCS SP5 confocal microscope (TechComm, College of Life Science, National Taiwan University).
Small RNA target identification
The candidate sRNAs and Tak-1 mRNA file (v5.1) were employed as queries for identifying potential miRNA targets using psRNAtarget with the default settings from the 2017 release. The prediction outcomes were subsequently cross-referenced with the degradome profile. Potential target sites were determined based on substantial degradome reads, specifically the specific accumulation of more than 20 reads at the 10th and 11th positions of the predicted site.
Real-time RT–PCR and stem–loop qRT-PCR
qRT-PCR was performed to detect the expression level of mRNA. The obtained total RNA was treated with TURBOTM DNase (Ambion) to remove genomic DNA contamination. Then, first-strand cDNA was synthesized by SuperScriptTM III Reverse Transcriptase (Invitrogen). The primer sets 5ʹ- GAAGTTCCAGCAATCGTCGG-3ʹ and 5ʹ-GCGGTGTGAGATGTAGCAAG-3ʹ were used for the detection of MpARF2. The primer sets 5ʹ-CTCGACTCTCAACGAGCAAG-3ʹ and 5ʹ-AGCCCAAAACAGAAGCAGAA-3ʹ and 5ʹ- ATTGCTCTGTGGAAGTTTGAGACT-3′ and 5ʹ- GTGGTTGAGTCGATGATGAGAAC-3ʹ were used to amplify MpCYP78A101 and the internal control gene EF1α, respectively. qRT-PCR was performed using the KAPA SYBR FAST qPCR kit (KAPA Biosystems, Wilmigton, MA, USA) in a Bio-Rad CFX real-time PCR machine (Bio-Rad). Three biological replicates and three technical repeats were included in the qRT-PCR assay.
Stem–loop qRT-PCR was performed to detect the expression levels of mature miRNAs. Stem–loop RT primers bind to the 3ʹ portion of miRNA molecules and are reverse-transcribed by SupersucriptTM IV (Invitrogen). Next, the RT product was quantified using the miRNA-specific forward primer and a universal reverse primer with the universal probe library (UPL) system. The RT primers 5ʹ- GTTGGCTCTGGTGCAGGGTCCGAGGTATTCGCACCAGAGCCAACGACGCT-3ʹ and 5ʹ-GTTGGCTCTGGTGCAGGGTCCGAGGTATTCGCACCAGAGCCAACAAAAAT-3ʹ were used for reverse transcription of MpmiR390 and MpU6, respectively. Then, the primer 5ʹ- GTTGAAGCTCAGGAGGGAT-3ʹ and the universal reverse primer 5ʹ- GTGCAGGGTCCGAGGT-3ʹ were used for the amplification of MpMIR390. The primers 5ʹ-GATACAGAGAAGATTAGCATGG-3ʹ and the universal reverse primer 5ʹ- GTGCAGGGTCCGAGGT-3ʹ were used to amplify the internal control MpU6.
Transient expression by agroinfiltration
The Agrobacterium tumefaciens strain ABI harboring the expression binary vector was incubated in LB medium supplemented with 100 μg/ml spectinomycin and 50 μg/ml kanamycin for 24 h at 28°C. Then, the cultures were subcultured at 1/10 volume in LB medium supplemented with 10 mM MES (pH 5.6), 40 µM acetosyringone, 100 μg/ml spectinomycin and 50 μg/ml kanamycin and incubated at 28°C for 16 h. The Agrobacterium were collected by centrifugation, and the resulting pellet was resuspended in inoculation buffer (ddH20 supplemented with 10 mM MgCl2 and 150 µM acetosyringone). For the appropriate infection conditions, the absorbance of the inoculation buffer with Agrobacteria was adjusted to OD600 = 1.0 and the cultures were incubated at room temperature for 3 h (Lin et al. 2013). Next, the Agrobacteria containing the MpMIR gene or target fragment fused with YFP were mixed equally and co-infiltrated into 4-week-old N. benthamiana plants. After 2- or 3 days of culture, the YFP fluorescence of the infiltrated leaves was detected by using a Leica TCS SP5 II confocal laser-scanning microscope (Joint Center for Instruments and Research, College of Bioresources and Agriculture, National Taiwan University), and the expression level of the miRNAs was validated by northern blotting.
Transient expression by agropenetration in M. polymorpha
Iwakawa et al. (2021) reported a transient transformation method for M. polymorpha, we referred to a pervious study (Iwakawa et al. 2021) and used 14-day-old thalli as the material, with some optimization based on our experimental needs. First, we utilized the Agrobacterium strain GV2260 for the transformation. Second, we substituted 2% sucrose in all media with 2% glucose to improve the transformation efficiency. Third, the pH of the co-culture medium was adjusted to 5.2 –5.3 to increase the transformation efficiency. Fourth, the Agrobacterium infection concentration for M. polymorpha was adjusted according to different transformation vectors: the concentrations for 78ARepYFP and 11689RepYFP were diluted to be at OD600 = 0.02 and that for 11700RepYFP was diluted to be at OD600 = 0.04. The coculture time was 3 days, during which vacuum penetration was applied on the first day (two times for 5 min each) and the second day (two times for 3 min each) to facilitate Agrobacterium entry into M. polymorpha. Notably, the construct expressing 11689RepYFP without the target site was used as an internal control. The YFP signals of transformed thalli were detected using a Leica TCS SP5 II confocal laser-scanning microscope (Joint Center for Instruments and Research, College of Bioresources and Agriculture, National Taiwan University).
Auxin sensitivity assay
For the assessment of auxin sensitivity, 9 gemmae per plate of WT and gemma from dcl4ge mutant, mir390ge mutant, mir390/11700ge mutant and amiR-TASI78AOE plants were cultured on plates on 1/2 Gamborg’s B5 media supplemented with/without 0 μM, 0.25 μM or 0.5 μM NAA for a duration of 14 days. Photographic records of the plates were captured, and plant categorization was performed at the end of the 14-day period. The thallus area was collected by quantifying the pixel values. The ratio of the cell area (with NAA/without NAA) was calculated and classified as extremely sensitive (ratio < 0.025), sensitive (ratio 0.025–0.2) or less sensitive (ratio > 0.2) to reflect the effects of NAA on plant growth. The percentage of thalli for various plants in the three levels of the NAA effect was calculated.
Cell size quantification
Staining gemma with fluorescein diacetate (FDA) was conducted according to the field protocol established by Westermann et al. (Westermann et al. 2020). Following staining, the gemmae were examined via fluorescence microscopy, and images of the cells were captured. Specifically, epidermal cells in the central region of the gemma (approximately 31–129 cells/gemma) were manually identified and delineated. The area of these cells was then quantitatively analyzed from 9 to 12 gemmae by measuring the pixel values in the image.
Phylogenetic tree of the CYP78 and CYP98 families
We searched for the 47 homologous genes of CYP78 and CYP98 from the MarpolBase and Phytozome (https://phytozome-next.jgi.doe.gov/) databases across the genomes of Ceratodon purpureus, Physcomitrium patens, Ceratopteris richardii, Diphasiastrum complanatum, Amborella trichopoda, Oryza sativa and Arabidopsis thaliana. Multiple sequence alignment of these CYP78 and CYP98 genes was performed using the Muscle algorithm with default parameters in MEGAX software (Kumar et al. 2018). The resulting phylogenetic tree was constructed using both neighbor-joining (NJ) (Tamura et al. 2004) and maximum likelihood (ML) algorithms with default settings. Bootstrapping (1000 replicates) was applied for both the ML and NJ analyses. The phylogenetic trees obtained through the ML and NJ methods are highly similar; hence, we present the ML tree in our findings.
Supplementary Data
Supplementary Data are available at PCP online.
Data Availability
Supplementary data are available at PCP online.
Funding
National Science and Technology Council, Taiwan (NSTC112-2321-B-005-008 and NSTC112-2311-B-002-024-MY3).
Acknowledgments
The authors thank Prof. Takayuki Kohchi (Kyoto University) and Prof. Ryuichi Nishihama (Tokyo University of Science) for sharing the CRISPR-Cas technology for gene knock-out on plant materials. We also thank the NGS High Throughput Genomics Core, Biodiversity Research Center, Academia Sinica, for the deep sequencing of small RNAs. We also appreciate Dr. Rui Sun (Kyoto University), who supported the vectors in the overexpression, gene editing and promoter assays of M. polymorpha.
Author Contributions
Y.L.H., Z.J.P., S.F.H., W.L.W., V.T., Q.Y.Y., J.Z.Y., S.C., and Y.C.C. conducted the experiments and performed the data analysis; Y.H.C., H.C.Y., and M.Y.J.L. performed the NGS of the small RNA. R.N. and T.K. supported the gene editing techniques. Y.L.H., T.K., R.N., C.N.W., and S.S.L. conceived and designed the study; Y.L.H., Z.J.P., S.F.H, C.N.W., J.B., and S.S.L. wrote the manuscript. All the authors reviewed and edited the manuscript and approved the final version.
Disclosures
The authors have no conflict of interest to declare.