-
PDF
- Split View
-
Views
-
Cite
Cite
Zhonghui Feng, Xiaohan Liang, Hongtao Tian, Yasuko Watanabe, Kien Huu Nguyen, Cuong Duy Tran, Mostafa Abdelrahman, Kun Xu, Mohammad Golam Mostofa, Chien Van Ha, Keiichi Mochida, Chunjie Tian, Maho Tanaka, Motoaki Seki, Zhengwei Liang, Yuchen Miao, Lam-Son Phan Tran, Weiqiang Li, SUPPRESSOR of MAX2 1 (SMAX1) and SMAX1-LIKE2 (SMXL2) Negatively Regulate Drought Resistance in Arabidopsis thaliana, Plant and Cell Physiology, Volume 63, Issue 12, December 2022, Pages 1900–1913, https://doi.org/10.1093/pcp/pcac080
- Share Icon Share
Abstract
Recent investigations in Arabidopsis thaliana suggest that SUPPRESSOR of MORE AXILLARY GROWTH 2 1 (SMAX1) and SMAX1-LIKE2 (SMXL2) are negative regulators of karrikin (KAR) and strigolactone (SL) signaling during plant growth and development, but their functions in drought resistance and related mechanisms of action remain unclear. To understand the roles and mechanisms of SMAX1 and SMXL2 in drought resistance, we investigated the drought-resistance phenotypes and transcriptome profiles of smax1 smxl2 (s1,2) double-mutant plants in response to drought stress. The s1,2 mutant plants showed enhanced drought-resistance and lower leaf water loss when compared with wild-type (WT) plants. Transcriptome comparison of rosette leaves from the s1,2 mutant and the WT under normal and dehydration conditions suggested that the mechanism related to cuticle formation was involved in drought resistance. This possibility was supported by enhanced cuticle formation in the rosette leaves of the s1,2 mutant. We also found that the s1,2 mutant plants were more sensitive to abscisic acid in assays of stomatal closure, cotyledon opening, chlorophyll degradation and growth inhibition, and they showed a higher reactive oxygen species detoxification capacity than WT plants. In addition, the s1,2 mutant plants had longer root hairs and a higher root-to-shoot ratio than the WT plants, suggesting that the mutant had a greater capacity for water absorption than the WT. Taken together, our results indicate that SMAX1 and SMXL2 negatively regulate drought resistance, and disruption of these KAR- and SL-signaling-related genes may therefore provide a novel means for improving crop drought resistance.
Introduction
Karrikins (KARs) are chemicals that were originally identified from smoke water and shown to stimulate seed germination in >1,000 plant species (Flematti et al. 2004, 2009, Nelson et al. 2012). Numerous investigations have also revealed that exogenous applications of KARs and smoke water promote the cotyledon expansion and greening (Nelson et al. 2010), seedling development (Nelson et al. 2012) and seedling vigor under abiotic stresses, such as high temperature, high salinity and low osmotic potential (Ghebrehiwot et al. 2008, Soos et al. 2009, Jamil et al. 2014). The multifaceted roles of KARs in a wide variety of angiosperms suggest that they coordinate important regulatory mechanisms in plants.
The KAR-signaling pathway of Arabidopsis thaliana (Arabidopsis) has been characterized through forward genetic screening of mutants. Transcriptome analyses have indicated that KARs promote Arabidopsis seed germination by enhancing sensitivity to light in a gibberellin-dependent manner (Nelson et al. 2009). The first KAR-signaling component was identified from a mutant screening of seeds whose germination was insensitive to KAR. This work led to the identification of MORE AXILLARY GROWTH 2 (MAX2), which encodes an F-box protein that participates in KAR signal transduction (Nelson et al. 2011) and was originally known for its role in strigolactone (SL) signaling (Umehara et al. 2008, Nelson et al. 2011). A second KAR-signaling component identified from another round of mutant screening was KAR INSENSITIVE2 (KAI2)/HYPOSENSITIVE TO LIGHT (HTL) (Sun and Ni 2011, Waters et al. 2012), which encodes an α/β-hydrolase that is an ancient paralog of the SL receptor DWARF14 (D14) (Yao et al. 2016). These results strongly suggest that KAR- and SL-signaling components are very similar, or even shared, and this conclusion is also supported by the similar chemical structures of KARs and SLs, both of which possess a butanolide ring (Conn and Nelson 2016). A third KAR-signaling component was identified from a screen for genetic suppressors of the hypocotyl elongation phenotype of max2 plants and was named SUPPRESSOR OF MAX2 1 (SMAX1) (Stanga et al. 2013). Later developmental analyses showed that SMAX1 and its homolog SMAX1 LIKE 2 (SMXL2) suppress KAR signaling and show partially redundant functions in plant growth and development (Stanga et al. 2013, 2016). As KARs are not endogenous chemical molecules, KAI2 is also assumed to recognize an as-yet-unknown endogenous molecule, the so-called KAI2 ligand (KL) (Conn and Nelson 2016).
Although there are more lines of biochemical evidence for the SL signal transduction pathway than for that of KARs, we can nonetheless summarize a proposed KAR signal transduction pathway based on available genetic evidence. In brief, KARs, their metabolites, or KL may bind to and activate KAI2, a scenario supported by the results of equilibrium microdialysis assays (Guo et al. 2013). The activated form of KAI2 may interact with MAX2 to form a KAI2–Skp1–Cullin–F-box (KAI2-SCF)MAX2 complex, which then polyubiquitinates SMAX1 and SMXL2, triggering their degradation by the 26S proteasome (Stanga et al. 2016, Khosla et al. 2020, Wang et al. 2020, Zheng et al. 2020). The degradation of SMAX1 and SMXL2 leads to the expression of downstream genes, thereby activating a series of biological processes (Stanga et al. 2016, Khosla et al. 2020, Wang et al. 2020).
A great progress has been made in understanding the functions of KAR/KL signaling in plant growth and development by observing the phenotypes of kai2 and smax1 smxl2 (s1,2) mutant plants. In addition to promoting seed germination, KAR/KL signaling also inhibits hypocotyl elongation in the light (Nelson et al. 2010, Waters et al. 2012), influences leaf morphology (Sun and Ni 2011), regulates root skewing (Swarbreck et al. 2019, Villaecija-Aguilar et al. 2019), and promotes root hair density and elongation in Arabidopsis (Villaecija-Aguilar et al. 2019, 2022, Carbonnel et al. 2020). In rice (Oryza sativa), recent investigations suggest that KAR/KL signaling also promotes arbuscular mycorrhizal symbiosis (Gutjahr et al. 2015) and inhibits mesocotyl elongation in the dark through its interaction with SL signaling (Zheng et al. 2020). The role of KAR/KL signaling in the mycorrhizal symbiosis has also recently been confirmed in Lotus japonicus (Carbonnel et al. 2020). In addition, several reports have shown that KAR/KL- and SL-signaling pathways positively regulate drought resistance based on comparative analyses of drought-resistance phenotypes of kai2, d14 and d14 kai2 plants (Li et al. 2017, 2020b), as well as smxl6,7,8 (SMXL6, 7 and 8 are homologs of SMAX1 and SMXL2 and are suppressors of the SL signaling) plants (Yang et al. 2020, Li et al. 2020c). A very recent investigation indicated that SMAX1 and SMXL2 were also involved in SL-signaling pathway through D14 and involved in osmotic stress, suggesting an overlap between the SL- and KAR-signaling pathways (Li et al. 2022). However, the mechanisms by which SMAX1 and SMXL2 function in drought resistance remain unknown (Li et al. 2022).
Here, we set out to characterize the mechanisms by which SMAX1 and SMXL2 participate in the regulation of drought resistance through phenotypic, physiological, transcriptomic and morphological analyses of the s1,2 mutant and WT Arabidopsis plants. We found that the s1,2 mutant plants showed enhanced drought resistance and lower leaf water loss when compared with WT plants. These traits were associated with increases in cuticle formation, stomatal closure, abscisic acid (ABA) response, reactive oxygen species (ROS)-scavenging capacity, root-to-shoot ratio and root hair development in the s1,2 mutants, which may be explained by changes in the expression of related genes as assessed by a transcriptomic study. These results suggest that SMAX1 and SMXL2 negatively regulate drought resistance through a number of mechanisms.
Results
The s1,2 mutant is more resistant to drought than the WT
To compare the drought-resistance levels of the s1,2 mutant and WT plants, we transferred 2-week-old agar-grown seedlings to a soil-filled tray and grew them side-by-side for 1 week (Fig. 1A). Water was then withheld for approximately 2 weeks. When symptoms of mortality, such as drying of the stem base, were observed in several seedlings, the trays were re-watered. Three days after re-watering, the s1,2 mutant plants showed a much higher survival rate than the WT plants (Fig. 1B). We also grew plants from both genotypes in parallel without water stress, and the s1,2 mutant and WT plants grew very similarly under well-watered conditions (Fig. 1C). After drought and re-watering, the survival rate of the s1,2 plants was 4.8 times higher than that of the WT plants (Fig. 1D). These results suggested that the s1,2 mutant was more resistant to severe drought stress than the WT. We observed no differences in survival rate in paired comparisons of the smax1 and smxl2 single mutants with WT plants under drought (Supplementary Fig. S1A–D), and we therefore focused on the s1,2 double mutant in subsequent analyses.
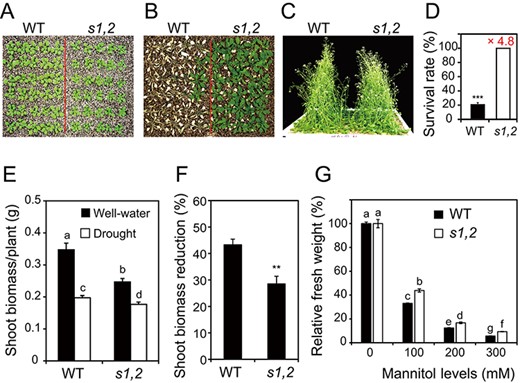
Loss-of-function of SMAX1 and SMXL2 enhances drought resistance. (A) Tray-grown 21-day-old seedlings of the WT and the smax1 smxl2 (s1,2) mutant before drought stress. (B) 21-Day-old WT and s1,2 plants grown under drought stress for 15 d and re-watered for 3 d. (C) 21-Day-old WT and s1,2 plants grown under well-watered conditions for another 18 d in parallel with the drought stress treatment. (D) Survival rates of plants were determined from four independent experiments (n = 4, 30 plants/genotype/experiment). Number above the column indicates the FC in the survival rate of the s1,2 vs. the WT seedlings. (E) Shoot dry weights of WT and s1,2 plants grown under well-watered and mild drought conditions. (F) Percentage of shoot biomass reduction for WT and s1,2 plants grown under mild drought stress (n = 12 plants/genotype/treatment). (G) Relative fresh weights of 5-day-old WT and s1,2 plants grown under different concentrations of mannitol stress for another 2 weeks (n = 12 plants/genotype/treatment). All data are means and SEs. Asterisks show significant differences between the s1,2 mutant and the WT (Student’s t-test; **P < 0.01 and ***P < 0.001). Different letters indicate significant differences (Duncan’s multiple range test; P < 0.05).
To further confirm the enhanced drought resistance of the s1,2 mutant, we next applied a mild drought stress and measured the shoot biomass (dry weight) of s1,2 and WT plants under well-watered and mild drought stress conditions. Under normal conditions, the s1,2 mutant plants had lower shoot biomass than the WT plants, and mild drought stress significantly reduced the shoot biomass of both genotypes (Fig. 1E). However, a comparison of the relative biomass reduction under drought stress and well-watered conditions demonstrated that biomass decreased less in the s1,2 mutant plants than in the WT plants (Fig. 1F). In addition, we also grew the s1,2 mutant and WT plants in agar plates with 0–300 mM mannitol to mimic drought stress. We found that the fresh weight (FW) of both genotypes decreased with increasing mannitol levels (Supplementary Fig. S1E) and that s1,2 mutant plants had a higher relative FW than WT plants under 100–300 mM mannitol osmotic stress conditions (Fig. 1G). This result indicated that the s1,2 mutant was more resistant than the WT to osmotic stress conditions induced by mannitol treatment. Taken together, these results suggest that SMAX1 and SMXL2 negatively regulate plant drought resistance under both severe and mild drought stresses.
Leaf water loss is slower in s1,2 plants than in WT plants
Limiting leaf water loss is an important mechanism of plant drought stress resistance (Fang and Xiong 2015). We therefore measured leaf surface temperatures, which reflect water transpiration rate from leaves. The leaf surface temperatures of s1,2 plants were higher than those of WT plants under well-watered and mild drought stress conditions (Fig. 2A and Supplementary Fig. S2). To confirm the speed of water loss, we measured the relative water contents (RWCs) of rosette leaves from the s1,2 mutant and WT plants during dehydration treatment. The rosette leaves of the s1,2 mutant lost water more slowly than the WT leaves under our laboratory conditions (Fig. 2B). These results indicated that SMAX1 and SMXL2 promote the process of rosette leaf water loss.
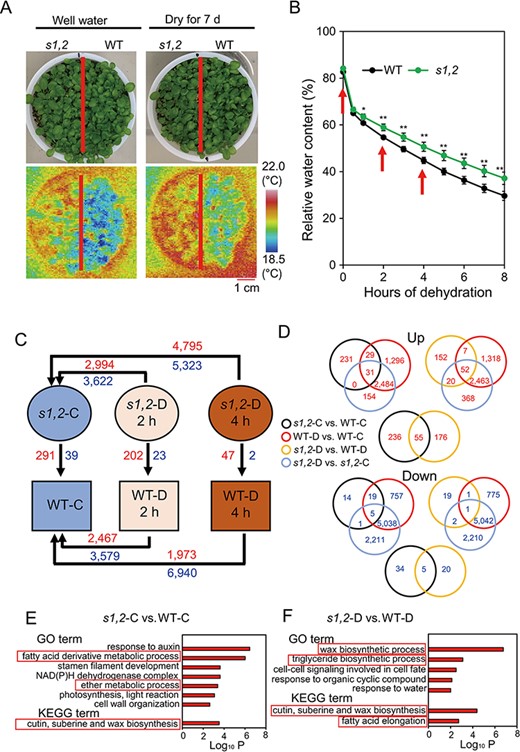
Leaf surface temperatures and comparative transcriptome analysis of the s1,2 mutant and WT plants under well-watered and dehydration conditions. (A) Leaf surface temperature of the 14-day-old s1,2 mutant and WT seedlings grown under well-watered and drought stress conditions. (B) Relative water content of rosette leaves from the 24-day-old s1,2 mutant and WT plants during the 8-h dehydration process (data are means and SEs, n = 5 plants/genotype). Asterisks show significant differences between the s1,2 mutant and the WT (Student’s t-test; *P < 0.05 and **P < 0.01). (C) Numbers of DEGs in different paired comparisons. Red and blue numbers indicate up- and downregulated genes, respectively. (D) Venn diagrams of overlapping and non-overlapping upregulated (red) and downregulated (blue) genes among the different comparisons. (E–F) Enriched terms in upregulated genes from the ‘s1,2-C vs. WT-C’ (E) and ‘s1,2-D vs. WT-D’ (F) comparisons. Enrichment analysis of GO biological process terms and KEGG pathways performed with Metascape. The x-axis shows the log10 cumulative hypergeometric P-values of the genes mapped by the GO or KEGG terms and indicates their abundance. The y-axis shows the enriched GO and KEGG terms. ‘s1,2-C vs. WT-C’, s1,2 well-watered control vs. WT well-watered control; ‘WT-D vs. WT-C’, WT dehydrated 2 h vs. WT well-watered control and/or WT dehydrated 4 h vs. WT well-watered control; ‘s1,2-D vs. s1,2-C’, s1,2 dehydrated 2 h vs. s1,2 well-watered control and/or s1,2 dehydrated 4 h vs. s1,2 well-watered control; ‘s1,2-D vs. WT-D’, s1,2 dehydrated 2 h vs. WT dehydrated 2 h and/or s1,2 dehydrated 4 h vs. WT dehydrated 4 h.
Transcriptome comparison of the s1,2 mutant and the WT under dehydration conditions
To clarify the molecular roles of SMAX1 and SMXL2 in drought responses, we performed a transcriptome analysis of the s1,2 mutant and the WT before and after dehydration. Rosette leaves of the s1,2 mutant and WT plants were sampled at 0, 2 and 4 h after the initiation of dehydration (Fig. 2B–C). The results of the microarray analysis are provided in Supplementary Table S1, and the original data are available under accession number GSE196838.
Differentially expressed genes (DEGs) in different comparisons were identified based on an expression-level fold change (FC) of log2 (FC) > 1 or log2 (FC) < −1 and a false-discovery-rate-adjusted P-value (i.e. q-value) < 0.05. The numbers of DEGs in different comparisons are summarized in Fig. 2C and Supplementary Table S2. Venn diagram analyses indicated that 60 genes were upregulated and 24 genes were downregulated in both s1,2 vs. WT plants under well-watered conditions (s1,2-C vs. WT-C) and dehydrated WT vs. well-watered WT plants (WT-D vs. WT-C) (Fig. 2D, Supplementary Tables S3C, S4G). These genes showed expression changes in response to dehydration in the WT but also differential expression between the s1,2 mutant vs. the WT under well-watered conditions. Intrinsic expression differences in these genes may therefore prepare the s1,2 mutant to better respond to dehydration stress.
In addition, many genes (79) were upregulated and only one was downregulated in the ‘s1,2-D vs. WT-D’, ‘WT-D vs. WT-C’ and ‘s1,2-D vs. s1,2-C’ comparisons (Fig. 2D, Supplementary Tables S3I, S4D). The expression levels of these genes changed in both WT and s1,2 plants under dehydration, but the extent of their differential expression under dehydration was greater in s1,2 plants. Some upregulated (55) and downregulated (5) genes also overlapped between the ‘s1,2-C vs. WT-C’ and ‘s1,2-D vs. WT-D’ comparisons (Fig. 2D, Supplementary Tables S3A, S4E), suggesting that these genes were stably regulated by SMAX1 and SMXL2 under both normal and dehydrated conditions. To confirm the microarray data, the relative expression levels of 25 genes involved in cuticle formation, glucosinolate biosynthesis and hormone biosynthesis and responses (Cui et al. 2016, Li et al. 2017) were assessed by quantitative real-time polymerase chain reaction (qRT-PCR) (Supplementary Fig. S3). The qRT-PCR results were consistent with the results of the microarray data, confirming their reliability.
Enrichment analyses of DEGs suggest that cuticle formation is affected in s1,2 mutant plants
To understand the role of SMAX1 and SMXL2 in drought resistance in a greater detail, we annotated the upregulated DEGs from the comparisons of ‘s1,2-C vs. WT-C’ (Supplementary Table S2M, Q) and ‘s1,2-D vs. WT-D’ (Supplementary Table S2P, T). Specifically, we performed enrichment analyses using Metascape (http://metascape.org) to classify the DEGs into several functional categories based on Gene Ontology (GO) terms and Kyoto Encyclopedia of Genes and Genomes (KEGG) pathways (Supplementary Table S5A–B). The enriched terms in the DEG sets obtained from ‘s1,2-C vs. WT-C’ and ‘s1,2-D vs. WT-D’ comparisons were used for the detailed analysis (Fig. 2E–F and Supplementary Table S5).
Two lipid-related GO terms (‘fatty acid derivative metabolic process’ and ‘ether metabolic process’) and one cuticle formation-related KEGG pathway (‘cutin, suberin and wax biosynthesis’) were enriched in the DEGs derived from the ‘s1,2-C vs. WT-C’ comparison (Fig. 2E and Supplementary Table S5A). These terms are related to leaf surface water loss and drought resistance. Three drought-resistance-related GO terms (‘wax biosynthetic process’, ‘triglyceride biosynthetic process’ and ‘response to water’) and two related KEGG pathways (‘cutin, suberin and wax biosynthesis’ and ‘fatty acid elongation’) were enriched in DEGs from the ‘s1,2-D vs. WT-D’ comparison (Fig. 2F and Supplementary Table S5B). Some cuticle-formation-related and wax-biosynthesis-related genes were included, such as ECERIFERUM 1 (CER1), CER3, CER4, WAX ESTER SYNTHASE AND DIACYLGLYCEROL ACYLTRANSFERASE (WSD1), 3-KETOACYL-COA SYNTHASE 1 (KCS1), GLYCEROL-3-PHOSPHATE SN-2-ACYLTRANSFERASE 4 (GPAT4), CYP86A2, MYB DOMAIN PROTEIN 94 (MYB94), GLYCOSYLPHOSPHATIDYLINOSITOL-ANCHORED LIPID PROTEIN TRANSFER 2 (LTPG2) and ATP-BINDING CASSETTE G11 (ABCG11) (Supplementary Tables S5, S6A). In summary, the ‘cutin, suberin and wax biosynthesis’ term was enriched in both the ‘s1,2-C vs. WT-C’ and the ‘s1,2-D vs. WT-D’ comparisons, suggesting that cuticle formation was affected by SMAX1 and SMXL2 under well-watered and drought stress conditions.
Cuticle formation and stomatal closure are enhanced in the s1,2 mutant
To clarify the effect of SMAX1 and SMXL2 on cuticle formation, we investigated the cuticular permeability of the s1,2 and WT rosette leaves by toluidine blue (TB) staining and chlorophyll leaching assays. Consistent with enrichment of the ‘cutin, suberin and wax biosynthesis’ KEGG pathway in upregulated DEGs from the ‘s1,2-C vs. WT-C’ comparison (Fig. 2E and Supplementary Table S6A), the rosette leaves of the s1,2 mutant showed less TB staining than those of WT plants (Fig. 3A). This result indicated that their cuticular membrane is less permeable to a hydrophilic dye and therefore has better ability to retain water (Tanaka et al. 2004). In addition, the rosette leaves of the s1,2 mutant showed slower chlorophyll leaching than those of WT plants (Fig. 3B), consistent with lower water permeability of the s1,2 mutant through its enhanced cuticle formation. Cuticle formation is associated with wax biosynthesis, and wax crystals are typically deposited on the surfaces of young stems and siliques (Yeats and Rose 2013). We, therefore, observed the surfaces of young stems and siliques of the s1,2 mutant and WT plants by scanning electron microscopy (SEM). As shown in Fig. 3C–D, the s1,2 mutant plants had a higher wax crystal density than the WT plants on the surfaces of young stems (Fig. 3C) and siliques (Fig. 3D). Taken together, these results indicate that SMAX1 and SMXL2 inhibit cuticle formation, wax synthesis and wax crystal formation in Arabidopsis plants.
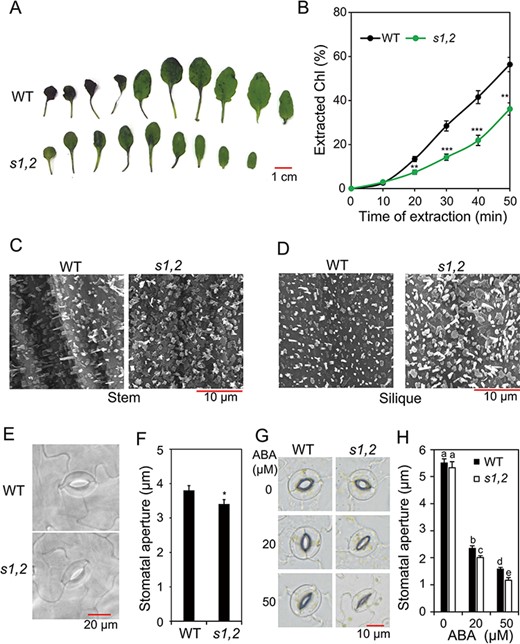
Cuticular permeability and stomatal aperture sizes of the s1,2 mutant and WT plants. (A) Cuticular permeability was detected by TB staining of rosette leaves from the 24-day-old s1,2 mutant and WT plants grown under well-watered conditions. (B) Chl leaching from the rosette leaves of the 24-day-old s1,2 mutant and WT plants grown under well-watered conditions (n = 5 plants/genotype). (C–D) Epicuticular wax crystal formation on stem (C) and silique (D) surfaces of the 28-day-old soil-grown plants examined by SEM. (E–F) Representative guard cells (E) and stomatal aperture sizes (F) from rosette leaves of the 24-day-old s1,2 mutant and WT plants grown under well-watered conditions (n = 5 plants/genotype; two leaves were selected from each plant and 12 stomata were measured on each leaf). (G–H) Representative guard cells (G) and stomatal aperture sizes (H) from rosette leaves of the 24-day-old s1,2 mutant and WT plants grown with different concentrations of ABA (n = 5 plants/genotype, two leaves were selected from each plant, and 15 stomata were measured on each leaf). All data are means and SEs. Asterisks show significant differences between the s1,2 mutant and the WT (Student’s t-test; *P < 0.05, **P < 0.01 and ***P < 0.001). Different letters indicate significant differences (Duncan’s multiple range test; P < 0.05).
In addition to non-stomatal leaf water loss through the cuticle, leaf water balance is strongly affected by water loss through the stomata. We, therefore, investigated the stomatal densities and aperture sizes of rosette leaves in the s1,2 mutant and WT plants. Stomatal apertures were significantly smaller in the s1,2 mutant than in the WT when we measured the stomatal apertures immediately after collecting the leaves from the plants (Fig. 3E–F). However, the stomatal densities of the two genotypes were similar (Supplementary Fig. S4A). These results suggested that SMAX1 and SMXL2 regulate leaf water loss by controlling both cuticle formation and stomatal closure.
ABA responsiveness is enhanced in the s1,2 mutant compared with the WT
We identified several ABA-inducible genes, such as RESPONSIVE TO ABA 28 (RAB28), HVA22 HOMOLOGUE B (HVA22B), HVA22C and ABA-RESPONSIVE ELEMENT BINDING PROTEIN 3 (AREB3) (Supplementary Table S6B−D), among the upregulated DEGs in the ‘s1,2-D vs. WT-D’ comparison. Because ABA responsiveness is well known to be associated with drought resistance (Chen et al. 2020, Gupta et al. 2020), we hypothesized that the ABA responsiveness might be enhanced in s1,2 plants. To test this hypothesis, we measured stomatal aperture in s1,2 and WT plants treated with different levels of ABA. We observed no significant differences in stomatal aperture size between the s1,2 mutant and WT plants when the epidermal peels were incubated in a buffer solution without ABA under high-light conditions for 2 h (Fig. 3G–H). However, in the presence of 20 and 50 μM ABA, stomatal aperture sizes were significantly smaller in the s1,2 mutant than in the WT (Fig. 3G–H), indicating that s1,2 mutant plants were more sensitive to ABA in terms of stomatal closure.
To confirm the greater ABA responsiveness of the s1,2 mutant, we also measured cotyledon opening and seedling FWs of s1,2 and WT plants treated with different concentrations of ABA. In the absence of ABA, the percentages of open cotyledons were comparable between s1,2 and WT plants (Fig. 4A). As ABA concentration increased, the percentages of open cotyledons decreased significantly in both the s1,2 mutant and the WT (Fig. 4A). However, the s1,2 mutant always showed a lower percentages of open cotyledons than the WT in the presence of 0.5, 1 and 2 μM ABA (Fig. 4A). Likewise, although seedling FW was significantly lower in the s1,2 mutant than in the WT in the absence of ABA (Supplementary Fig. S4B), the s1,2 mutant also showed significantly lower relative FWs than the WT in the presence of 0.5, 1 and 2 μM ABA (Fig. 4B–C). We also measured chlorophyll levels and root FWs in both genotypes at different ABA concentrations. In the absence of ABA, chlorophyll levels were significantly higher in s1,2 leaves than in WT leaves, and as ABA concentration increased, chlorophyll levels decreased significantly in both genotypes (Fig. 4D and Supplementary Fig. S4C). However, in the presence of 0.5, 1 and 2 μM ABA, the s1,2 mutant always showed a faster decrease in the chlorophyll content than the WT (Fig. 4E and Supplementary Fig. S4C). Similar results were noted for root FW: the s1,2 mutant showed a lower root FW than the WT in the presence of 1 μM ABA (Fig. 4F–G). Taken together, these data indicated that s1,2 was more sensitive to ABA in terms of stomatal closure, cotyledon opening, seedling growth and ABA-induced senescence, demonstrating that SMAX1 and SMXL2 negatively regulate ABA responsiveness.
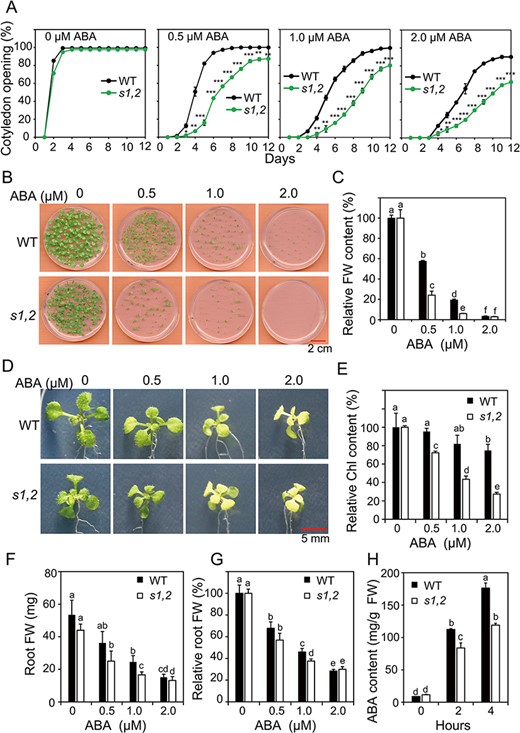
Responsiveness to ABA of the s1,2 mutant and WT plants in terms of cotyledon opening, seedling growth, chlorophyll (Chl) content and root growth and differences in ABA content between the genotypes. (A) Percentage of open cotyledons of the s1,2 mutant and WT seeds grown with different levels of ABA from 0 to 12 d (n = 3, 50 seeds/genotype/experiment). (B) Growth of the s1,2 mutant and WT seedlings in agar with different ABA levels for 12 d. (C) Relative FWs of the s1,2 mutant and WT seedlings grown in agar with different levels of ABA for 12 d (n = 5, 6 seedlings/genotype/treatment). (D-G) Representative pictures of rosette leaves (D), relative Chl levels (E), root FWs (F) and relative root FWs (G) from the 7-day-old s1,2 mutant and WT seedlings grown in agar with different levels of ABA for another 12 d (n = 8 plants/genotype) (H) ABA contents of rosette leaves from the 24-day-old soil-grown s1,2 mutant and WT plants during the dehydration process (n = 5 plants/genotype). All data are means and SEs. Asterisks show significant differences between the s1,2 mutant and the WT (Student’s t-test; *P < 0.05, **P < 0.01 and ***P < 0.001). Different letters indicate significant differences (Duncan’s multiple range test; P < 0.05).
The ABA catabolism gene CYP707A3 was upregulated in the s1,2 mutant relative to the WT under dehydration conditions (Supplementary Fig. S3 and Table S6B), and we were therefore curious about the endogenous levels of ABA in the two genotypes under these conditions. Before dehydration treatment, endogenous ABA levels were comparable between the s1,2 mutant and WT plants (Fig. 4H). As the duration of dehydration increased, endogenous ABA levels rose rapidly in seedlings of both genotypes (Fig. 4H). However, s1,2 mutant plants contained lower levels of ABA than WT plants after 2 and 4 h of dehydration (Fig. 4H). Taken together, these results suggested that SMAX1 and SMXL2 increase ABA accumulation under dehydration conditions and decrease ABA responsiveness at least under normal conditions.
ROS-scavenging capacity is enhanced in the s1,2 mutant compared with the WT
In the microarray data, we found that GLUTATHIONE PEROXIDASE 3 (GPX3) and GPX7 were significantly upregulated in ‘s1,2-D vs. WT-D’ (Supplementary Table S2A, S6C). The GPX genes play important roles in ROS scavenging and signal transduction in response to drought (Chang et al. 2009, Miao et al. 2006). Higher expression levels of GPX genes in the s1,2 mutant than in the WT implied a stronger ability of s1,2 plants to scavenge ROS. To evaluate this hypothesis, we investigated the accumulation of superoxide (O2• −) and hydrogen peroxide (H2O2) using nitro blue tetrazolium (NBT) and 3,3′-diaminobenzidine (DAP) staining of rosette leaves. The rosette leaves of s1,2 plants contained less O2• −and H2O2 than those of WT plants (Fig. 5A–B), suggesting that the s1,2 mutant indeed had a higher ROS-scavenging capacity. To further confirm this result, we applied exogenous paraquat (PQ) and 3-amino-1,2,4-triazole (3-AT) to seedlings of the two genotypes. PQ acts as a source of O2• −, and 3-AT is an inhibitor of catalases (CATs) (Kurepa et al. 1998, Wang et al. 1999), which scavenge H2O2; both chemicals thus induce ROS accumulation in leaves. We found that although PQ and 3-AT treatments decreased the FWs and chlorophyll contents of both s1,2 and WT plants, they caused a faster decrease of FWs in WT plants (especially at 1.0 μM PQ and 30 μM 3-AT) than s1,2 plants and a faster decrease in the chlorophyll content of WT plants (especially at 30 μM 3-AT) than s1,2 plants (Fig. 5C–E). These results indicated that ROS-scavenging capacity was higher in s1,2 plants than in WT plants.
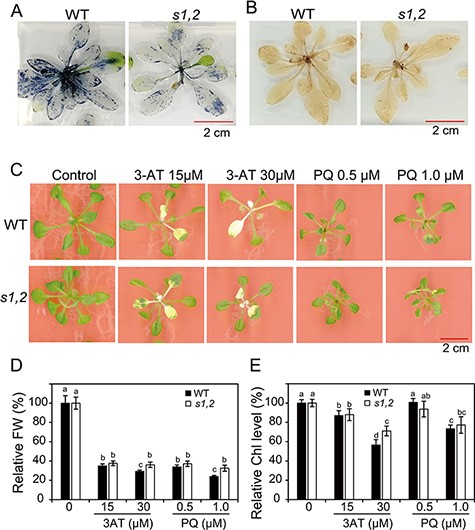
The differential scavenging capacity of ROS between the s1,2 mutant and WT plants. (A) Histochemical analysis of O2• − accumulation by NBT staining of rosette leaves from the 28-day-old s1,2 mutant and WT plants. (B) Histochemical analysis of H2O2 accumulation by DAP staining of rosette leaves from the 28-d-old s1,2 mutant and WT plants. (C) Growth inhibition assays of the s1,2 mutant and WT plants using 3-AT and PQ. Representative pictures of 21-day-old plants are shown. (D–E) Relative fresh weights (D) and relative Chl levels (E) of the 21-day-old s1,2 mutant and WT plants grown on medium with different levels of 3-AT or PQ. All data are means and SEs (n = 12 plants/genotype). Different letters indicate significant differences (Duncan’s multiple range test; P < 0.05).
Root-to-shoot ratio and root hair development are enhanced in the s1,2 mutant
Although the shoots of the s1,2 seedlings were smaller than those of the WT (Figs. 1A, 3A, 4D, 5C), the root systems of the s1,2 mutant and WT plants were comparable (Fig. 6A). As a result, the root-to-shoot ratio was higher in mutant seedlings than in WT seedlings (Fig. 6B). We also observed longer root hairs and higher root hair densities in the mutant than in the WT (Fig. 6C−E), suggesting that the mutant has a greater capacity for water absorption from the soil.
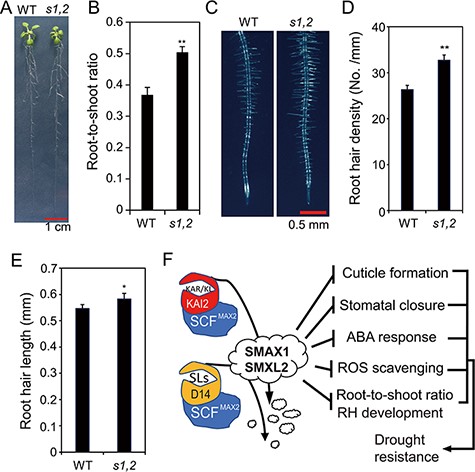
Morphological phenotypes of the s1,2 mutant and WT plants and a proposed mechanistic model of SMAX1 and SMXL2 functions during drought resistance. (A) Representative pictures of the 19-day-old s1,2 mutant and WT seedlings grown in agar. (B) Root-to-shoot ratios of the 19-day-old s1,2 mutant and WT seedlings grown in agar. (C) Representative pictures of 7-day-old root hairs (RHs) of the s1,2 mutant and WT plants grown in agar. (D–E) RH density (D) and RH length (E) of the 7-day-old s1,2 mutant and WT plants grown in agar. All data are means and SEs (n = 12 plants/genotype). Asterisks show significant differences between the s1,2 mutant and the WT (Student’s t-test; *P < 0.05 and **P < 0.01). (F) The proposed mechanistic model of SMAX1 and SMXL2 functions in KAR/KL- and SL-signaling pathways to regulate drought resistance. The binding of KAR/KL with the KAI2 receptor induces the recruitment of the SCFMAX2 E3 ubiquitin ligase complex, which targets SMAX1 and SMXL2 for degradation. Similarly, the binding of SLs with the D14 receptor induces the recruitment of the SCFMAX2 E3 ubiquitin ligase complex, which also targets SMAX1 and SMXL2 for degradation. SMAX1 and SMXL2 function as negative regulators of cuticle formation, stomatal closure, ABA responsiveness, ROS scavenging, root-to-shoot ratio and RH development. Thus, the degradation of SMAX1 and SMXL2 releases these processes, resulting in enhanced drought resistance. Arrows indicate promotion, and blunt bars indicate inhibition.
Discussion
Previous reports have shown that the positive regulator KAI2 of KAR signaling and the positive regulator D14 of SL signaling promote drought resistance in Arabidopsis at different levels by differentially enhancing cuticle formation, stomatal closure, anthocyanin accumulation and ABA responsiveness (Li et al. 2017, 2020b). Recently, a study reported that the negative regulators SMAX1 and SMXL2 of KAR- and SL-signaling pathways inhibit osmotic stress responses in Arabidopsis (Li et al. 2022). However, the mechanisms of action of the negative KAR- and SL-signaling regulators SMAX1 and SMXL2 under drought remain unknown. Thus, in this study, we compared the drought-resistance phenotypes of s1,2 and WT plants and investigated the physiological, transcriptional and morphological differences between s1,2 and WT plants under normal and drought stress conditions to gain a mechanistic insight into the roles of SMAX1 and SMXL2 in Arabidopsis response to drought.
We demonstrated the enhanced drought resistance of s1,2 plants relative to WT plants using three approaches: survival rate under severe drought stress (Fig. 1A–D), biomass mass reduction under mild drought stress (Fig. 1E–F) and response to mannitol-induced osmotic stress (Fig. 1G). Detailed investigations indicated that leaf water loss was lower in s1,2 mutants than in WT plants (Fig. 2B). Leaves can lose water by both stomatal and non-stomatal pathways (Varone et al. 2012). Transcriptome analysis and subsequent GO and KEGG analyses indicated that many cuticle-formation-related and wax-biosynthesis-related genes were significantly upregulated in s1,2 plants relative to WT plants under well-watered and/or dehydration conditions (Fig. 2C–F). These included CER1, CER3, CER4, WAX WSD1, KCS1, GPAT4, CYP86A2, MYB94, LTPG2 and ABCG11 (Supplementary Tables S5, S6A). Such gene expression changes might result in better cuticle integrity on the s1,2 leaf surfaces than that of WT plants as evidenced by the results obtained by TB staining and chlorophyll leaching assays (Fig. 3A–B). Wax has an important function in cuticle formation and prevention of non-stomatal water loss, and high cuticle integrity is typically correlated with enhanced wax biosynthesis (Yeats and Rose 2013). Indeed, we found greater wax crystal deposition on stem and silique surfaces of the s1,2 mutant compared with that of the WT (Fig. 3C–D). These findings suggest that SMAX1 and SMXL2 negatively regulate cuticle formation and wax biosynthesis, thereby promoting leaf water loss through the cuticle and decreasing drought resistance.
In addition to water loss through the cuticle, stomatal water loss is an important means of water transpiration from leaves (Chen et al. 2020). The smaller stomatal apertures of the s1,2 mutant (Fig. 3E–F) were consistent with a reduced water loss in the mutant when compared with that of WT. Stomatal closure is well known to be affected by ABA content and/or ABA signaling (Chen et al. 2020), and we therefore measured the endogenous levels of ABA. There was no significant difference in ABA levels between s1,2 and WT plants under normal growth conditions (Fig. 4E–F). Nonetheless, it remained possible that ABA signal transduction (as indicated by ABA responsiveness) was affected in s1,2 plants. Consistent with this hypothesis, the s1,2 mutant showed greater ABA responsiveness than the WT in terms of stomatal closure (Fig. 3G–H), cotyledon opening (Fig. 4A), seedling and root FW (Fig. 4C, F) and ABA-induced leaf senescence (Fig. 4D–E). In addition, the expression of several genes related to stomatal movement and ABA signaling was significantly upregulated in the s1,2 vs. WT comparison. These genes included RAB28, HVA22B, HVA22C and AREB3 (Supplementary Table S6B−D). However, the content of ABA was lower in s1,2 plants than in WT plants under dehydration (Fig. 4H), perhaps because of the increased expression of the ABA-catabolism-related gene CYP707A3 (Umezawa et al. 2006) (Supplementary Fig. S3 and Table S6C). These results implied a feedback mechanism between ABA levels and ABA responsiveness, which was modulated by SMAX1 and SMXL2 during dehydration stress. Crosstalk between the KAR-signaling and ABA-signaling pathways was also suggested by our previous investigation of kai2 plants, which exhibited lower expression of CYP707A3 and higher ABA levels than WT plants under dehydration conditions (Li et al. 2017, 2020c). It is worth mentioning that seedlings of SL-deficient L. japonicus and tomato (Solanum lycopersicum) mutants showed a decreased accumulation of ABA than WT plants under drought (Liu et al. 2015, Visentin et al. 2016, Chi et al. 2021), which suggest that the ABA metabolism may be modulated in different ways by SL-signaling and/or KAR-signaling pathways in different plant species. In total, the results of this study suggest that SMAX1 and SMXL2 negatively regulate stomatal closure and ABA responsiveness, thereby promoting leaf water loss through stomatal closure and other ABA-dependent pathways during drought resistance (Fig. 6F).
With regard to the role of ABA signaling in drought resistance, a recent report showed that the sucrose transporters SWEET11 and SWEET12 were phosphorylated and activated by ABA-signaling components’ SUCROSE NONFERMENTING 1-RELATED PROTEIN KINASE 2 (SnRK2) proteins in Arabidopsis (Chen et al. 2022). The activated SWEET11 and SWEET12 would enhance the transportation of sucrose from shoots to roots, which drove an increase in the root-to-shoot ratio and drought resistance (Yu et al. 2015, Durand et al. 2016, Chen et al. 2022). This result was consistent with the negative role of SMAX1 and SMXL2 in ABA responsiveness (Fig. 4A–E) and the higher root-to-shoot ratio in the s1,2 mutant reported here (Fig. 6B). The increase in the root-to-shoot ratio indicated that s1,2 plants may have a greater capacity for water uptake than WT plants (Fig. 6B), and this may also contribute to their enhanced drought resistance (Iwata et al. 2013, Uga et al. 2013, Durand et al. 2016, Du et al. 2020) (Fig. 1A, D).
We also found longer root hairs and a higher root hair density in the s1,2 mutant than in the WT (Fig. 6C–E), which is consistent with results of previous reports (Villaecija-Aguilar et al. 2019, 2022, Carbonnel et al. 2020). Their enhanced root hair development suggested that the s1,2 mutants had a greater capacity for water and nutrient uptake than the WT plants (Comas et al. 2013). Likewise, enhanced root hair development (Fig. 6C–E) may be related to the increased ABA sensitivity of the s1,2 mutant plants (Fig. 4), as ABA signaling positively regulates root hair development in Arabidopsis (Lombardo and Lamattina 2018, Li et al. 2020a). Several recent reports indicate that auxin signaling in Arabidopsis (Villaecija-Aguilar et al. 2022) and ethylene biosynthesis in L. japonicus (Carbonnel et al. 2020) are involved in root hair development, which is regulated by SMAX1 and/or SMXL2 in these species. These results suggest the involvement of SMAX1 and/or SMXL2 in the crosstalk of ABA, auxin and ethylene in the regulation of root hair development, which may then affect drought responses in plants.
Our previous results showed that KAI2 and D14 enhance drought resistance by promoting anthocyanin biosynthesis (Li et al. 2017, 2020b). Nonetheless, we did not find any difference in anthocyanin accumulation between s1,2 and WT plants under drought stress (Supplementary Fig. S5). One possible interpretation of this result is that s1,2 mutants are less stressed by water deprivation, and thus it was difficult to see the differential anthocyanin accumulation caused by both drought and loss-of-function of SMAX1 and SMXL2 in s1,2 and WT plants. This hypothesis as well as the potential involvement of SMAX1 and SMXL2 in the regulation of the endogenous anthocyanin content are supported by the slightly higher expression of anthocyanin-biosynthesis-related genes in the ‘s1,2-C vs. WT-C’ comparison but not in the ‘s1,2-D4 vs. WT-D4’ comparison (Supplementary Table S6E). In addition, the enhanced drought resistance of the s1,2 mutant may also be attributed to its higher ROS-scavenging capacity (Fig. 5). Plants contain multiple antioxidant enzymes for ROS scavenging, including CATs, GPXs, manganese superoxide dismutases (MSDs) and ascorbate peroxidases (APXs) (Noctor and Foyer 1998, Hasanuzzaman et al. 2020). Here, we found higher relative expression levels of APX3, APX4, CAT1, GPX3, GPX7 and MSD1 in the s1,2 mutant than in WT plants under both normal and dehydration conditions (Supplementary Table S6C).
Taken together, our results indicate that SMAX1 and SMXL2 negatively regulate drought resistance by inhibiting cuticle formation and wax biosynthesis, stomatal closure, ABA responsiveness, ROS-scavenging capacity, root-to-shoot ratio and root hair development (Fig. 6F). In addition, transcriptome analysis reveals that SMAX1 and SMXL2 regulate the genes associated with photosynthesis, suggesting that their effects on primary metabolism may also influence drought resistance. Additional studies of the regulation of drought resistance by SMAX1 and SMXL2 can promote improvements in plant drought tolerance to increase crop productivity in arid lands.
Materials and Methods
Plant materials and growth conditions
The Columbia ecotype (Col-0) of Arabidopsis was used as the WT in all experiments. Seeds of the s1,2 mutant were originally generated by Stanga et al. (2016). Seeds of WT and the s1,2 mutant were sown on an agar germination medium (GM) and incubated at 4°C in the dark for 3 d. Then, the plates with seeds were transferred to a growth chamber (16 h light/8 h dark cycle, 80 µmol m−2 s−1 photon flux density, 22°C) for 14 d to produce 14-day-old plate-grown seedlings. The 14-day-old seedlings were transferred to soil for another week to obtain 21-day-old seedlings for further experiments and treatments.
Drought resistance
Leaf surface temperature, dehydration treatments and transcriptome analysis
To measure leaf surface temperatures, a thermal camera system (FLIR-530; FLIR Optoelectronic Technology, Shanghai Co., Ltd, USA) was used to take pictures of rosette leaves on 14-day-old seedlings. WT and s1,2 mutant seedlings were grown on soil from direct-sown seeds. RWCs of rosette leaves from the s1,2 mutant and WT plants were measured after dehydration. In brief, rosette leaves were cut from 24-day-old (14 d on agar and 10 d in soil) the s1,2 mutant and WT plants and then placed on plastic trays for drying under laboratory conditions. FWs of the leaf samples were measured at different time points after the initiation of dehydration (0, 0.5, 1, 2, 3, 4, 5, 6, 7 and 8 h) as described previously (Li et al. 2020b). RWCs of the leaf samples were calculated using the following equation:
For transcriptome analysis, rosette leaves from 24-day-old seedlings were subjected to a dehydration treatment under the same environmental conditions described above for RWC measurements, and leaves were harvested after 0, 2 and 4 h of dehydration. Total RNA was extracted using the TRIzol Reagent kit (Thermo Fisher Scientific, USA). RNA samples from four biological replicates of each treatment and genotype were processed using the Arabidopsis Oligo 44K DNA microarray (version 4.0; Agilent, USA). Details of data acquisition and processing have been described previously (Ha et al. 2014), and more information on the microarray data is available at the Gene Expression Omnibus (https://www.ncbi.nlm.nih.gov/geo/) under the accession number GSE196838.
TB staining and chlorophyll leaching assays and observations of leaf surface waxes and stomatal aperture
TB staining and chlorophyll leaching assays were performed with the 24-day-old (14 d on agar and 10 d in soil) the s1,2 mutant and WT plants as described in detail in previous studies (Tanaka et al. 2004, Li et al. 2020b). Epicuticular wax was observed by SEM (Quanta 250, FEI, USA) as described previously (Li et al. 2020b).
Cotyledon opening, growth inhibition and stomatal closure assays
Stomatal closure assays were performed as described previously (Lv et al. 2018). In brief, the fourth and fifth leaves were detached from 28-day-old WT and s1,2 mutant plants, and the abaxial epidermis was peeled off. The epidermal strips were first preincubated in 2-(N-morpholino)ethanesulfonic acid-KCl buffer (10 mM MES, 50 mM KCl, 0.1 mM CaCl2, pH 6.15) for 2 h under strong light (150 μmol m−2 s−1). The strips were then transferred to fresh MES-KCl buffer with 0, 20 or 50 μM ABA and incubated for another 2 h. Stomatal densities and aperture sizes were calculated after photographing the strips under a light microscope.
ROS detection and plant ROS resistance responses
ROS levels and plant ROS resistance responses were measured as described previously (Li et al. 2020c).
Measurement of endogenous ABA and chlorophyll levels
Rosette leaves were harvested from 24-day-old soil-grown seedlings for dehydration treatment for 0, 2 or 4 h, which were the same as described above for RWC measurements. Rosette leaves exposed to dehydration for 0, 2 and 4 h were used for the determination of endogenous ABA contents as previously described (Kanno et al. 2016). The chlorophyll concentrations of the collected solutions and leaves were determined by measuring their absorbance at 645 nm (A645) and 663 nm (A663). The chlorophyll concentration of each solution was then calculated as:
qRT-PCR
The PrimeScript II 1st strand cDNA Synthesis Kit (TaKaRa, Kusatsu, Shiga, Japan) was used for reverse transcription and cDNA synthesis from the same RNA samples used for microarray analysis. The qRT-PCR procedure was performed following a previously reported method (Le et al. 2011) with UBQ10 as the reference gene and three biological replicates. All primers used for qRT-PCR analyses are listed in Supplementary Table S7.
Statistical analysis
The data are processed and shown as means ± SEs from three or more independent experiments. To compare the difference between the s1,2 mutant and the WT, Student’s t-test was performed. Asterisks show significant differences between the s1,2 mutant and the WT (*P < 0.05, **P < 0.01 and ***P < 0.001). To compare the different among genotypes and treatments, an analysis of variance followed by Duncan’s multiple range test (P < 0.05) was performed. Different letters above the error bars indicate statistically significant differences by using SPSS statistical software for calculation (IBM, USA).
Supplementary Data
Supplementary data are available at PCP online.
Data Availability
The data of this paper will be shared upon reasonable request to the corresponding author. The original microarray analysis results are available under the accession number GSE196838. Sequence data of this paper are available in the Arabidopsis Genome Initiative database as the following accession numbers: SMAX1 (AT5G57710) and SMXL2 (AT4G30350).
Funding
Strategic Priority Research Program of the Chinese Academy of Sciences (XDA28110100); National Key R&D Program of China (2018YFE0194000, 2018YFD0100304, 2016YFD0101006) from the Ministry of Science and Technology of the People’s Republic of China; National Natural Science Foundation of China (31770300, 31601821, 32170271); Key Scientific Research Projects of Institutions of Higher Education in Henan Province (22A180012); Cabinet Office, Government of Japan, Moonshot Research and Development Program for Agriculture, Forestry and Fisheries (Bio-oriented Technology Research Advancement Institution, No. JPJ009237).
Acknowledgements
We thank David C. Nelson from the University of California (USA) for kindly providing seeds of smax1, smxl2 and smax1 smxl2 mutants.
Authors Contributions
W.L. and L.-S.P.T. designed and planned the research. W.L., Z.F., X.L., H.T. and Y.W. performed the experiments and analyzed the data. K.H.N., C.D.T., M.A., K.X., M.G.M., C.V.H., K.M., C.T., M.T. and M.S. helped with designing, executing and interpreting the experiments. W.L., C.T., Y.M., Z.L. and L.-S.P.T. wrote and edited the manuscript. W.L. and L.-S.P.T. revised the manuscript.
Disclosures
The authors have no conflicts of interest to declare.
References
Author notes
contributed equally to this work.