-
PDF
- Split View
-
Views
-
Cite
Cite
Junjie Tan, Mingna Jin, Jiachang Wang, Fuqing Wu, Peike Sheng, Zhijun Cheng, Jiulin Wang, Xiaoming Zheng, Liping Chen, Min Wang, Shanshan Zhu, Xiuping Guo, Xin Zhang, Xuanming Liu, Chunming Wang, Haiyang Wang, Chuanyin Wu, Jianmin Wan, OsCOL10 , a CONSTANS-Like Gene, Functions as a Flowering Time Repressor Downstream of Ghd7 in Rice , Plant and Cell Physiology, Volume 57, Issue 4, April 2016, Pages 798–812, https://doi.org/10.1093/pcp/pcw025
- Share Icon Share
Abstract
Flowering time, or heading date, is a critical agronomic trait that determines the cropping season and regional adaptability, and ultimately grain yield in rice. A number of genes involved in photoperiodic flowering have been cloned and their roles in modulating expression of the flowering genes have been characterized to a certain extent. However, much less is known about the pathway in transmitting the day length response signal(s) to induce transition to reproductive growth. Here, we report a constitutive flowering repressor OsCOL10 , which encodes a member of the CONSTANS-like (COL) family. Transgenic rice plants overexpressing OsCOL10 (driven by a strong promoter or by fusing it to the activation domain of VP64) showed delayed flowering time under both short and long days. OsCOL10 is affected by the circadian clock and is preferentially expressed in leaf mesophyll cells; it is localized to the nucleus and has transcriptional activation activity. Further studies show that OsCOL10 represses the expression of the FT -like genes RFT1 and Hd3a through Ehd1. Transcripts of OsCOL10 are more abundant in plants carrying a functional Ghd7 allele or overexpressing Ghd7 than in Ghd7 -deficient plants, thus placing OsCOL10 downstream of Ghd7. Taking these findings together, we conclude that OsCOL10 functions as a flowering time repressor that links Ghd7 and Ehd1 in rice.
Introduction
Flowering is a complex transition from the vegetative phase to the reproductive phase in plants, and is triggered by both endogenous and environmental signals, such as light and temperature ( Song et al. 2013 ). Among environmental signals, the photoperiod or day length is one of the most important regulators of flowering, and is perceived by plant leaves ( Thomas 1997 , Hayama et al. 2003 , Song et al. 2013 ). Based on their response to photoperiod, plants generally fall into three classes: long-day (LD) and short-day (SD) plants, whose flowering times are promoted under LDs and SDs, respectively, and day-neutral plants, which are insensitive to day length. Flowering time (also called heading date) in crops is a key agronomic trait that determines the cropping season and regional adaptability. Control of flowering time has been extensively studied for >100 years ( Jung and Muller 2009 ).
Numerous genetic and molecular analyses of photoperiodically controlled flowering which have been undertaken in the model LD plant Arabidopsis have established the GIGANTEA ( GI )– CONSTANS ( CO )– FLOWERING LOCUS ( FT ) pathway, where GI integrates cellular signals from light sensory transduction and the circadian clock, and then activates CO , a plant-specific CCT (CO, CO-like and TOC1) domain transcription activator ( Putterill et al. 1995 , Fowler et al. 1999 , Park et al. 1999 , Yanovsky and Kay 2002 ). CO promotes flowering through direct activation of FT , which encodes a small mobile protein synthesized in the phloem of leaves. This protein is then transported to the shoot apical meristem where it induces expression of floral meristem identity genes (such as AP1 and LEAFY ) to initiate transition to reproductive growth ( Weigel et al. 1992 , Putterill et al. 1995 , Kardailsky et al. 1999 , Kobayashi et al. 1999 , Samach et al. 2000 , Abe et al. 2005 , Wigge 2006, Corbesier et al. 2007 , Turck et al. 2008 ).
The GI–CO–FT regulatory pathway is conserved in rice, an SD plant ( Turck et al. 2008 ). Under SDs, OsGI regulates expression of Heading date 1 ( Hd1 ), the ortholog of CO ( Yano et al. 2000 ). Hd1 positively regulates transcription of Heading date 3a ( Hd3a ), an ortholog of FT , and promotes flowering under SDs ( Kojima et al. 2002 , Hayama et al. 2003 ). Expression of Hd3a is also induced by another flowering activator known as Ehd1 ( Early heading date 1 ), a B-type response regulator that functions independently of Hd1 under SD conditions ( Doi et al. 2004 ). Under LDs, Hd1 converts into a flowering suppressor by reducing expression of Hd3a ( Hayama et al. 2003 ). LD suppression of flowering in rice is also mediated by the strong repressor Ghd7 ( Grain number, plant height and heading date 7 ), which encodes a small protein with a CCT motif ( Xue et al. 2008 ). Ghd7 is up-regulated under LDs and represses expression of Ehd1 and thus the downstream gene Hd3a ( Xue et al. 2008 ). In addition, expression of Ghd7 is activated by morning red light under LDs and at midnight under SDs. Loss-of-function of SE5 ( Photoperiod sensitivity 5 ), encoding a heme oxygenase involved in phytochrome chromophore biosynthesis, severely abolishes the expression of Ghd7 ( Itoh et al. 2010 ). Ehd1 and Ghd7 have no orthologs in the Arabidopsis genome, suggesting that Ghd7–Ehd1–Hd3a evolved as a rice-specific flowering suppression pathway ( Tsuji et al. 2011 ). However, the mechanism of Ghd7 suppression remains elusive.
Rice is a facultative SD plant and can eventually flower under non-inductive LD conditions. Recently, molecular genetic studies revealed that an ‘LD activation pathway’ exists to regulate flowering, where a key floral activator, RFT1 ( RICE FLOWERING LOCUS T1 ), the closest paralog of Hd3a , plays a central role ( Chardon and Damerval 2005 , Komiya et al. 2008 ). It was shown that RFT1 acts as a major LD activator, in contrast to Hd3a, which functions as a major SD activator ( Komiya et al. 2008 ). The expression of RFT1 is induced by Ehd1 and OsMAS50 , a homolog of SOC1 ( SUPPRESSOR OF OVEREXPRESION OF CONSTANS 1 ) ( Lee et al. 2004 , Komiya et al. 2008 , Komiya et al. 2009 ) . Thus, the OsMADS50–Ehd1–RFT1 pathway is involved in floral activation under LD conditions.
Accumulating data indicate that Ehd1 is a critical integrator of multiple flowering signals and is regulated by various factors, including multiple positive regulators, such as OsMADS51 under SDs, OsMADS50 and DTH2 ( Days to heading 2 ) under LDs, Ehd2 , Ehd3 , Ehd4 ( Early heading date2 , 3 , 4 ) under both SDs and LDs, negative regulators, such as Ghd7 , DTH8 , DTH7/OsPRR37/Ghd7.1 and OsCO3 , under either SDs or LDs, and OsphyB and OsCOL4 in a day length-independent manner ( Izawa et al. 2002 , Lee et al. 2004 , Kim et al. 2007 , Kim et al. 2008 , Matsubara et al. 2008 , Xue et al. 2008 , Komiya et al. 2009 , Lee et al. 2010 , Wei et al. 2010 , Matsubara et al. 2011 , Gao et al. 2013 , Koo et al. 2013 , Wu et al. 2013 , Yan et al. 2013 , Gao et al. 2014 ).
Previous studies have showed that the CONSTANS-like ( COL ) gene family plays critical roles in regulating flowering time in Arabidopsis and other diverse plant species. For example, Arabidopsis COL3 acts as a flowering repressor under SDs and LDs, and additionally as a positive regulator of red light signaling and root growth ( Datta et al. 2006 ). AtCOL9 attenuates flowering under LDs and AtCOL5 works as a flowering activator under SDs ( Cheng and Wang 2005 , Hassidim et al. 2009 ). HvCO9 regulates photoperiodic flowering in barley under SDs ( Kikuchi et al. 2012 ). The rice genome contains >16 COL members ( Robson et al. 2001 , Griffiths et al. 2003 ). Although some of them were shown to be regulators of photoperiodic flowering, including Hd1 , OsCO3 , OsCOL4 , Ghd7 and DTH2 ( Yano et al. 2000 , Kim et al. 2008 , Xue et al. 2008 , Lee et al. 2010 , Wu et al. 2013 ), the remainder are not characterized. In this study, we show that transgenic rice plants overexpressing OsCOL10 or the OsCOL10–VP64 fusion display delayed flowering under both SDs and LDs. We also show that OsCOL10 down-regulates expression of the florigen genes Hd3a and RFT1 through the flowering integrator Ehd1. Moreover, we demonstrated that expression of OsCOL10 is positively regulated by Ghd7 , a key LD-specific flowering suppressor. Our findings identify OsCOL10 as a constitutive and Ghd7 -regulated flowering repressor in rice.
Results
OsCOL10–VP64 overexpression causes a delayed flowering phenotype under SDs and LDs
VP64 is a strong transcriptional activator that has been used to study functionally redundant plant transcription factors (TFs) ( Beerli et al. 1998 , Parcy et al. 2002 , Silveira et al. 2007 ). To gain insight into the regulation mechanism underlying photoperiodic flowering in rice, we carried out a large-scale gain-of-function screening for flowering time-associated TFs by fusing various rice TFs to the VP64 activation domain (TFs–VP64) under control of the maize Ubiquitin ( Ubi ) promoter. This allowed us to overexpress the resulting constructs in a photoperiod-insensitive japonica rice cultivar (cv.) Kita-ake ( Fig. 1 A). Such fusions (TFs–VP64) in this system were previously shown to cause strong dominant effects and, moreover, were useful in studies of redundantly acting plant TFs ( Beerli et al. 1998 , Parcy et al. 2002 , Zhang 2003 , Silveira et al. 2007 , Ohmori et al. 2009 , Hanano and Goto 2011 ). Through phenotypic characteristics and genomic PCR analysis, we isolated >50 TFs that changed flowering time upon activation. Among them, a CONSTANS-like protein, OsJ (Os03g50310) ( Robson et al. 2001 , Griffiths et al. 2003 ), delayed flowering time significantly after fusion with VP64. We renamed OsJ as OsCOL10 and further studied its role in controlling photoperiodic flowering.
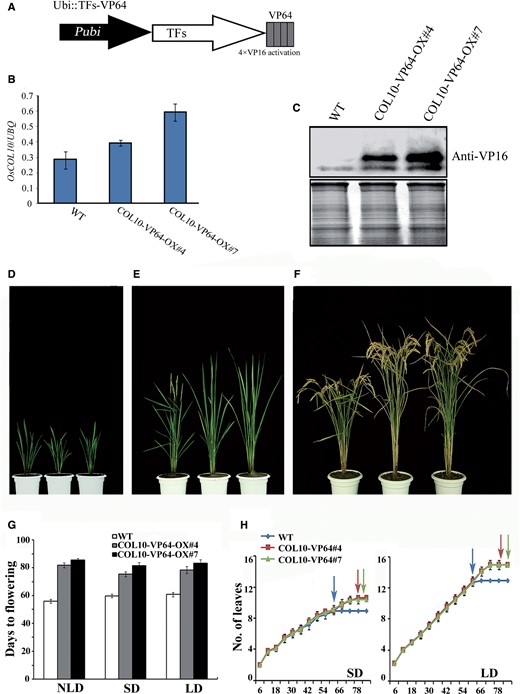
Overexpression of OsCOL10–VP64 showing delayed flowering time. (A) Schematic diagram of the P ubi::TFs-VP64 fusion construct. The tetrameric VP16 activation domain (gray boxes) made up the VP64 domain. P ubi , maize Ubiquitin promoter. (B) qRT-PCR analyses of OsCOL10 in the wild type (WT) and two transgenic lines. Samples were harvested at 4 h after dawn from second leaf blades from the top of 55-day-old plants under NLD conditions. (C) Western blot analyses of OsCOL10 in WT and transgenic lines with a VP16-specific antibody. The same filter was stained with Ponceau S to visualize total protein (bottom). (D–F) Phenotypic characteristics of WT and transgenic lines at the young (D), flowering (E) and mature stages (F); the plants from left to right in every panel are the WT and two independent transgenic lines (#4 and #7). (G) Comparison of flowering times among WT and two transgenic lines under different day length conditions. Days to flowering were scored when the first panicle emerged. Error bars indicate the standard deviation (s.d.); n = 10–20 plants. (H) Leaf emergence rate of WT and transgenic plants under both SDs and LDs ( n = 20). The arrow indicates the flowering times of corresponding lines.
We selected two independent homozygous transgenic lines (#4 and #7) showing increased accumulation of both mRNA and protein levels of OsCOL10 from a T 2 population ( Fig. 1 B, C). We next investigated growth of the two lines under natural long day (NLD) conditions. During the vegetative stage, growth of the transgenic plants was visually indistinguishable from that of wild-type plants ( Fig. 1 D). When transition from the vegetative to productive phase took place with emergence of young panicles in the wild type, the transgenic plants remained vegetative and continued to generate new leaves ( Fig. 1 E). The longer vegetative growth phase allowed the transgenic lines to grow taller and to produce larger panicles and more seeds than wild-type plants ( Fig. 1 F; Supplementary Fig. S1 ). We quantitatively compared several agronomic traits, including tiller number, plant height, panicle length, grain number per panicle, 1,000-grain weight, yield per plant and plot yield, between wild-type and transgenic plants under NLD conditions ( Supplementary Table S1 ). Among these traits, plant height, panicle length, grain number per panicle and grain yield (per plant and plot) in both transgenic lines were significantly higher than in wild-type plants.
Under NLD conditions, the transgenic lines flowered about 4 weeks (#4, 81.6 ± 1.8 d; #7, 85.5 ± 1.2 d) later than the wild-type plants (56.1 ± 1.5 d). To test whether the transgenic lines have different responses to day length, we grew the transgenic lines and wild type under SD (10 h light/14 h darkness) and LD (14 h light/10 h darkness) conditions. The transgenic plants flowered about 2 weeks later than wild-type plants under both light regimes ( Fig. 1 G). To determine whether the delayed flowering phenotype of transgenic plants was associated with reductions in growth rate, we compared the leaf emergence rates of the transgenic lines and wild type. As shown in Fig. 1 H, wild-type plants flowered after emergence of the ninth leaf under SDs and the 13th leaf under LDs. The transgenic plants had a similar leaf emergence rate until the wild-type plants flowered, indicating that the late flowering phenotype of transgenic plants was due to delayed floral induction rather than retarded growth rate. Thus, the enhanced production of OsCOL10–VP64 caused delayed flowering under NLDs, SDs and LDs. We conclude that OsCOL10–VP64 is a constitutive flowering suppressor.
To confirm further the role of OsCOL10 in photoperiodic control of flowering time, we created another overexpression construct (P ubi::OsCOL10 ) by placing the full-length coding sequence under control of the maize Ubi promoter ( Fig. 2 A). Three independent T 2 lines (OE4, OE9 and OE14) with increased OsCOL10 expression showed delayed flowering phenotypes under NLD conditions ( Fig. 2 B–D). Under SD conditions (10 h light/14 h darkness), we observed that as the wild-type plants were maturing, the OsCOL10 -overexpressing plants were still at the stem elongation stage ( Fig. 2 E). These results further support the notion that OsCOL10 contributes to flowering repression.
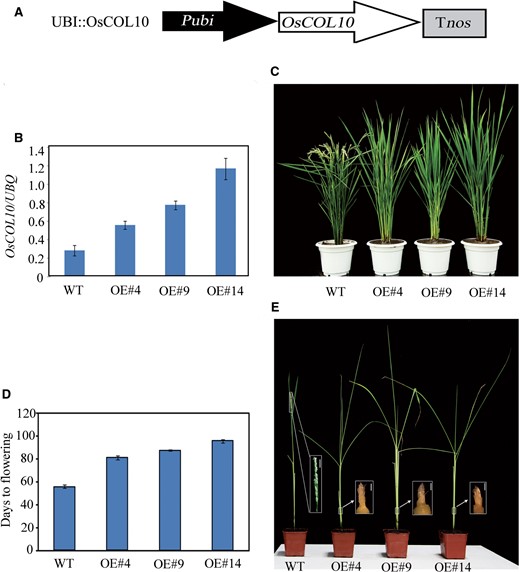
Phenotypic characterization of OsCOL10 -overexpressing plants. (A) Schematic diagram of the P ubi::OsCOL10 construct. Tnos , terminator of the nopaline synthase gene ( nos ). (B) qRT-PCR analyses of OsCOL10 in the wild type (WT) and three independent P ubi::OsCOL10 transgenic lines (OE#4, OE#9 and OE#14). Samples were harvested 4 h after dawn from second leaf blades from the tops of 55-day-old plants grown under NLD conditions. (C) Phenotypes of P ubi::OsCOL10 plants at flowering stages. (D) Comparison of flowering times among the WT and transgenic lines under NLD conditions. (E) Phenotypic characteristics of the WT and transgenic lines under SDs when the WT plants showed complete emergence of panicles from the apices. The white boxes indicate the sources of the corresponding magnified images. The first bar is 2 mm and the others are 1 µm.
To investigate the effect of down-regulation of OsCOL10 on flowering time, RNA interference (RNAi)-mediated OsCOL10 knockdown plants were produced. Three independent transgenic lines (RNAi-1, RNAi-2 and RNAi-3) with decreased OsCOL10 expression showed similar flowering times to the wild type ( Supplementary Fig. S2 ). To exclude the possibility that reduced expression in RNAi lines is also sufficient for plant growth, we generated oscol10 mutants by the CRISPR (clustered, regularly interspaced, short palindromic repeats)–Cas method ( Miao et al. 2013 ) and obtained homozygous oscol10 mutant lines, oscol10-1 , oscol10-2 and oscol10-3. In the oscol10-1 mutant, a 1 bp insertion was present at a position 410 bp from the ATG start codon, resulting in a frameshift ( Supplementary Fig. S3A ). We then compared flowering times among the wild type and the mutant lines, and no significant differences were found ( Supplementary Fig. S3B, C ), suggesting that OsCOL10 shared functional redundancy with other gene(s) in floral regulation.
OsCOl10 is a circadian clock-regulated gene and mainly expressed in the leaves
CO is a member of the COL gene family, which is characterized by a CCT domain near the C-terminus ( Putterill et al. 1995 , Griffiths et al. 2003 ). In Arabidopsis, COL proteins can be classified into three groups based on the structure of the B-box near the N-terminus. Group I members (CO and AtCOL1–AtCOL5) possess two B-boxes; group II (AtCOL9–AtCOL15), one B-box and a zinc-finger domain; and group III (AtCOL6–AtCOL8 and AtCOL16), one B-box ( Griffiths et al. 2003 ). In rice, there are at least 16 members of the COL family that similarly fall into the three main phylogenetic clades ( Griffiths et al. 2003 , Huang et al. 2012 ; Supplementary Fig. S4 ). OsCOL10 belongs to group II, and none of them has been functionally characterized ( Supplementary Fig. S4 ). It is predicted that OsCOL10 contains a B-box domain at the N-terminus and a CCT domain at the C-terminus ( Finn et al. 2014 ). A BLAST search with the OsCOL10 protein sequence revealed close homologs in Setaria italica , Sorghum bicolor , Zea mays , Hordeum vulgare and Brachypodium distachyon ( Supplementary Fig. S5 ). Unfortunately, the functions of these homologs were largely unknown.
We examined the temporal expression pattern of OsCOL10 using the samples collected from rice plants grown under SD and LD conditions. The expression of OsCOL10 showed a circadian oscillation ( Fig. 3 A). Under LDs, the abundance of OsCOL10 mRNA was low at night, and increased at dawn, with a peak in expression after 4 h. Under SDs, the abundance of OsCOL10 was low at night and increased at 4 h before dawn, with a peak of expression at dawn ( Fig. 3 A). The rhythmic amplitude of COL10 expression was higher in plants grown under SDs than in those grown under LDs ( Fig. 3 A). Moreover, OsCOL10 started to accumulate during vegetative growth from the fourth week after germination and reached a peak at the sixth week under SDs, whereas the accumulation under LDs was delayed to the sixth week and peaked at the eighth week ( Fig. 3 B).
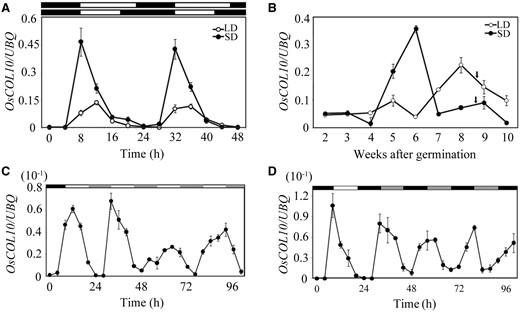
OsCOL10 is a circadian clock-regulated gene. (A, B) Rhythmic and developmental expression of OsCOL10. In (A), the penultimate leaf blades were harvested at the indicated time points from 30-day-old (SDs) and 35-day-old (LDs) plants. In (B), the penultimate leaf blades were harvested at dawn of the indicated day. Arrowheads represent the flowering time of plants at corresponding growth stages. (C, D) qRT-PCR analysis of diurnal expression patterns of OsCOL10 under continuous light (LL) (C) and continuous darkness (DD) (D) conditions. The plants were first grown in 12 h light/12 h darkness for 30 d and then transferred to LL or DD at dusk. In (A), (C) and (D), white bars indicate light; black bars indicate darkness. The gray bars represent the subjective darkness in (C) and subjective light in (D). Values represent means ± standard deviation (s.d.) from three independent biological replicates. The rice Ubiquitin ( UBQ ) gene was used as the internal control.
To determine further whether OsCOL10 expression is regulated by the circadian clock, wild-type plants were first grown in cycles of 12 h light/12 h darkness for 30 d and then transferred to continuous light (LL) or continuous darkness (DD). Remarkably, the rhythmic amplitude of OsCOL10 expression was drastically reduced, but continued oscillating for a time after the plants were moved into DD or LL ( Fig. 3 C, D). The results indicate that expression of OsCOL10 is under circadian clock control.
According to the data submitted to the microarray transcript profiling database RiceXPro ( http://ricexpro.dna.affrc.go.jp/ , Locus ID: Os03g0711100), OsCOL10 was mainly expressed in leaves. To verify these data, we analyzed the spatial expression pattern of OsCOL10 by quantitative real-time PCR (qRT-PCR) using RNA samples from various tissues and leaves at different developmental stages ( Fig. 4 A). OsCOL10 was expressed preferentially in the leaves, especially in young developing leaves ( Fig. 4 B). Histochemical staining of transgenic plants carrying the GUS (β-glucuronidase) reporter gene driven by the OsCOL10 promoter supported the above expression pattern and further delimited GUS activity to cells outside the vascular tissues ( Fig. 4 C–J). In situ hybridization assays confirmed that OsCOL10 was enriched in leaf mesophyll cells ( Fig. 4 K, L).
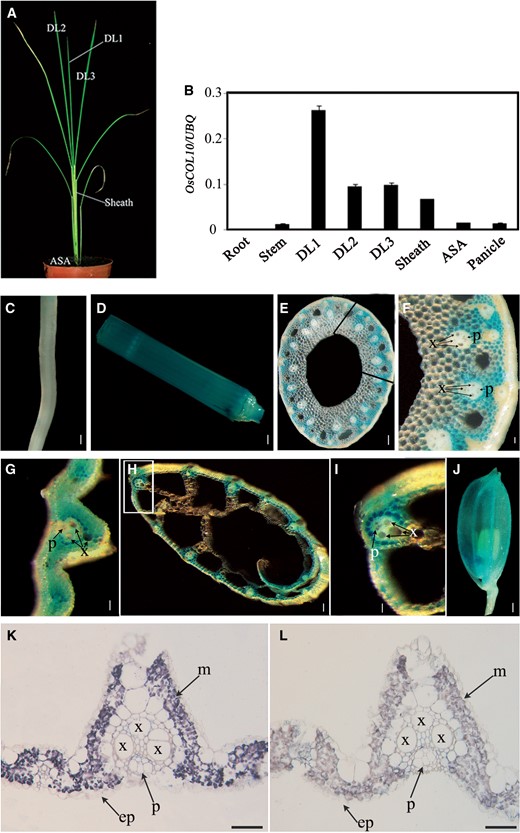
OsCOL10 was preferentially expressed in the leaves. (A) Thirty-day-old wild-type plants grown under SDs were used for qRT-PCR. DL1, newly emerging leaf; DL2, expanding leaf; DL3, fully expanded leaf; ASA, around the shoot apex. (B) OsCOL10 transcript levels in various organs (means ± standard deviation, n = 3). (C–J) Histochemical staining of various organs in p OsCOL10 :: GUS transgenic plants. (C) Root; (D) stem; (E) transverse sections of the stem; (F) magnified image of the boxed area in (E); (G and H) transverse sections of the leaf blade and sheath; (I) enlarged image of the boxed area in (H); (J) floret. (K–L) RNA in situ hybridization analysis of OsCOL10. The materials were harvested at 4 h after dawn under LD conditions. Leaf blades of 35-day-old wild-type plants were cross-sectioned and hybridized with OsCOL10 -specific antisense (K) or sense (L) probes. x, xylem; p, phloem; ep, epidermis; m, mesophyll.
OsCOl10 may act as a transcriptional regulator
The CCT domain of OsCOL10 suggests that it is likely to be localized in the nucleus ( Robson et al. 2001 , Zhang et al. 2015 ). To confirm that OsCOL10 is a nuclear protein, a translational fusion of OsCOL10 and green fluorescent protein (GFP) under control of the Cauliflower mosaic virus (CaMV) 35S promoter (35S:OsCOL10–GFP) was constructed. A transient expression assay in onion epidermis cells was used to assess the subcellular localization of the fusion protein. In cells bombarded with expression of the fusion protein, a signal of GFP fluorescence was localized in the nucleus; whereas in cells expressing free GFP, GFP fluorescence could be detected in both the nucleus and cytoplasm ( Fig. 5 A–F). Nuclear localization of OsCOL10 was further confirmed by observation of the OsCOL10–GFP fusion protein in stable transgenic rice root cells ( Fig. 5 G–I). These results confirm that OsCOL10 is localized in the nucleus.
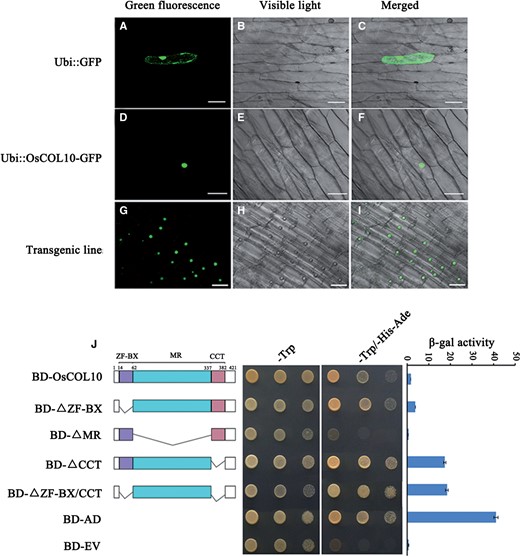
OsCOL10 acts as a transcriptional regulator. Subcellular localization of fused OsCOL10–GFP in onion epidermal cells (A–F) and transgenic rice roots cells (G–I). Scale bars = 50 µm. (J) Transactivation activity assays of OsCOL10 and its deletion derivatives in the yeast GAL4 system. BD, GAL4-DNA-binding domain; AD, GAL4 activation domain; ZF, MR and CCT indicate the zinc finger region, the middle region and the CCT domain of OsCOL10, respectively; EV denotes empty vector. β-Galactosidase (β-gal) activity was measured using a liquid culture assay. Mean values ± standard deviation (s.d.) were collected from three independent experiments.
We next investigated whether OsCOL10 has transcriptional activation activity. As shown in Fig. 5 J, full-length OsCOL10 had weak transcriptional activation activity in yeast cells. Deletion analysis showed that neither the B-box zinc finger domain nor the CCT domain alone could induce transcriptional activation activity; rather, the middle region between them was essential in a similar way to CO and other previously reported COL proteins ( Tiwari et al. 2010 , Wu et al. 2013 ). These results support the notion that OsCOL10 may have an important role in transcriptional regulation of downstream gene expression.
OsCOL10 delayed flowering time mainly through repression of Ehd1
In rice, Hd3a and RFT1 , known as florigen genes, are required for floral induction ( Kojima et al. 2002 , Tamaki et al. 2007 , Komiya et al. 2008 ). To understand the molecular mechanism by which OsCOL10 contributes to flowering repression, we determined the transcriptional levels of Hd3a and RFT1 in wild-type and transgenic plants by qRT-PCR. The expression levels of Hd3a and RFT1 were diminished in transgenic plants overexpressing modified OsCOL10 ( OsCOL10–VP64 ) and OsCOL10 under both SD and LD conditions ( Fig. 6 B, C, G, H; Supplementary Fig. S6B, C, G, H ). The transcript abundance of downstream floral meristem identity genes, such as OsMADS14 and OsMADS15 , was also reduced in the transgenic plants ( Supplementary Figs. S7A, B, K, L, S8A, B, K, L ).
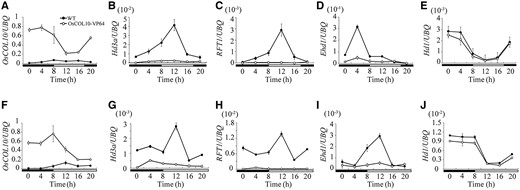
Rhythmic expression pattern of OsCOL10 , Hd3a , RFT1 , Ehd1 and Hd1 in the wild type and OsCOL10-VP64 transgenic plants under SDs (A–E) and LDs (F–J). Penultimate leaf blades were harvested at the indicated time point from 30-day-old (SDs) and 35-day-old (LDs) wild-type and transgenic plants. The open and filled bars at the bottom represent light and dark periods, respectively. Expression levels are relative to the rice UBQ gene. Values are shown as means ± standard deviation (s.d.) of three independent biological replicates.
Transduction of floral signals in rice mainly occurs through two key floral integrators, Hd1 and Ehd1 ( Tsuji et al. 2011 ). To understand whether OsCOL10 -regulated expression of the two florigen genes is mediated by Hd1 , Ehd1 , or both, we compared the mRNA levels of these two genes in wild-type and transgenic plants under SD and LD conditions. Interestingly, the abundance of Ehd1 was reduced in the transgenic plants, whereas the expression level of Hd1 was similar in transgenic and wild-type plants ( Fig. 6 D, E, I, J; Supplementary Fig. S6D, E, I, J ). In transgenic plants, where OsCOL10 (on its own or fused to VP64) is under control of the maize Ubi promoter, the expression of OsCOL10 maintains relatively high levels at each time point, which contributes to enhance the repression of Ehd1 ( Fig. 6 A, F, D, I; Supplementary Fig. S6A, F, D, I ). These results suggest that OsCOL10 regulates Hd3a and RFT1 expression through Ehd1. We also examined the expression of other known flowering regulators in transgenic and wild-type plants, and found that transcriptional levels were not affected in the transgenic plants ( Supplementary Figs. S7, S8 ). These findings suggest that OsCOL10 functions are not upstream of the known floral regulators.
To verify further that OsCOL10 suppresses Hd3a and RFT1 through Ehd1 , we used qRT-PCR to compare expression levels of the three genes in wild-type and transgenic plants during a 10-week growth period. Transcription levels of Ehd1 , Hd3a and RFT1 in transgenic plants were significantly reduced in comparison with those in wild-type plants from 2 weeks after germination ( Fig. 7 ), thus further supporting the notion that OsCOL10 down-regulates expression of Ehd1 and then the two downstream florigen genes ( Hd3a and RFT1 ).
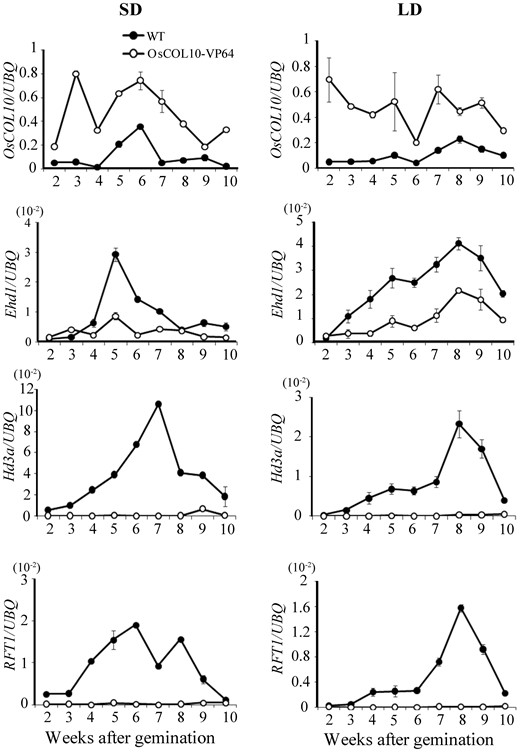
Changes in levels of OsCOL10 , Ehd1 , Hd3a and RFT1 transcripts during development in wild-type and OsCOL10-VP64 plants. Samples were collected from the penultimate leaf blades at dawn of the indicated day under SD and LD conditions. Expression levels are relative to that of the rice UBQ gene. Values are shown as means ± standard deviation (s.d.) of three biological replicates.
To understand further whether OsCOL10 regulated transcription of Ehd1 in a direct manner, a yeast one-hybrid assay was performed. The results showed that OsCOL10 could not directly bind to the promoter region of Ehd1 ( Supplementary Fig. S9 ).
Expression of OsCOL10 is regulated by Ghd7
To determine whether OsCOL10 is regulated by known flowering genes we examined the circadian expression of OsCOL10 in near isogenic lines (NILs) carrying defective Hd1 , Hd2 , Ehd1 , Hd3a , DTH2 , DTH7 , DTH8 or Ghd7 alleles, as well as in OsphyA , OsphyB and OsphyC mutants under SD and LD conditions. Expression of OsCOL10 was detected with no significant differences from wild-type counterparts in most of the mutants and NILs except for the NILs of Ghd7 ( Fig. 8 A; Supplementary Fig. S10 ). Additionally, there was no significant change of the OsCOL10 expression observed in NILs deficient in either Hd3a or Ehd1 ( Supplementary Fig. S10G, I ).
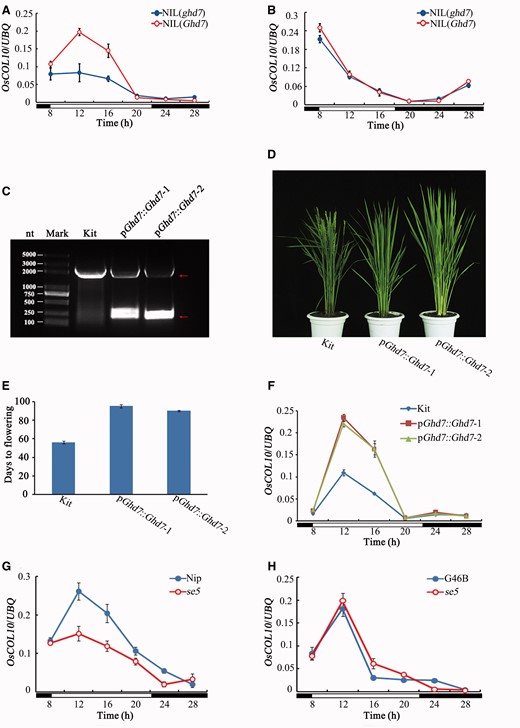
Expression of OsCOL10 is regulated by Ghd7. (A, B) Comparison of circadian expression of OsCOL10 between the near isogenic lines carrying functional ( Ghd7 ) and non-functional ( ghd7 ) alleles under LD (A) and SD (B) conditions. (C) The functional Ghd7 allele was introduced into the Ghd7 -deficient rice cv. Kita-ake, and transgenic positive plants were checked by PCR with the primers flanking the intron. Red rows represent the two amplified fragments in the transgenic positive plants. (D) Phenotypic characterization of transgenic positive plants under NLD conditions. (E) Comparison of flowering time between the Ghd7 transgenic and wild-type plants under NLD conditions. (F) Circadian expression of OsCOL10 in the wild-type plants and Ghd7 transgenic lines. (G, H) Diurnal expression of OsCOL10 in the wild type and se5 mutants with the background of Nipponbare (Nip) (G) or Gang46B (G46B) (H) under LD conditions. Penultimate leaf blades were harvested at the indicated time point from 30-day-old (SDs) (B) and 35-day-old (LDs) (A, F, G–H) transgenic plants or mutants and corresponding wild-type plants. Open and filled bars at the bottom in (A, B) and (F–H) represent the light and dark periods, respectively. Expression levels are relative to the rice UBQ gene. Values are means ± standard deviation (s.d.) of three independent biological replicates.
The abundance of OsCOL10 transcripts in the NIL carrying a defective allele of Ghd7 [NIL ( ghd7 )] was lower than that in the NIL carrying a functional allele of Ghd7 [NIL ( Ghd7 )] under LD conditions, suggesting that Ghd7 regulates its expression under LDs. Given that wild-type Kita-ake plants carry a defective Ghd7 allele ( Gao et al. 2013 ), we introduced the functional full-length Ghd7 coding sequence driven by its native promoter from japonica cv. Nipponbare into Kita-ake to create a Ghd7/ghd7 pair of a different genetic background. Transgenic Ghd7 and non-transgenic plants amplified distinctly different PCR fragments with primers flanking the Ghd7 intron ( Fig. 8 C). The transgenic plants exhibited a late-flowering phenotype relative to the wild type ( Fig. 8 D, E) under NLDs. We then compared the expression pattern of OsCOL10 between the transgenic and wild-type plants under LDs. As expected, the mRNA transcripts of OsCOL10 became more abundant as a result of the introduction of the functional Ghd7 allele ( Fig. 8 F). Since Ghd7 expression was severely impaired in the se5 mutant ( Itoh et al. 2010 ), we also investigated the circadian expression of OsCOL10 in se5 in the background of Nipponbare ( Sun et al. 2012 ). The abundance of OsCOL10 transcript was significantly reduced in se5 compared with that in the wild type ( Fig. 8 G). To determine whether such a reduction in OsCOL10 expression was mediated by Ghd7 , we used another se5 mutant in the background of Gang46B ( Chen et al. 2013 ), where Ghd7 activity is absent ( Supplementary Fig. S11 ). Differences in expression of OsCOL10 wild-type and se5 plants were not significant ( Fig. 8 H), suggesting that the reduced expression of OsCOL10 in this se5 mutant resulted from lack of Ghd7 expression, thus further supporting involvement of Ghd7 in regulation of OsCOL10. Ghd7 was reported to function as a TF ( Xue et al. 2008 ). A yeast one-hybrid assay was performed to determine whether transcription of OsCOL10 was directly regulated by Ghd7. The result indicated that Ghd7 could not bind directly to the promoter region of OsCOL10 ( Supplementary Fig. S12 ). We also measured the circadian expression of OsCO3 and OsCOL4 , two other COL family members acting as flowering repressors, as well as OsK and O sL , close to OsCOL10 in the phylogenetic tree of group II, in the Ghd7 NIL and transgenic plants, together with the corresponding controls. No significant differences were detected ( Supplementary Fig. S13 ). Thus the overall results suggest that Ghd7 regulates OsCOL10 specifically under LDs.
Discussion
OsCOL10 is a constitutive flowering repressor under SD and LD conditions
In this study we characterized a constitutive flowering repressor, OsCOL10 , that was previously identified as a member of the COL gene family ( Griffiths et al. 2003 , Huang et al. 2012 , Zhang et al. 2015 ). Although some members involved in photoperiodic control of flowering time had been reported in rice, OsCOL10 acts differently from most of these COL members because it represses flowering independently of day length. For example, Hd1 , the first identified member and an ortholog of CO , functions as a flowering promoter in SDs but as a repressor in LDs ( Yano et al. 2000 ); OsCO3 negatively regulates flowering under SD conditions ( Kim et al. 2008 ); Ghd7 is an LD-specific flowering repressor ( Xue et al. 2008 ); and Days to heading 2 ( DTH2 ), encoding a COL protein, works as an LD-specific flowering inducer ( Wu et al. 2013 ). OsCOL4 was known as the only COL member that repressed flowering under both SD and LD conditions ( Lee et al. 2010 ). Although OsCOL10 and OsCOL4 have many similarities in regulating flowering in rice, such as flowering repression by reducing the expression of Ehd1 , they are nevertheless involved in different photoperiodic regulatory pathways. OsCOL4 acts downstream of OsphyB , another constitutive flowering repressor, and thus the OsphyB–OsCOL4 regulatory pathway is shared in SDs and LDs ( Lee et al. 2010 ). In contrast, OsCOL10 is not regulated by phytochrome genes and has different regulatory mechanisms under SD and LD conditions ( Fig. 9 ; Supplementary Fig. S10 ). Expression of OsCOL10 peaked at dawn in SDs and 4 h after dawn in LDs, whereas Ehd1 expression peaked at 4 h before and after dawn in SD and LD conditions, respectively ( Figs. 3 A, 6D, I), suggesting that OsCOL10 may have a role in switching off Ehd1 expression in a circadian manner. OsCOL10 RNAi plants and CRISPR–Cas-induced oscol10 mutants showed no visible phenotypic differences, suggesting that, like other COL gene(s), OsCOL10 shared functional redundancy in floral regulation. Similar results were reported in COL studies in Arabidopsis. For example, overexpression of ATCOL5 or ATCOL9 promoted or repressed flowering, respectively, but their mutants showed no significant changes in phenotype ( Cheng and Wang 2005 , Hassidim et al. 2009 ). These findings demonstrated that COL family members act distinctly and redundantly to co-ordinate regulation of flowering. The mechanism by which this occurs needs to be further elucidated.
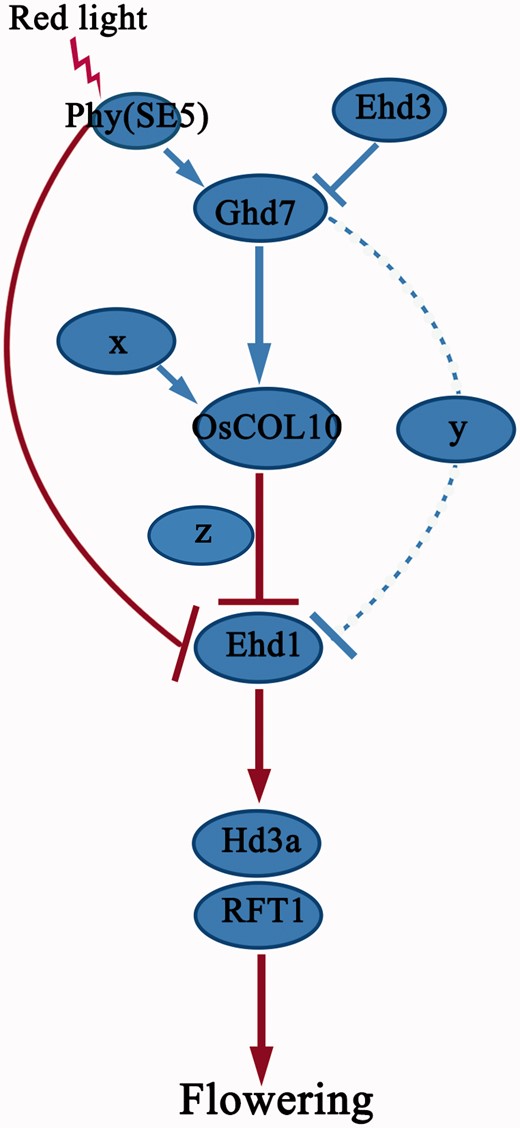
A proposed model of OsCOL10 in regulation of photoperiodic flowering time in rice. Blue lines represent pathways in LDs and red lines indicate pathways shared between SDs and LDs. x, y and z represent existing but unknown gene(s). Dashed lines indicate unknown regulatory genes that may function by activation or repression.
Plants overexpressing a modified OsCOL10 ( OsCOL10–VP64 ) or OsCOL10 itself displayed parallel delayed flowering under SD and LD conditions, indicating that OsCOL10–VP64 acts like the native OsCOL10, suggesting that the native OsCOL10 functions as a transcriptional activator or a carrier of an activator to promote the transcription of unknown downstream flowering repressors. It is noteworthy that OsCOL10 has only weak transcriptional activation activity in yeast cells ( Fig. 5 J). We propose that OsCOL10 might act as a mediator in regulatory complexes. In other words, OsCOL10 may interact with a co-activator to participate in transcriptional activation. However, we failed to isolate such candidate proteins by yeast two-hybrid screening, and their identification remains an important task for future studies. In addition, OsCOL10 represses Ehd1 probably in an indirect fashion ( Supplementary Fig. S9 ), suggesting the possibility that OsCOL10 activates an unknown factor which represses Ehd1 ( Fig. 9 ).
In OsCOL10–VP64 plants, expression levels of Hd3a and RFT1 under SDs and LDs were almost completely repressed until 10 weeks after germination ( Fig. 7 ). However, they flowered around 12 weeks after germination ( Fig. 1 ). A possible explanation is that the samples in Fig. 7 were taken at dawn while expression of Hd3a and RFT1 peaks at 4 h after dawn under SD and LD conditions ( Fig. 6 ), thus accumulation of expression at the peak point may be capable of inducing flowering.
OsCOL10 is regulated by Ghd7 under LDs
Flowering in rice is inhibited under LD conditions. One of the major contributors to LD-dependent flowering repression in rice is the presence of a small CCT domain protein, Ghd7, which not only acts as a key negative regulator of flowering, but also has crucial roles in promoting productivity and adaptability ( Xue et al. 2008 ). Rice cultivars with impaired function of Ghd7 ( Ghd7-0 or Ghd7-0 a ) with reduced sensitivity to photoperiod flower very early even in LD conditions ( Xue et al. 2008 ). It was shown that Ghd7 -associated repression of the flowering time was mainly through the suppression of the flowering promoter Ehd1 ( Xue et al. 2008 , Komiya et al. 2009 ). In addition, repression of Ehd1 by Ghd7 is crucial for rice to recognize the critical day length ( Itoh et al. 2010 ). Although some genes were reported to regulate the expression of Ghd7 , such as SE5 and Ehd3 ( Itoh et al. 2010 , Matsubara et al. 2011 ), the mechanism by which Ghd7 represses Ehd1 is still unknown. In the current study, we obtained evidence to show that expression of OsCOL10 is regulated by Ghd7 ( Fig. 8 ). Both Ghd7 and OsCOL10 act as repressors of Ehd1. Therefore, OsCOL10 establishes a functional link between Ghd7 and Ehd1 in the photoperiodic control of flowering. It is noteworthy that expression of OsCOL10 is still abundant and rhythmic when Ghd7 is deficient ( Fig. 8 A, F), indicating that Ghd7 is not the sole regulator of OsCOL10. Thus, we speculated that OsCOL10 is diurnally regulated by another unknown flowering regulator(s) under LDs ( Fig. 9 ). In addition, repression of Ehd1 by Ghd7 is not only mediated by OsCOL10 , because OsCOL10 RNAi and oscol10 plants showed no visible phenotypes ( Supplementary Figs. S2, S3 ) and the effect of OsCOL10 on flowering in rice is far less than that of Ghd7. These findings lead us to believe that there are at least two pathways through which Ghd7 represses the expression of Ehd1 ( Fig. 9 ). In other words, the final repression effect of Ehd1 through Ghd7 comes from the combined effects of these pathways. Identification of the other pathway(s) is still a challenge for future studies.
Ghd7 is induced by phytochromes, and its expression is severely reduced in se5 mutants ( Itoh et al. 2010 ). In addition, SE5 repressed the expression of Ehd1 under both SD and LD conditions ( Andres et al. 2009 ). SE5 suppression of Ehd1 can be mediated by Ghd7 ( Itoh et al. 2010 ), but we also showed that SE5 represses Ehd1 independently of Ghd7 in LDs ( Supplementary Fig. S11D ), because the expression of Ehd1 is still significantly increased in the se5 mutant with the background of G46B where Ghd7 is not functional. Therefore, there are two pathways through which SE5 suppresses the expression of Ehd1 under LDs: a Ghd7 - dependent pathway and a Ghd7 -independent pathway ( Fig. 9 ). Our results showed that OsCOL10 can be regulated by SE5 in a Ghd7 -mediated manner under LDs ( Fig. 8 G, H). Therefore, OsCOL10 is involved in the Ghd7 -dependent pathway, whereas the previously indentified COL gene, OsCOL4 , which functions between OsPHYB and Ehd1 , is implicated in the other pathway.
OsCOL10 was preferentially expressed in leaf mesophyll cells ( Fig. 4 K, L), whereas Ghd7 mainly accumulated in vascular tissues ( Xue et al. 2008 ). Moreover, yeast one-hybrid assays showed that Ghd7 does not directly regulate the expression of OsCOL10 ( Supplementary Fig. S12 ), implying that OsCOL10 is not the direct target of Ghd7, and that other unidentified proteins must transmit the signal to mediate the regulatory machinery.
Materials and Methods
Plant material and growth conditions
Overexpression lines for OsCOL10 fused or not fused to VP64 were generated in Oryza sativa japonica cv. Kita-ake. All plants were grown in a paddy field in Beijing (40°13′N, 116°13′E) or in controlled chambers under SDs (10 h light at 30°C/14 h darkness at 25°C) or LDs (14 h light at 30°C/10 h dark at 25°C) with a relative humidity of approximately 70% and light intensity of about 800 µmol m –2 s –1 .
For gene expression analyses, the following accessions and their NILs were used: Kita-ake carrying a non-functional DTH7 and a NIL carrying a functional DTH7 allele; Asominori carrying a functional DTH2 allele and a NIL carrying a non-functional DTH2 allele; Nipponbare carrying a functional Hd1 allele and a NIL carrying a non-functional Hd1 allele; Nipponbare carrying a functional Hd2 allele and a NIL carrying a non-functional Hd2 allele; Nipponbare carrying a partially functional Hd3a allele and a NIL carrying a functional Hd3a allele; Taichun 65 carrying a non-functional Ehd1 allele and a NIL carrying a functional Ehd1 allele; Asominori carrying a functional DTH8 allele and a NIL carrying a non-functional DTH8 allele; and a NIL pair carrying functional or non-functional Ghd7 alleles in the Zhenshan97 background.
Vector construction and transformation
To create the overexpression construct P ubi :: OsCOL10-VP64 , the OsCOL10 full-length cDNA driven by the maize Ubi gene was cloned into the binary vector CVP64B using the Gateway cloning system. For P ubi :: OsCOL10 , OsCOL10 full-length cDNA driven by the maize Ubi promoter was cloned into binary vector pCAMBIA1390 using In-Fusion Advantage PCR Cloning Kits (Clontech). To generate a p Ghd7 :: Ghd7 construct, the coding sequence of Ghd7 driven by its native promoter was cloned into pCAMBIA-1305.1. The resulting plasmids were transformed into Kita-ake plants by Agrobacterium tumefaciens -mediated transformation as described previously ( Hiei et al. 1994 ).
For RNAi, the construct pCUbi1390-ΔFAD2 ( Ubi promoter and a FAD2 intron inserted into pCAMBIA1390) was used as an RNAi vector ( Tan et al. 2014 ). Both antisense and sense versions of a specific 153 bp fragment from the coding region were amplified (primer pairs COL10RNAi-1 and COL10RNAi-2; Supplementary Table S2 ), and inserted into pCUbi1390-ΔFAD2 to form the RNAi construct pUbi-dsRNAi-COL10, which was then transformed into japonica cv. Nipponbare by the above method.
The CRISPR-Cas system was used as described previously ( Miao et al. 2013 ) to obtain oscol10 mutant lines. Briefly, an 18 bp OsCOL10 -specific spacer sequence was first cloned into entry vector pOs-sgRNA followed by ligation into a binary vector containing the Cas9 expression cassette using the Gateway cloning system. The resulting combination vector was then transformed into cv. Kita-ake as described above. All primer sequences are listed in Supplementary Table S2 .
RNA preparation and qRT-PCR analysis
Rice total RNA was extracted with an RNA Prep Pure Kit (Zymo Research) according to the manufacturer’s instructions. Then, 20 µl of cDNA was synthesized using 1 µg of RNA with the QuantiTect Reverse Transcription Kit (QIAGEN). Primer pairs ( Supplementary Table S2 ) were designed using Primer Express (Applied Biosystems). qRT-PCR was conducted using SYBR Premix Ex Taq Kit (TAKARA; RR041A) in an ABI PRISM 7900HT (Applied Biosystems) according to the manufacturer’s instructions. The procedure was: initial polymerase activation for 30 s at 95°C followed by 40 cycles of 95°C for 5 s and 60°C for 34 s. The rice Ubi gene was used as the internal control. For each sample, qRT-PCR was performed with three technical and three biological replicates. The 2 –ΔΔCT method was used to analyze relative transcript levels of gene expression ( Livak and Schmittgen 2001 ).
RNA in situ hybridization.
Five-week-old leaf blades were collected and fixed in FAA solution (50% ethanol, 5% acetic acid, 3.7% formaldehyde) overnight at 4°C, followed by a series of dehydration steps, and then embedded in paraffin (Paraplast Plus, Sigma), where RNA in situ hybridization was performed as described previously ( Bradley et al. 1993 ). To prepare the probe, we used a pair of primers, COL10-in situ-F (5′-AGAGGACGACGAGGACCT-3′) and COL10-in situ-R (5′-ATGACTCGCTGGGATCGAA-3′), to amplify a 503 bp unique sequence of OsCOL10 from a cDNA clone. The fragment was then inserted into the pGEM-T vector (Promega) for RNA transcription. Digoxigenin-labeled RNA probes were prepared using a DIG Northern Starter Kit (Cat. No. 2039672, Roche) following instructions provided by the manufacturer. The slides were observed under bright field with a Leica DM5000B microscope and photographed with a Leica DFC490 camera.
Subcellular localization of OsCOL10–GFP proteins
The coding sequence of OsCOL10 was amplified and cloned into the N-terminus of GFP under control of the CaMV 35 S promoter in the transient expression vector pA7-GFP, generating recombinant pA7-OsCOL10–GFP. The recombinant vector was then transformed into onion epidermal cells via shotgun bombardment (PDS-1000/He; Bio-Rad). The GFP signal was visualized using a confocal laser scanning microscope (LSM 700; Carl Zeiss).
Transactivation activity assays
Transactivation activity assays were performed using the Match-maker GAL4 Two-Hybrid System 3 (Clontech). Plasmids containing the GAL4 DNA-binding domain fused with OsCOL10 deletions were transformed into yeast strain AH109. The substrate chlorophenol red-β- d -galactopyranoside (CPRG; Roche Biochemicals) was used to measure β-galactosidase activity according to the Yeast Protocols Handbook (Clontech).
Yeast one-hybrid assay
The assay was carried out as described ( Gao et al. 2013 ). To generate AD-Ghd7 and AD-OsCOL10, the full-length Ghd7 and OsCOL10 coding sequences were amplified by RT-PCR from cv. Minhui 63 and Kita-ake, respectively, and ligated into the pB42AD vector (Clontech) digested with Eco RI. To generate OsCOL10p :: LacZ and Ehd1p :: LacZ reporter genes, various fragments of the OsCOL10 and Ehd1 promoters were amplified from Kita-ake genomic DNA and inserted into corresponding sites in the reporter plasmid pLacZi. Plasmids were co-transformed into yeast strain EGY48. Transformants were grown on SD/Trp–/Ura plates for 48 h and then transferred onto X-gal (5-bromo-4-chloro-3-indolyl-β- d - galactopyranoside) plates for blue color development.
Funding
This work was supported by the National Natural Science Foundation[grant Nos. 31401466 and 31371601]; the National Transformation Science and Technology Program [2013ZX08009-003].
Abbreviations
- CO
CONSTANS
- COL
CONSTANS-like
- CRISPR
clustered, regularly interspaced, short palindromic repeats
- Ehd1
Early heading date 1
- FT
FLOWERING LOCUS T
- GFP
green fluorescent protein
- Ghd7
Grain Number, Plant Height, and Heading Date7
- Hd1
Heading date 1
- Hd3a
Heading date 3a
- LD
long day
- NIL
near-isogenic line
- NLD
natural long day
- qRT-PCR
quantitative real-time PCR
- RFT1
RICE FLOWERING LOCUS T1, RNAi, RNA interference
- SD
short day
- TF
transcription factor
- Ubi
Ubiquitin
Disclosures
The authors have no conflicts of interest to declare.
Acknowledgements
We thank Dr. Masahiro Yano (National Institute of Agrobiological Sciences, Japan) for kindly providing NILs ( Hd1 and Hd3a ), Dr. Atsushi Yoshimura (Kyushu University, Japan) for mutants ( OsphyA , B , C ), Dr. Qifa Zhang (Huazhong Agricultural University, Wuhan) for the NIL Ghd7 / ghd7 NIL pair, and Dr. Chengcai Chu (Chinese Academy of Sciences, Beijing) for the se5 mutant.
References