-
PDF
- Split View
-
Views
-
Cite
Cite
Federica Fiorentino, Matthias Thoms, Klemens Wild, Timo Denk, Jingdong Cheng, Jakub Zeman, Irmgard Sinning, Ed Hurt, Roland Beckmann, Highly conserved ribosome biogenesis pathways between human and yeast revealed by the MDN1-NLE1 interaction and NLE1 containing pre-60S subunits, Nucleic Acids Research, Volume 53, Issue 7, 24 April 2025, gkaf255, https://doi.org/10.1093/nar/gkaf255
- Share Icon Share
Abstract
The assembly of ribosomal subunits, primarily occurring in the nucleolar and nuclear compartments, is a highly complex process crucial for cellular function. This study reveals the conservation of ribosome biogenesis between yeast and humans, illustrated by the structural similarities of ribosomal subunit intermediates. By using X-ray crystallography and cryo-EM, the interaction between the human AAA+ ATPase MDN1 and the 60S assembly factor NLE1 is compared with the yeast homologs Rea1 and Rsa4. The MDN1-MIDAS and NLE1-Ubl complex structure at 2.3 Å resolution mirrors the highly conserved interaction patterns observed in yeast. Moreover, human pre-60S intermediates bound to the dominant negative NLE1-E85A mutant revealed at 2.8 Å resolution an architecture that largely matched the equivalent yeast structures. Conformation of rRNA, assembly factors and their interaction networks are highly conserved. Additionally, novel human pre-60S intermediates with a non-rotated 5S RNP and processed ITS2/foot structure but incomplete intersubunit surface were identified to be similar to counterparts observed in yeast. These findings confirm that the MDN1-NLE1-driven transition phase of the 60S assembly is essentially identical, supporting the idea that ribosome biogenesis is a highly conserved process across eukaryotic cells, employing an evolutionary preservation of ribosomal assembly mechanisms.
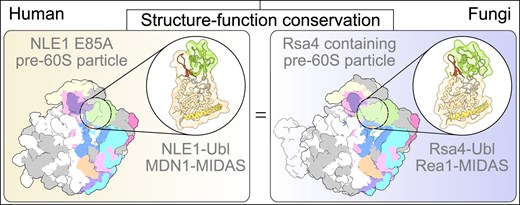
Introduction
Ribosomes are essential cellular machines made of proteins and RNAs which mediate the protein synthesis by decoding the messenger RNA and catalysing the peptide bond formation between amino acids [1]. The mature eukaryotic ribosome (80S) comprises two subunits, the small 40S subunit and the large 60S subunit, which follow separate biogenesis pathways. The assembly pathway of the 60S and 40S subunit starts in the nucleolus [2–5] with the transcription of the ribosomal DNA [6], producing a primary polycistronic rRNA transcript called 47S pre-rRNA in humans (or 35S in yeast), which is the precursor of the mature 18S, 5.8S, and 25S rRNAs in yeast (or 18S, 5.8S, and 28S in humans) [7, 8]. Within the polycistronic rRNA transcript, the 18S, 5.8S, and 25S/28S rRNA sequences are separated by the internal transcribed spacers 1 (ITS1) and 2 (ITS2) and flanked by the 5′ and 3′ external transcribed spacers (5′-ETS and 3′-ETS) [7, 8]. After cleavage at the A2 site (site 2 in human) that is located within the ITS1 the 60S and 40S assembly pathways are separated [7, 9].
The ribosome biogenesis pathway is coupled with several energy-consuming enzymes, like GTPases and ATPases, which govern specific checkpoints and provide directionality to the progression of the process by removing other assembly factors and/or remodelling the pre-ribosome [2–4, 10]. Among them is the AAA+ ATPase Rea1 in yeast (or MDN1 in humans), which is the largest protein in S. cerevisiae, composed of 4910 amino acids (5596 amino acids in human), and conserved from yeast to humans [11]. Rea1 is essential for cell growth and its genetic depletion or specific mutations in yeast [12, 13], or knockdown in humans [14] is lethal or strongly affects cell growth. It is composed of five distinct domains: the N-terminal domain (NTD), AAA + ATPase ring (or ring domain) containing six tandem AAA + protomers (from AAA1 to AAA6), the linker domain, aspartate/glutamate (D/E)-rich domain, and carboxy-terminal Metal Ion-Dependent Adhesion Site (MIDAS) domain [15]. The MIDAS domain is directly involved in the binding to the Ubl domain (ubiquitin-like domain) of its interacting partners Ytm1 (WDR12 in human) and Rsa4 (NLE1, Notchless homolog 1 in human) [13, 16, 17]. The binding of Rea1-MIDAS to Rsa4-Ubl or Ytm1-Ubl is essential for the ATP-hydrolysis dependent reaction resulting in the removal of Ytm1 and Rsa4 from nucleolar and nuclear pre-60S intermediates, respectively [13, 16, 18]. Crystal structures of the MIDAS domain in complex with its ligands showed that the divalent metal ion (Mg2+) is coordinated by five conserved residues of the MIDAS fold (consensus DXSXS-X70-T-X30- (S/T)DG) and the sixth coordinating glutamate residue is provided by the Ubl, an interaction which is very similar to the interaction of the integrins to their ligands [17].
The hyperactivation of ribosome biogenesis, either initiated by oncogenic pathways or by the loss of tumor suppressor genes, is crucial for cancer initiation and progression [19, 20], and the human homolog of Rsa4, NLE1, plays an essential role in cell proliferation, transcription and signal transduction [21]. NLE1 is highly expressed in many cancers, such as melanoma and breast cancer, where it promotes a malignant phenotype through the activation of the PI3K/AKT signalling cascade [22], a pathway known for its role in cell survival, proliferation, and metabolism [19]. Aberrant activation of the PI3K/AKT pathway mediated by NLE1 supports tumor progression and resistance to apoptosis, underlining its significance as a potential therapeutic target. Additionally, other studies have reported that MDN1 is also overexpressed in specific subtypes of breast cancer, especially those resistant to hormonal therapy, suggesting an emerging role for the protein MDN1 as a cancer biomarker, and as a factor favouring the cancerogenic and malignant phenotype and drug resistance in breast cancer [23, 24].
Cryo-electron microscopy (cryo-EM) structures of yeast pre-60S ribosomal particles have shed light on yeast ribosome biogenesis processes [25–34]. More recently, the cryo-EM structures of the corresponding human pre-60S states have been solved [35–37]. However, less is known about the human ribosome assembly pathway during the phase of MDN1 activity and to what extent it is conserved. Therefore, we aimed at a structural and functional characterization of the MDN1–NLE1 interaction and the related assembly intermediates. In this study, we reconstituted the complex consisting of the human MDN1–MIDAS domain and the NLE1–Ubl domain and report its X-ray structure. Moreover, we further investigated the effects of the loss of this interaction in human cells and on the overall architecture of human pre-60S intermediates which we compared with corresponding particles from yeast. Altogether, these findings provide structural and functional insights into the role of the MDN1–NLE1 interaction during human ribosome biogenesis and on the high degree of conservation of the 60S biogenesis pathways between yeast and humans. These insights may pave the way for further exploration of potential therapeutic tools aimed at disrupting this interaction, with the goal of impairing ribosome biogenesis as a novel strategy for cancer therapy.
Materials and methods
MDN1 and NLE1 constructs
Plasmids were constructed using standard recombinant DNA techniques. Amplification of DNA fragments was performed by polymerase chain reaction and transformed in Escherichia coli DH5α cells by heat shock after purification and DNA ligation using standard protocols. All plasmids used for this study are listed in Supplementary Tables S1.
Recombinant protein expression and purification
Recombinant protein expression was carried out in E. coli BL21 cells. Cells were grown in LB medium supplemented with chloramphenicol (34 μg/ml) and either kanamycin (30 μg/ml) or ampicillin (100 μg/ml) and protein expression was induced with 0.5 mM IPTG at 18°C overnight for the MDN1-MIDAS-(His)6 construct or at 23°C for 3 h for the GST-NLE1-Ubl constructs. Cells were harvested by centrifugation, and cell pellets were flash-frozen in liquid nitrogen and stored at −20°C. For protein purification, cell pellets were resuspended in the respective lysis buffer, lysed with a Microfluidizer homogenizer (Microfluidics) and subsequently cleared by centrifugation at 20 000 rpm for 20 min at 4°C.
Recombinant MDN1-MIDAS-(His)6 and GST-NLE1-Ubl were affinity purified using Ni-NTA and GSH beads, respectively, followed by size exclusion chromatography. After incubation of the cleared lysate with either Ni-NTA or GSH resin the beads were centrifuged at 1000 rpm for 1 min at 4°C. The beads were transferred to a column (Bio-Rad), washed three times with 15 ml of the respective wash buffer and eluted with elution buffer. The MDN1-MIDAS-(His)6 eluates were concentrated, centrifuged and applied to a Superdex® 200 26/60 column equilibrated with SEC buffer (Gel filtration ÄKTA system). Peak fractions were pooled, concentrated to 10–15 mg/ml, flash frozen in liquid nitrogen and stored at −80°C. The GST-Rsa4-Ubl eluate was applied to a Superdex® column 75 26/60 column, equilibrated with SEC buffer. Peaks fractions were pooled and concentrated up to 9–11 mg/mL.
Buffers for MDN1-MIDAS-(His)6 purification
Lysis Buffer: 20 mM HEPES, pH 8, 30 mM Imidazole, 500 mM NaCl, 5mM MgCl2, protease inhibitors (SIGMAFAST Sigma-Aldrich S8830).
Wash Buffer: 20 mM HEPES, pH 8, 30 mM Imidazole, 500 mM NaCl, and 5 mM MgCl2.
Elution Buffer: 20 mM HEPES, pH 7.5, 400 mM Imidazole, 500 mM NaCl, and 5 mM MgCl2.
SEC Buffer: 20 mM HEPES, pH 7.5, 150 mM NaCl, 5 mM MgCl2, and 1 mM DTT.
Buffers for GST-NLE1 purification
Lysis Buffer: 20 mM HEPES, pH 8, 150 mM NaCl, 5 mM MgCl2, protease inhibitors (SIGMAFAST Sigma-Aldrich S8830).
Wash Buffer: 20 mM HEPES, pH 8, 150 mM NaCl, and 5 mM MgCl2.
Elution Buffer: 20 mM HEPES, pH 7.5, 150 mM NaCl, 5 mM MgCl2, and 30 mM reduced glutathione.
SEC Buffer: 20 mM HEPES, pH 7.5, 150 mM NaCl, 5 mM MgCl2, and 1 mM DTT.
Protein purification of the MDN1-MIDAS (His)6/ (His)6-NLE1-ubl complex
For the reconstitution of the MDN1-MIDAS-(His)6/ (His)6-NLE1-Ubl complex used for crystallization, a N-terminal (His)6 version of the NLE1-Ubl domain was used. The protein domains were separately overexpressed in E. coli BL21 cells and lysate were mixed and incubated with Ni-NTA beads for 2 h at 4°C. Beads were washed and samples were eluted with buffer supplemented with 400 mM imidazole. The eluate was applied to a Superdex® 200 HiLoad 26/60 column equilibrated with SEC buffer and peak fractions containing stoichiometric MDN1-MIDAS-(His)6/ (His)6-NLE1-Ubl complex were pooled and concentrated to 9 mg/ml with a concentrator (Amicon® Ultra, Millipore). The samples were stored overnight at 4°C and used for crystallization trials.
Crystallization of the MDN1-MIDAS/NLE1-Ubl complex
Crystals were grown at our in-house crystallization platform in a solution of 0.2 M (NH4)2SO4, 18.2% (v/v) polyethylene glycol 300, 0.1 M NaOAc, and 3.5 M NH4Cl using the sitting-drop vapor diffusion at 4°C. Crystals appeared between 1 week and 10 days. Prior to data collection, crystals were harvested in reservoir solution supplemented with 20% glycerol and flash-cooled with liquid nitrogen.
X-ray structure determination
Diffraction data were collected at 100 K at the Deutsches Elektronen-Synchrotron (DESY, Hamburg) on beamline P13 and data processed with XDS [38]. Molecular replacement was performed with the PHENIX suite [39] using the fungal homolog from Chaetomium thermophilum (PDB-ID: 6QTA) as a search model (four MDN1-NLE1 complexes per asymmetric unit). Model building was done by mutating the fungal structure and de novo building of the human-specific regions in COOT [40] and reciprocal space refinement was performed with PHENIX. Validation was done with MolProbity [41]. Figures were prepared using PyMOL (DeLano Scientific). Statistics on data collection and refinement can be found in Supplementary Table S3.
GST-pull down assay
For the in vitro binding assay of the MDN1-MIDAS-(His)6 and GST-NLE1-Ubl domains, GST-NLE1-Ubl WT alone or GST-NLE1-Ubl WT and E85K bound to GSH beads was incubated in binding buffer (20 mM HEPES, pH 7.5, 150 mM NaCl, 5mM MgCl2, 0,01% NP-40) with increasing or fixed amounts of MDN1-MIDAS-(His)6 respectively, on a rotating wheel for 30 min at 4°C, to allow protein interaction. Next, GST-tagged bait protein bound to the beads was pulled down by centrifugation at 2000 rpm for 1 min, the supernatant was collected as flow-through, and beads were washed three times with binding buffer. Bound proteins were then eluted by incubating the beads with elution buffer (10 mM reduced glutathione in 50 mM Tris-HCL, pH 8.0) for 10 min at 4°C. The eluted samples were mixed with SDS sample buffer, boiled for 5 min at 92°C and analyzed by SDS-PAGE to assess the interaction between MDN1-MIDAS-(His)6 and GST-NLE1-Ubl WT and E85K.
Stable cell lines and adherent cell culture
HEK 293 Flp-In T-REx cells (Thermo Fisher) were transfected with the pOG44 plasmid (Invitrogen) and either pcDNA5/FRT/TO-3xFlag-3C-NLE1 WT or pcDNA5/FRT/TO-3xFlag-3C-NLE1 E85A plasmids using PEI. Stable cell lines were generated by selection with Hygromycin B and Blasticidin S following the manufacturer's protocol (see Supplementary Table S2). Adherent 3xFLAG-NLE1 and 3xFLAG-NLE1 E85A HEK 293 Flp-In T-REx cells were maintained in DMEM, supplemented with 10% (v/v) FBS and 1% (v/v) Penicillin/Streptomycin (Gibco) in a humidified atmosphere at 37°C and 5% CO2.
Transient transfection
HEK 293 Flp-In T-REx cells were transfected with pcDNA3.1(+)-C-GFP-eL29 and pcDNA3.1(+)-C-YFP-uS5. Transfection experiments were performed according to manufacturer's instructions using Lipofectamine 2000 (Thermo Fisher). Cells were transfected at 80% confluency.
Confocal fluorescence microscopy
HEK 293 Flip-In T-REx 3xFlag-NLE1-Ubl WT and E85A mutant cells were seeded on poly-lysine coated glass bottom petri dish at high confluency. One day after seeding, cells were transiently transfected with eL29-GFP or uS5-YFP reporter plasmid and incubated for 24 h with 1 μg/ml doxycycline. After induction cells were washed with PBS, fixed with 2% PFA for 15 min at RT and stained with Hoechst 1:1000 (Thermo Fisher). Cells were imaged with a confocal fluorescence microscope (Zeiss Imager Axio Examiner.Z1).
Colony formation assay
HEK 293 Flip-In T-REx 3xFlag-3C-NLE1 WT and NLE1 E85A cells were seeded in 6-well plates and incubated with 1 μg/ml doxycycline. After 5 days of induction, cells were washed with PBS, fixed with cold methanol for 10 min and stained with crystal violet (Sigma-Aldrich) for 2 h. The plates were carefully rinsed with water and photographed with a Nikon camera. Images of the colonies were analysed with ImageJ. The experiment was performed in triplicates and the same background adjustment was applied to both control and target wells.
Viability assay
HEK 293 Flip-In T-REx 3xFlag-3C-NLE1 WT, E85A were seeded in a 96-well plate (1 × 104 cells per well) and induced with 1 μg/ml doxycycline. After 72 h of induction, cells were replaced with fresh medium and 20 μL of CellTiter-Blue® Cell Viability Assay (Promega) was added and incubated for 2 h at 37°C. Then, fluorescence was measured with a plate reader (SpectraMax M5 Multi-Mode Microplate Reader) at 560Ex/590Em.
Isothermal titration calorimetry
Isothermal Titration Calorimetry (ITC) assay was conducted in calorimetry buffer (20 mM HEPES, pH 7.5, 150 mM NaCl, 5 mM MgCl2) using MicroCal ITC instrument (Malvern Panalytical). The experiment was performed with a protein concentration of 20 μM in the sample cell and 200 μM in the syringe. A single ITC assay, performed at the constant temperature of 25°C, consisted of one injection of 0.4 μL and 18 injections of 2 μL each, with a total number of 19 injections of the titrant into the solution in the cell under constant stirring at 750 rpm. The assay was performed in triplicates.
Polyribosome gradient analysis
HEK 293 Flip-In T-REx cell lines were cultured in a 15 cm dish. When cells reached about 40% confluency expression of NLE1 WT or E85A mutant was induced with 0.5 μg/ml tetracycline for 20 h. Cycloheximide at a final concentration of 100 μg/ml was added to the media and cells were treated for 15 min at 37°C. Cells were washed once with PBS supplemented with 100 μg/ml cycloheximide, scrapped from the dish and collected by centrifugation at 300 x g for 5 min. The cell pellet was resuspended in 500 μl lysis buffer (20 mM HEPES-KOH pH 7.5, 100 mM KCl, 10 mM MgCl2, 1 mM DTT, 1x EDTA-free protease inhibitor (Roche), 0.5% IGEPAL CA-630 (Sigma-Aldrich) and passed five times through a 26 gauge needle. Cell debris was removed by centrifugation at 15 000 x g for 15 min. Then, a total of 6 A260 units per sample were separate through a 10–50% sucrose gradient (20 mM HEPES-KOH pH 7.5, 100 mM KCl, 5 mM MgCl2, 1 mM DTT, 10–50% (w/v) sucrose) in a SW 40 Ti rotor (Beckmann Coulter) at 40 000 rpm for 2.5 h.
Purification of 3xFLAG-NLE1 WT and E85A mutant from HEK 293 Flp-in T-REx cells
Cell from 50 plates (15 cm) with 80–90% confluency were resuspended in purification buffer (50 mM Tris pH 7.5, 150 mM NaCl, 5 mM MgCl2, 1 mM DTT) supplemented with 5% glycerol, 1% Triton X-100 and 1x EDTA-free protease inhibitor (Roche), and sonicated five times for 10 s with 30 s breaks on ice in between (Branson Sonifier 250) The lysate was cleared by centrifugation for 15 min at 4000 rpm (Eppendorf 5810 R) and a second time for 25 min at 17 500 rpm at 4°C (Sorvall LYNX 6000). Anti-FLAG M2 agarose beads (Sigma-Aldrich) were added to the cleared lysate and incubated on a turning wheel for 90 min at 4°C. Beads were washed twice with purification buffer supplemented with 0,2% Triton X-100 and two time with purification buffer supplemented with 0.05% octaethylene glycol monododecyl ether (Nikkol). For sample elution 3C protease (homemade) was added and samples were incubated for 1 h at 4°C. Eluates were loaded on a 1 M sucrose cushion containing purification buffer with 0.05% Nikkol and centrifuged in a TLA 120.2 rotor (Beckmann Coulter) for 1 h at 100 000 rpm. The supernatant was removed and the pellet resuspended in purification buffer containing 0.05% Nikkol. Samples were used directly for cryo-EM and analysed on a 4–12% NuPAGE gel (Thermo Fisher). Protein bands were analysed by mass spectrometry.
Cryo-EM imaging and data processing
The sample was applied onto R3/3 carbon support copper grids with 3 nm continuous carbon (Quantifoil) using a Vitrobot Mark IV (FEI) at 4°C and 95% humidity and incubated on the grid for 45 s, blotted for 3 s and plunge frozen in liquid ethane. Data was collected on a Titan Krios microscope equipped with a K2 summit direct electron detector (Gatan) operated at 300 kV with a pixel size of 1.045 Å. MotionCor2 was applied to sum, dose-weight and motion correct the collected movies [42] and the contrast transfer function parameters of the summed micrographs were estimated with CTFFIND4 [43]. Micrographs were manually inspected and 7087 micrographs (8098 micrographs in total) were used for further processing.
Particles were picked in Relion 3.1 [44] with the Autopick function and extracted with a pixel size of 2.090 Å/pixel and a box size of 200 × 200 pixel. 2D classification was performed in cryoSPARC [45] and good 2D class averages were used for Ab-Initio Reconstruction followed by Homogeneous Refinement. Particles were imported to Relion 3.1 and re-extracted with a pixel size of 3.135 Å/pixel. Elaborate 3D classification without image alignment was performed and is described in detail in Supplementary Fig. S3. Particles of the final classes were extracted without down sampling at a pixel size of 1.045 Å/pixel (box size 500 × 500 pixel) and reimported to cryoSPARC. Homogeneous Refinement with defocus and global CTF Refinement was performed and the resolution of flexible parts was further increased using Local Refinement. Composite maps of the consensus map and the individual local refinements were generated with ChimeraX [46]. Details are given in Supplementary Figs S3–S5.
Model building and refinement
The following molecular models were used as initial models: PDB-ID: 6LSS [37], PDB-ID: 6ZM7 [47], and PDB-ID: 7UOO [48]. Models were rigid body fitted into the cryo-EM density map with ChimeraX [46]. The best resolved State 5 with 2.84 Å was used for model building. Models of protein factors were generated with SWISS-MODEL [49] and Alphafold [50]. Models were rigid body fitted into the cryo-EM density and manually corrected with Coot [49]. The rRNA was manually build in Coot using the yeast rRNA (PDB-ID: 7UOO) and the human rRNA (PDB-ID: 6LSS and 6ZM7) as initial models. The generated State5 model was used as initial model for the States 1A, 1B, 2, and 3 and was manually adjusted with Coot. In case of State 1B the model of the L1 stalk and H79 was used from PDB-ID: 6ZM7. The model was rigid body fitted and corrected with Coot. Final molecular models were reals-space refined in Phenix using secondary structure restraints [51]. Figures of cryo-EM maps and models were generated with ChimeraX [46].
Results
Structure of the human MDN1-MIDAS/NLE1-Ubl complex
To initiate studies on the interaction between the human 60S biogenesis factors MDN1 and NLE1 (Fig. 1A), we reconstituted the complex between the MDN1-MIDAS (residues 5287 to 5596) and NLE1-Ubl (residues 11 to 104) domains in vitro. For MDN1-MIDAS, an internal loop (eII) containing the unstructured nuclear localization sequence (NLS, residues 5337–5376) and dispensable for the respective fungal complex from Chaetomium thermophilum (ct) [17] was replaced by a short Gly-Ser-Gly linker (Supplementary Fig. S1A–C). The stable complex allowed for X-ray structure determination at a resolution of 2.3 Å by molecular replacement using the fungal counterpart as template (PDB-ID: 6QTA) [17] (Fig. 1B and C, Supplementary Table S3 and Supplementary Fig. S2A). The overall architecture reveals a typical MIDAS–ligand interaction centered around a magnesium ion (Fig. 1D). The MIDAS domain assumes an α/β Rossman-like fold and houses the metal-binding site. In comparison to the MIDAS-core fold as typically found in cell-adhesive integrins, MDN1 contains three Rea1-specific elements (eI to eIII) (Fig. 1B and C, Supplementary Fig. S1A) [17]. The N-terminal element eI forms a kinked α-helix wrapping around the MIDAS-core with unknown function and low sequence conservation (single conserved anchoring tryptophan). Element eII, here substituted with Gly-Ser-Gly for the structural analysis, corresponds to a flexible loop harbouring the highly conserved NLS and is essential for intramolecular positioning on the AAA+ ring and NLE1 release from the pre-ribosome [17]. Element eIII forms a β-hairpin that is known to undergo structuring and a large induced fit movement upon Ubl-domain binding. Although not highly conserved, eIII has been shown to play an important role in 60S biogenesis in yeast (Supplementary Fig. S1A) [17]. NLE1 forms a typical β-grasp Ubl-fold consisting of a five-stranded anti-parallel β-sheet surrounding a single α-helix [52]. This grasp is extended in the MDN1/NLE1 complex by the β-hairpin of element eIII that interacts via β-completion. Interaction with the MIDAS-core is established by the N-terminal region of NLE1-Ubl including strand β1 and by an extended β4-β5 loop in direct contact with the metal ion. The overall interface comprises 1200 Å2 similar as found for the fungal complex [17]. The resemblance of the human and fungal MIDAS domains is closer (38.4% identity, r.m.s.d. of 1.55 Å for 229 residues) than of the Ubl domains (21.4% identity, r.m.s.d. of 2.0 Å for 84 residues) (Fig. 1B-C and Supplementary Fig. S2B). Both MIDAS-core domains (without elements eI to eIII) share structural similarity to the integrin I domains (r.m.s.d. of 2.3 Å for 125 residues of MDN1 and human integrin-αLβ2). Importantly, also the metal-dependent interaction resembles integrin–ligand complexes, although the extracellular integrin ligands (e.g. collagen, fibronectins or ICAMs) have different folds [53]. NLE1-Ubl superposes well with other Ubl-domains like human WDR12 (PDB-ID: 6P0Q, r.m.s.d. of 1.53 Å for 75 residues, Ytm1 in yeast), the second important interacting partner of MDN1-MIDAS during 60S pre-ribosome biogenesis [16, 17, 54, 55].
![Structure of the human MDN1-MIDAS/NLE1-Ubl complex. (A) Scheme of the ATP dependent NLE1 release by MDN1 during 60S ribosome biogenesis (left panel) and domain organization of MDN1 and NLE1 (right panel). (B) Overall structure of the MDN1-MIDAS/NLE1-Ubl complex. Termini are numbered and Rea1-specific MIDAS elements eI to eIII are highlighted. (C) Comparison with a typical integrin–ligand complex (PDB-ID: 1T0P, left) [75] and with the respective fungal Rea1-MIDAS/Rsa4-Ubl complex from Chaetomium thermophilum (PDB-ID: 6QTA, right) [17]. (D) The MIDAS site showing the central coordination of the magnesium ion. Water molecules are shown in aquamarine. Octahedral coordination in the first shell is shown in black lines and in the second shell in grey. (E) ITC analysis of the MDN1-MIDAS/NLE1-Ubl interaction. (F) The interface (shaded) of MDN1-MIDAS element eIII with NLE1-Ubl helix α1. Hydrophobic contacts and a central π-cation stacking are detailed. (G) Rotational differences in the domain orientations between the human and the fungal (ct) complex involving MIDAS element eIII. (H) Overall view of the domain interface for the human (coloured) and C. thermophilum (grey) complex highlighting relevant differences. Respective rotations and involved residues are indicated. The structural environments of the central Mg-ligating glutamate residues (E85 in NLE1) is encircled. The extra helical turn in the fungal structure is highlighted in light blue.](https://oup.silverchair-cdn.com/oup/backfile/Content_public/Journal/nar/53/7/10.1093_nar_gkaf255/1/m_gkaf255fig1.jpeg?Expires=1750183475&Signature=CB289Qxeoz8tDWwcD4Pv38SBpjPuMMowPHGuDTzpWlWv55-v9U5YCmCrZ5Ev2~so7krV7dQAdAgDGf7h17dIsERs2LYFaDB~lR~5G6wuzsGf6ZFtIPxk5C1od4Qbq5qDobgY9R2WTyJGw~3AfalRK4akUmlOBpGwor699F3uzlUu2e9zLmFEwFhm2P~Wv1SjZofjybrg0ZLPyIsi1mYc0-8JrARcetcXuLnNppYTxn9dNk75jF-GxsmPkbL9vIhsOBlb2vDEy1OgoENxZH2eDOmP75f0o74VBsvwcLR-P0jxJ2tEe-RcrI6bIeVS54o5iTiXI~LHnGf6SNCDntf3SA__&Key-Pair-Id=APKAIE5G5CRDK6RD3PGA)
Structure of the human MDN1-MIDAS/NLE1-Ubl complex. (A) Scheme of the ATP dependent NLE1 release by MDN1 during 60S ribosome biogenesis (left panel) and domain organization of MDN1 and NLE1 (right panel). (B) Overall structure of the MDN1-MIDAS/NLE1-Ubl complex. Termini are numbered and Rea1-specific MIDAS elements eI to eIII are highlighted. (C) Comparison with a typical integrin–ligand complex (PDB-ID: 1T0P, left) [75] and with the respective fungal Rea1-MIDAS/Rsa4-Ubl complex from Chaetomium thermophilum (PDB-ID: 6QTA, right) [17]. (D) The MIDAS site showing the central coordination of the magnesium ion. Water molecules are shown in aquamarine. Octahedral coordination in the first shell is shown in black lines and in the second shell in grey. (E) ITC analysis of the MDN1-MIDAS/NLE1-Ubl interaction. (F) The interface (shaded) of MDN1-MIDAS element eIII with NLE1-Ubl helix α1. Hydrophobic contacts and a central π-cation stacking are detailed. (G) Rotational differences in the domain orientations between the human and the fungal (ct) complex involving MIDAS element eIII. (H) Overall view of the domain interface for the human (coloured) and C. thermophilum (grey) complex highlighting relevant differences. Respective rotations and involved residues are indicated. The structural environments of the central Mg-ligating glutamate residues (E85 in NLE1) is encircled. The extra helical turn in the fungal structure is highlighted in light blue.
MIDAS domains comprise a consensus motif with five conserved residues (DXSXS–T–D; D5390, S5392, S5394, T5461, D5497 in MDN1) that provide five coordination sites to the central Mg2+ ion, while the sixth coordination is provided by the Rsa4/NLE1 or Ytm1/WDR12 ligands in trans contributing in general a glutamate (E85 in NLE1) [53] (Fig. 1D, Supplementary Fig. 2A and B). Two water molecules complete the first coordination shell and are held in place by hydrogen bonds to the second coordination shell (D5390, D5497, and carbonyl of R5499 of MDN1). As the metal ion is embedded in the MIDAS domain, the single ligand carboxylate group (E85) needs to be presented on an exposed surface, which in NLE1 corresponds to the β4-β5 loop of NLE1. Of importance, this loop is complementary in shape and charge only with the metal ion bound (Supplementary Fig. 2C), affirming the MIDAS definition. The MDN1-NLE1 interaction is strong with a KD value in the low nanomolar range (5.5 nM) as measured by ITC (Fig. 1E). Reverse-charge mutation of E85 to lysine is sufficient to completely abolish the MDN1-NLE1 interaction (Supplementary Fig. S1D and E).
While the MIDAS-core interaction is rather hydrophilic and includes several water-mediated contacts, the interaction of the Rea1-specific β-hairpin (eIII element) with Ubl-helix α1 is mainly hydrophobic and contains a central π–cation stacking interaction (MDN1 F5545, NLE1 R43) (Fig. 1F, Supplementary Fig. S2B). Furthermore, the hairpin is generally stabilized by proline residues (three residues in MDN1). Pictorially spoken, the hairpin forms the back rest of a MIDAS chair with a sitting Ubl-domain. In this sense, the chair forms a cantilever and the hairpin together with the Ubl-domain show rotational differences (12°) with respect to the MIDAS seat comparing the MDN1 and ctRea1 complexes (Fig. 1G). In addition, the Ubl-domains are rotated sidewise (15°) around helix a1. However, the Mg2+-coordination by the invariant Ubl-glutamate (E85) and the position of the ligating carboxylate stay unaffected, thus forming a pivot point for the rotation (Fig. 1H). The rotational differences are enabled by respective amino acid deviations in the interface. Towards the hairpin, MDN1 MIDAS is extended, while on the opposite, it is reduced and tightly fixed to the N-terminus of NLE1. Interestingly, the central glutamate of the Ubl-domains is presented in a different structural context. In ctRsa4, it is part of a helical turn containing two additional residues (PF) (Supplementary Fig. S2B), with the phenylalanine being compensated for in NLE1 by an opposing leucine residue (Fig. 1H). The insertion sterically prevents Ubl rotation according to the human complex.
Taken together, the human MDN1-MIDAS/NLE1-Ubl pre-ribosome biogenesis complex forms a high-affinity and metal-based integrin-MIDAS-like ligand interaction with Rea1-specific elements and an adjustable interface when compared to the fungal Rea1/Rsa4 complex. Yet, the overall architecture remains essentially identical between yeast and humans, indicating a high degree of evolutionary conservation.
Function of the MDN1-NLE1 interaction in human cells
Taking into consideration that mutation of the critical residue E85 within the NLE1-Ubl abolishes the MDN1–NLE1 interaction similar to what was described for the Rea1-MIDAS–Rsa4-Ubl interaction in yeast, we investigated its effect in human cells [13]. For this purpose, we expressed full-length NLE1 (wild-type or WT) or the NLE1 E85A mutant, each modified with an 3x FLAG-3C tag at the N-terminus and under the control of the TET-On promoter, in HEK 293 Flip-In T-REx cells. Initially, we probed the ability of these cells to proliferate upon overexpression of the NLE1 E85A mutant by analysing cell confluency for 5 days in medium with or without doxycycline by staining with crystal violet. Cells induced with doxycycline and overexpressing NLE1 WT did not show difference in cell number compared to cells without expression (Fig. 2A). In contrast, cells overexpressing the NLE1 E85A mutant displayed a substantial impairment of cell growth when compared to uninduced cells or cells overexpressing NLE1 WT (Fig. 2A and B). This suggests that mutation of the key residue E85 within the Ubl domain of NLE1, causing impairment of the MDN1-NLE1 interaction, exerts a dominant-negative effect on human cell growth similar to what was observed in yeast [13].
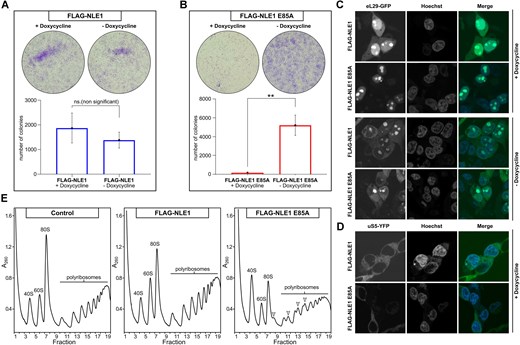
Overexpression of the NLE1 E85A mutant is dominant lethal in human cells. (A-B) Cells overexpressing FLAG-NLE1 E85A (+ doxycycline) show reduced cell number compared to uninduced mutant cells (- doxycycline) and NLE1 WT cells with or without overexpression. Colony formation assay performed in Flp-In 293 T-REx NLE1 WT (A) and NLE1 E85A mutant (B) cells with and without doxycycline induction. Cells stained with crystal violet and the number of cells shown as mean of three experiments. Error bars represent standard deviation of three independent experiments. An unpaired t test was conducted to assess statistical significance (ns, not significant; *, P < 0.05; **). (C-D) Confocal microscopy monitoring the subcellular localization of eL29-GFP (C) and uS5-YFP (D) in NLE1 WT and E85A mutant cells induced with doxycycline and uninduced. Nuclei are counterstained with Hoechst. (E) Polysome gradient analysis of NLE1 WT and NLE1 E85A mutant cells compared to untagged Flp-In cells (control). Whole cell lysates were separated by ultracentrifugation on a 10–50% sucrose gradient. The migration of the 40S, 60S, and 80S is labelled accordingly. Half-mers appearing in the FLAG-NLE1 E85A induced cells are labelled with arrows.
Next, to assess the effects of the dominant-negative mutant on 60S ribosome biogenesis, we expressed the ribosomal reporter protein eL29 (Rpl29), tagged with GFP [56], in our HEK 293 Flip-In T-REx cell lines that expressed either NLE1 WT or NLE1 E85A. Subsequently, the eL29-GFP subcellular localization was followed by confocal microscopy. In cells overexpressing NLE1 WT, eL29-GFP exhibited a typical distribution, with locations in the cytoplasm and nucleolus. However, in cells overexpressing NLE1 E85A, the cytoplasmic eL29-GFP signal was barely detectable, while the nucleolar signal remained. The localization of eL29-GFP in NLE1 WT and NLE1 E85A cells without doxycycline induction did not show such differences (Fig. 2C). In contrast, when the YFP-tagged reporter for the 40S subunit, uS5 (Rps2) was tested [57], the NLE1 E85A mutant did not cause an inhibition of the nuclear export of uS5-YFP, suggesting that disturbing the MDN1-NLE1 interaction in human cells specifically affects 60S biogenesis and nuclear export (Fig. 2D). Consistent with this data, ribosomal subunit and polysome profile analysis by sucrose gradient centrifugation of whole cell lysates revealed a specific reduction of 60S subunits when compared to 40S subunits, and formation of a half-mer phenotype in the polysome fractions of the sucrose gradient upon induction of the NLE1 E85A mutant (Fig. 2E). Taken all these findings together, the NLE1 E85A mutant blocks the assembly of 60S subunits in human cells, similar to what has been previously observed for orthologous mutations in the Ubl domain of Rsa4 in yeast [13].
Cryo-EM analysis of nucleoplasmic NLE1-E85A containing pre-60S intermediates
Based on our biochemical and crystallographic data described above, we next aimed at a structural characterization of the pre-60S particles that accumulate in the NLE1 E85A mutant using cryo-electron microscopy (cryo-EM). Therefore, we affinity-purified the inducible 3xFLAG-tagged NLE1 E85A construct from HEK 293 Flp-In T-REx cells under native conditions and analysed the final sample by SDS-PAGE and Coomassie staining (Fig. 3A and Supplementary Fig. S1F). Subsequent cryo-EM analysis and extensive 3D classification revealed typical pre-60S particles in different states, but always carrying NLE1, with the best resolved intermediate named State 5 at 2.84 Å resolution (Fig. 3B, Supplementary Figs 3–5 and Supplementary Table S4). This particle showed a pre-rotated 5S RNP but lacked the prominent ITS2/foot structure. However, we also identified a small population representing an essentially identical particle (State 4), but with an partly processed ITS2/foot (Fig. 3C). Apart from the completely processed foot, State 5 showed a high overall resemblance to the Nog2/Arx1 pre-60S particle that was described earlier in yeast [48, 58] (Fig. 4A). Yet, the architectural similarity is not limited to the overall architecture of the intermediates, it is also present in molecular detail of interactions in many regions as described below.
![Cryo-EM structures of NLE1 E85A containing pre-60S intermediates. (A) SDS-PAGE of the affinity purified NLE1 E85A containing pre-60S particles. Ribosome biogenesis factors identified by mass spectrometry are labelled. (B) Coloured cryo-EM density map and molecular model of the Nog2-like State 5 in two orientations. (C) Density map of the pre-60S State 4 coloured according to (B) and close-up of the ITS2-Foot area. The Foot model of PDB-ID: 8FL2 [35] was rigid body fitted into the density. Parts of the model without corresponding density are shown transparent.](https://oup.silverchair-cdn.com/oup/backfile/Content_public/Journal/nar/53/7/10.1093_nar_gkaf255/1/m_gkaf255fig3.jpeg?Expires=1750183475&Signature=mOBI~uziZiY-l4KXAOA-0IVQg6QwArqYXJdEj93SSWNwy8HpbvLNNu3VunnYdWVl9FDPwKrdn1rx9b3Mtcvk8FxL9ezdRjwdHRaaLl4YXnu9l~nQM1t6222wgPtIQmNywGPtxnce~1z8Se9me8C1qfRLf9XDeei2fa5N-8Rug0j7dEMralMP7q6-~o~9BUTYOLhkrjgM6MpuGD6VGH~jcBaIWIC-GNocHikgo4gHAR0fhK5rVZr1EleJ6~A7rLeOJ~ilwikDjJniM~0JAr9qHoTV7f-YFS-5qU6flGSQ86Xm0oY4DZ9w8c4~76OSgSFyWM~P3sFcxUI1PkgUSXkLvQ__&Key-Pair-Id=APKAIE5G5CRDK6RD3PGA)
Cryo-EM structures of NLE1 E85A containing pre-60S intermediates. (A) SDS-PAGE of the affinity purified NLE1 E85A containing pre-60S particles. Ribosome biogenesis factors identified by mass spectrometry are labelled. (B) Coloured cryo-EM density map and molecular model of the Nog2-like State 5 in two orientations. (C) Density map of the pre-60S State 4 coloured according to (B) and close-up of the ITS2-Foot area. The Foot model of PDB-ID: 8FL2 [35] was rigid body fitted into the density. Parts of the model without corresponding density are shown transparent.
![Comparison of human NLE1 E85A and yeast Rsa4 containing pre-60S intermediates. (A) Comparison of the human pre-60S State 5 and the Nog2 like state from yeast (PDB-ID: 7UOO) [48]. (B–J) Close-ups comparing structural features of the NLE1-E85A (State 5) and the Rsa4 containing pre-60S particles (PDB-ID: 7UOO) from human and yeast, respectively. (B) The pre-rotated CP containing the 5S RNP (5S rRNA, uL5, uL18) and 28S rRNA Helices 80–88 (H80-88), shown together with RPF2-RRS1 and NLE1 (human) or Rpf2-Rrs1 and Rsa4 (yeast). (C-D) Close-up views of the CP focussing on the C-terminal extensions (CTE) of RPF2/Rpf2 (C) and RRS1/Rrs1 (D). (E) NLE1-E85A and Rsa4 from human and yeast, respectively, with its interacting partners uL18, uL11 and NSA2/Nsa2. (F) Interacting ribosome biogenesis factors of GNL2/Nug2. The N-terminal extensions (NTE) and C-terminal domains (CTD) of GNL2/Nug2 are highlighted. (G-H) Magnification views of the GTPase GTPB4/Nog1 focusing on the interacting ribosome biogenesis factors (G) and 28S rRNA H89 (H). Close-ups of the C-terminus of GTPB4/Nog1 occupying the PET are shown (G). (I) The Zn-finger containing ribosome biogenesis factor ZNF593/Bud20 interacting with 28S rRNA H62/H63, GNL2/Nug2 and RLP24/Rlp24. (J) Close-ups of the PET, highlighting the missing Arx1 homolog EBP1 on NLE1 E85A containing human pre-60S particles. Ribosomal proteins of the exit are labelled.](https://oup.silverchair-cdn.com/oup/backfile/Content_public/Journal/nar/53/7/10.1093_nar_gkaf255/1/m_gkaf255fig4.jpeg?Expires=1750183475&Signature=mYTgwPpAUmQYLhd8ZH65v5MDsa4VPxK3Svq25huaJB6OzbY8qUA9rhGdqIkmnJ7h02uxS7hR6t3ULEoesm2rZPcfy57Q7uoRC~CZDZsdpLVQzOvWJcZnWmlaSrHeiZcSHLjAT~ZroHpr-p6ahfAM4Ouegtt9SeAey0aRteAoj~hK0e0Kkfu0xuUT1YjyO0BtxNnILk8eo68yNhuLZDyFs2HTxK~V~hc3xJqZpgE1nskFe6wg8UPmm7nvv-bBpkEq8uxIZ8ag0Upz6BUoLWDeOY2CMZxkx~mZksWciImy~zWlm8rZiYD6H4nfMifgU0V1kk3XqXkBIMoejF4UNmHnmw__&Key-Pair-Id=APKAIE5G5CRDK6RD3PGA)
Comparison of human NLE1 E85A and yeast Rsa4 containing pre-60S intermediates. (A) Comparison of the human pre-60S State 5 and the Nog2 like state from yeast (PDB-ID: 7UOO) [48]. (B–J) Close-ups comparing structural features of the NLE1-E85A (State 5) and the Rsa4 containing pre-60S particles (PDB-ID: 7UOO) from human and yeast, respectively. (B) The pre-rotated CP containing the 5S RNP (5S rRNA, uL5, uL18) and 28S rRNA Helices 80–88 (H80-88), shown together with RPF2-RRS1 and NLE1 (human) or Rpf2-Rrs1 and Rsa4 (yeast). (C-D) Close-up views of the CP focussing on the C-terminal extensions (CTE) of RPF2/Rpf2 (C) and RRS1/Rrs1 (D). (E) NLE1-E85A and Rsa4 from human and yeast, respectively, with its interacting partners uL18, uL11 and NSA2/Nsa2. (F) Interacting ribosome biogenesis factors of GNL2/Nug2. The N-terminal extensions (NTE) and C-terminal domains (CTD) of GNL2/Nug2 are highlighted. (G-H) Magnification views of the GTPase GTPB4/Nog1 focusing on the interacting ribosome biogenesis factors (G) and 28S rRNA H89 (H). Close-ups of the C-terminus of GTPB4/Nog1 occupying the PET are shown (G). (I) The Zn-finger containing ribosome biogenesis factor ZNF593/Bud20 interacting with 28S rRNA H62/H63, GNL2/Nug2 and RLP24/Rlp24. (J) Close-ups of the PET, highlighting the missing Arx1 homolog EBP1 on NLE1 E85A containing human pre-60S particles. Ribosomal proteins of the exit are labelled.
High similarity between the human pre-60S intermediate and the equivalent yeast state
As known from nucleoplasmic pre-60S particles from yeast, the human 5S RNP is found in its immature pre-rotated conformation chaperoned by the RPF2-RRS1 heterodimer (Fig. 4B) [28, 58]. Highly similar to yeast, the 5S RNP/RPF2-RRS1 complex is anchored to the pre-60S particle through the C-terminal extensions (CTE) of RPF2 and RRS1 (Fig. 4C and D): the C-terminus of RPF2 binds between the GTPase domain of GNL2 and the immature H69-H70 of the 28S rRNA (Fig. 4C) while the CTE of RRS1 has intrinsic interactions with immature CP helices H80-88 (Fig. 4D). The bait protein NLE1 E85A binds next to the pre-rotated 5S RNP with direct interactions with uL18 (Rpl5) and uL11 (Rpl12). Again similar to yeast [58, 59], the interaction partner NSA2 (Nsa2) binds with an internal loop to the top side of the NLE1 ß-propeller (Fig. 4E) and with a highly conserved tyrosine residue (Y89 in H.s., Y90 in S.c.) reaching into the central pore of the NLE1 β-propeller (Rsa4). This tyrosine residue is essential in yeast and a Y90A mutation prevents complex formation [59]. Also, the NSA2-interacting GTPase GNL2 (Nog2/Nug2 in yeast) shows several interactions, e.g. with GTPB4, ZNF593 and RPF2, that essentially resembled those described in yeast (Fig. 4F). Moreover, the GTPase GTPB4 (Nog1 in yeast) has a highly similar mode of interaction with the human pre-60S when compared to yeast intermediates. The C-terminal extension shows extensive contacts with the biogenesis factors eIF6 and RLP24 and the very C-terminus inserts into the peptide exit tunnel (PET) (Fig. 4G). In addition, the conformation of the NTD and GTPase domains of GTPB4 and its mode of rRNA helix H89 contact resemble that of its yeast counterpart Nog1 (Fig. 4H). Also the nearby Zn-finger protein ZNF593 (Bud20), which was shown to act as a shuttling factor in yeast [60, 61], interacts with rRNA H62-H63, the C-terminal domain (CTD) of GNL2 and with the eL24 (Rpl24) placeholder RLP24 in the same way as in yeast (Fig. 4I).
Besides these overall similarities, there are also a few differences between these human and yeast pre-60S particles, most noticeably being the lack of the tunnel exit interacting protein EBP1 (Arx1 in yeast) in the human complexes (Fig. 4J). This agrees with previous observations of human nuclear pre-60S intermediates lacking EBP1, a protein that has apparently evolved in humans to mainly fulfil a function in the cytoplasm and on mature 60S and 80S ribosomes as anti-association factor [37, 62, 63].
Taken together, the overall architecture, assembly factor composition and interaction network in the human pre-60S particles are nearly identical when compared to equivalent states in yeast. Only minor differences, besides the absence of the Arx1 homolog EBP1, exist in specific areas: Rsa4 for example contains an N-terminal extension that is absent in NLE1 and reaches towards Rrs1. In turn, the human RRS1 contains a specific N-terminal helix which interacts with the Ubl domain of NLE1 and probably replaces this interaction (Fig. 4B).
Identification of intermediates with immature intersubunit surface
Unexpectedly, the majority of our identified pre-60S intermediates (State 1A-D, State 2 and 3) had an immature intersubunit surface (ISS) while already containing the stably bound 5S RNP in its pre-rotated conformation (Fig. 5). We placed these intermediates into a logical order according to an increasing number of stably incorporated ribosomal proteins and rRNA moieties. Among these states, the States 1A–D lack density for the complete 28S rRNA domains III and IV and parts of domain II and V. Thereby, they display an ISS and a PET that is a still open, differing only in the presence and orientation of the L1 stalk (Supplementary Fig. S6A and B and Supplementary Fig. S7). In contrast, the further matured States 2 and 3 have fully incorporated rRNA domain III, parts of domain IV and nine additional ribosomal proteins, resulting in the formation of the ISS and a closed PET. The State 3 particle contains in addition the ribosomal protein eL38 which coincides with further compaction of this intermediate (Fig 5, Supplementary Fig. S6C and Supplementary Fig. S7). In the subsequent States 4/5 incorporation of H62/H63 of domain IV and the interacting biogenesis factor ZNF593 has occurred (Figs 3B and C and 5, Supplementary Fig. S6C). Finally, State 6 represented the furthest progressed intermediate displaying a completely rotated 5S RNP. Here, the assembly factors involved in this rotation, SDA1 (Sda1 in yeast) and the RIX1 complex, were still present, while the RPF2–RRS1 complex had already dissociated (Fig. 5). Identification of this state as the most mature intermediate is in full agreement with the failure of NLE1 E85A removal by the MDN1 ATPase which would follow as the subsequent maturation step.
![Sequence of the different NLE1 E85A containing pre-60S states. Pre-60S states discovered in this study arranged according to the ribosomal proteins and rRNA domains incorporated. Ribosome biogenesis factors are coloured and labelled and density maps are shown in two orientations (left and top right panels). The molecular models of the incorporated rRNA domains are shown (bottom right). The states are compared to State H (PDB-ID: 8FL0) [35].](https://oup.silverchair-cdn.com/oup/backfile/Content_public/Journal/nar/53/7/10.1093_nar_gkaf255/1/m_gkaf255fig5.jpeg?Expires=1750183475&Signature=aLG7w3hhVnyfRGiODxMgw~jhJlb-k5zGgBmG~7CPKAaG562C0d7a01PtyiBX9PTZNJPFFmr4jCCHNmScIHYJZ~hja1HXqLYH4yw5cqvkYkuDVnZ-US0wqQYWec~ugKrh15GN6JaaOax4xpIQCETPh3PA6aeLKKiYxEOpvunKIvEaEzcohEiQgV12QND~BpgQrn~IKhjP2gihQIarQtaTtlSQjaPO0KGzOTxGFG5aKGKsuzCKyE--81SPEHimLaOa1U8dotksu7nX~svSWpZ4c26iBhwBulstuhhCNbJ54EtvyQi6O5MN62bnAu0xNeieD06-~XTAWEY89vTTmiCqcw__&Key-Pair-Id=APKAIE5G5CRDK6RD3PGA)
Sequence of the different NLE1 E85A containing pre-60S states. Pre-60S states discovered in this study arranged according to the ribosomal proteins and rRNA domains incorporated. Ribosome biogenesis factors are coloured and labelled and density maps are shown in two orientations (left and top right panels). The molecular models of the incorporated rRNA domains are shown (bottom right). The states are compared to State H (PDB-ID: 8FL0) [35].
When comparing our series of pre-60S intermediates with the previously described human 60S particles purified from endogenously tagged MK67I cells (Nop15 in yeast) [35], we noticed that our State 1A can be clearly placed after the described State H. Also, this particle displayed a stable bound, non-rotated 5S RNP and contained e.g. RPF2-RRS1, NLE1, GTBP4, RLP24, and stable incorporated rRNA domains I, II, V, and VI (Figs 5 and Supplementary Fig. S7). However, similar to our intermediates, it is missing rigidly incorporated rRNA domains III and IV but also the central core domain 0 and the complete 5.8S rRNA together with the interacting ribosomal proteins which results in a lack of the ISS and PET. Our States 1A–D display already slightly further matured rRNA, and together with our following states, States 2–5, can close the gap to the next previously described state, State I [35], This State resembles our State 6 with all rRNA domains readily formed, the 5S RNP in rotated conformation and the human RIX1 complex and SDA1 bound to the ISS while still containing the ITS2-Foot (Fig. 5 and Supplementary Fig. S7). Our identified states may thereby reveal in more detail the transitions that can occur between this 60S biogenesis intermediates, in particular for the maturation of the rRNA forming the ISS. Our particles can thus be arranged in a sequential order with respect to structural transitions and fill the gap between the previously observed States H and I [35]. Moreover, similar states have been observed in the lower eukaryotes S. cerevisiae (uL4 Δ (aa63-87) and C. thermophilum (Rsa4-E117D) displaying immature ISS/PET but already stably incorporated 5S RNP [64, 65]. It is therefore plausible that these particles may indeed belong to a physiological assembly pathway. If so, this may indicate that an rRNA unfolding event can occur upon the nucleolus-to-nucleoplasm transition or that alternative/partially parallel maturation pathways can be employed during this phase. Such parallel pathways have been reported before for both, lower eukaryotes and humans, showing for example that the order of 5S RNP rotation and ITS2/foot processing is not strictly interdependent [35, 36, 65, 66], again documenting the high degree of conservation of pathways in these evolutionary distant species.
Discussion
Here we present the X-ray structure of the essential MDN1–MIDAS/NLE1–Ubl interaction and a series of human pre-60S cryo-EM structures harbouring the NLE1 E85A mutant. Overall, our data reveal the high degree of evolutionary conservation between the human 60S biogenesis pathway and pathways observed in lower eukaryotes like S. cerevisiae or C. thermophilum, thereby further validating their significance as model systems for this pathway.
It should be noted, however, that some of our pre-60S intermediates with incomplete ISS could also represent degradation products which accumulated due to the NLE1 mutation-triggered arrest of 60S biogenesis. This would explain why preceding nucleolar particles observed by us and others [25, 26, 29, 31, 35, 36, 67] harbour already parts of the rRNA and ribosomal proteins that are missing in State H (and in some of the here described intermediates). However, so far, we have not found any degradation factors associated with our particles, and also in cells with unperturbed ribosome assembly pathways similar pre-60S intermediates with incomplete ISS have been observed [35]. Unfortunately, it is not possible for us to clearly distinguish between on-pathway assembly intermediates and accumulating degradation products.
In any case, recent structural studies of the human 40S biogenesis pathway comprising early 90S/SSU processome and later nucleoplasmic or cytoplasmic intermediates also revealed highly conserved components, interaction networks and structural transitions [68–71]. Thus, despite the differences in rRNA length, its more complex pre-rRNA processing and the higher number of known assembly factors in humans, the structural basis and the fundamental principles underlying ribosome biogenesis appear to be highly conserved during evolution. This may also apply to the release mechanism mediated eventually by the MDM1–NLE1 interaction: Given the evolutionary conservation of these factors, it is reasonable to hypothesize that human MDN1 triggers NLE1 removal through a mechanism akin to its yeast homolog, Rea1. In yeast, Rea1 utilizes ATP hydrolysis to generate mechanical force, extracting its substrates from the pre-60S subunit via large-scale conformational transitions, including rotational shift of its MIDAS domain [18, 30, 72].
The observed overall conservation compares well to other fundamental cellular pathways involving complex RNA-protein assemblies such as nuclear transport, co-translational protein targeting to the ER or ribosome associated quality control, which all turned out to have diverged structurally only to a limited degree during evolution from lower eukaryotes to humans. To that end it will be important for future studies of ribosome biogenesis to identify differences between the organisms and characterize their functional significance. One well known example is the signalling of impaired 60S assembly into the p53 pathway in metazoans mediated by accumulating 5S RNPs that fail to be incorporated into the nascent pre-60S subunit [73, 74]. The use of mutant factors could provide a useful tool for the identification of rare or otherwise short-lived assembly intermediates. Furthermore, introducing specific halt points in maturation progression might also give insight into assembly quality control and degradation mechanisms as well as potential alternative or secondary assembly pathways. Moreover, with the ongoing progress of cryo-EM technology, including better detectors, faster data acquisition and improved data processing, it can be anticipated that more such underrepresented states will be identified in the future. It is safe to assume that despite the overall conservation of the ribosome biogenesis pathways more fundamental differences between lower eukaryotes and humans remain to be discovered.
Acknowledgements
We thank Otto Berninghausen, Susanne Rieder and Charlotte Ungewickel for cryo-EM data collection and Jürgen Kopp for access to the protein crystallization platform, a core facility of University of Heidelberg. We acknowledge access to beamline P13 at the Deutsches Elektronen-Synchrotron (DESY, Hamburg/Germany) and thank the staff for valuable support. We are grateful to Thomas Fröhlich for MS analysis of the NLE1 E85A purification and to Ulrike Kutay for supplying the eL29-GFP and uS5-YFP plasmids, to Walter Nickel for access to the cell culture facility, to Valentin Mitterer and Giuseppe La Venuta for valuable scientific discussions and to Selene Cordeiro for the media support.
Author contributions: F.F. carried out recombinant protein purification and in vitro binding assays with help of M.T., as well as, confocal microscopy and colony formation assays. Polysome gradients were analysed by J.Z. Crystallization of the MDN1-MIDAS/NLE1-Ubl complex and ITC measurements were performed by F.F. and K.W. X-ray structure modelling, refinement and interpretation was performed by K.W. and I.S. Flip-In cell lines were generated by M.T. and the purification of human pre-60S particles was carried out by M.T. and T.D. Cryo-EM data was processed by M.T. and molecular models were generated by M.T. with help of J.C. and T.D. Cryo-EM models were interpretated by M.T., T.D. and R.B. M.T., K.W., and F.F. prepared the figures and F.F., M.T., K.W., I.S., E.H., and R.B wrote the initial manuscript. All authors discussed the results and commented on the manuscript.
Supplementary data
Supplementary data is available at NAR online.
Conflict of interest
None declared.
Funding
The study was funded by the European Research Council grants ADG 885711 HumanRibogenesis (R.B.) and ADG 741781 GLOWSOME (E.H.), the German Research Foundation grant HU363/12-1 (E.H.), and the Leibniz Program SI586/6-1 (I.S.). Funding to pay the Open Access publication charges for this article was provided by ERC.
Data availability
The MDN1-MIDAS/NLE1-Ubl X-ray structure has been deposited to the Protein Data Bank (PDB) with the accession code 8QL1. The cryo-EM maps including composite maps, consensus maps and local refined maps have been deposited to the Electron Microscopy Data Bank (EMDB): EMD-53090, EMD-19324, EMD-19323, EMD-19322, EMD-19321, EMD-19318 for State 1A; EMD-53092, EMD-19320, EMD-19319, EMD- 19317, EMD-19316, EMD-19315 for State 1B; EMD-19329, EMD-19302, EMD-19301, EMD-19300, EMD-19299, EMD-19298 for State 1C; EMD-19328, EMD-19297, EMD-19296, EMD-19295, EMD-19294, EMD-19293 for State 1D; EMD-53091, EMD-19314, EMD-19313, EMD-19312, EMD-19311 for State 2, EMD-53196, EMD-19310, EMD-19309, EMD-19308, EMD-19307 for State 3; EMD-19327, EMD-19292, EMD-19291, EMD-19290, EMD-19288 for State 4; EMD-19330, EMD-19306, EMD-19305, EMD-19304, EMD-19303 for State 5; EMD-19326, EMD-19287, EMD-19289 for State 6 and EMD-19325 for State 7. The corresponding molecular models were deposited to the Protein Data Bank with the accession codes: 9QER for State 1A, 9QET for State 1B, 9QES for State 2, 9QIW for State 3 and 8RL2 for State 5.
References
Author notes
The first two authors should be regarded as Joint First Authors.
Comments