-
PDF
- Split View
-
Views
-
Cite
Cite
Elzbieta E Iwaniuk, Thuwebat Adebayo, Seth Coleman, Caitlin G Villaros, Irina V Nesterova, Activatable G-quadruplex based catalases for signal transduction in biosensing, Nucleic Acids Research, Volume 51, Issue 4, 28 February 2023, Pages 1600–1607, https://doi.org/10.1093/nar/gkad031
- Share Icon Share
Abstract
Discovery of oxidative catalysis with G-quadruplex•hemin constructs prompted a range of exciting developments in the field of biosensor design. Thus, G-quadruplex based DNAzymes with peroxidase activity found a niche as signal transduction modules in a wide range of analytical applications. The ability of nucleic acid scaffolds to recognise a variety of practically meaningful markers and to translate the recognition events into conformational changes powers numerous sensor design possibilities. In this work, we establish a catalase activity of G-quadruplex•hemin scaffolds. Catalase activated hydrogen peroxide decomposition generates molecular oxygen that forms bubbles. Observation of bubbles is a truly equipment free signal readout platform that is highly desirable in limited resources or do-it-yourself environments. We take a preliminary insight into a G-quadruplex structure—folding topology—catalase activity correlation and establish efficient operating conditions. Further, we demonstrate the platform's potential as a signal transduction modality for reporting on biomolecular recognition using an oligonucleotide as a proof—of—concept target. Ultimately, activatable catalases based on G-quadruplex•hemin scaffolds promise to become valuable contributors towards accessible molecular diagnostics applications.
INTRODUCTION
Over the past decades, artificial DNA scaffolds with enzymatic activity (DNAzymes) emerged as powerful signal transduction tools in chemical and biochemical analysis (1,2). Intrinsically stable, non-toxic, and affordable, small nucleic acid scaffolds align with requirements set forth by the World Health Organization (WHO) to operate under limited resources environments (so called ASSURED (Affordable, Sensitive, Specific, User-Friendly, Robust and rapid, Equipment free, Deliverable) criteria (3–6)).
A class of DNAzymes, G-quadruplexes•hemin constructs that mimic protein peroxidases introduced in 1998 by Dipankar Sen et al. (7) has advanced into the chemical/biochemical analysis field as translators of target recognition events into separation free readouts. The scaffolds are compatible with a range of detection platforms (e.g. optical (8,9) and electrochemical (10)) including truly equipment-free colorimetric (7,11,12) and liquid-to-gel transition readouts (13). The equipment-free transduction of molecular recognition events is an essential requirement for design of ASSURED-compliant diagnostic devices. However, an interpretation of colour intensity and hue is contingent on lighting conditions and/or on the colour vision proficiency of the observer. Liquid-to-gel transition set up (13) employs acrylamide that is highly toxic and, therefore, is problematic to handle by end-users. Therefore, alternative equipment-free signal readout platforms are still actively searched after.
In this work, we extend the reach of G-quadruplex•hemin scaffolds as signal transduction tools and build up their utility as activatable catalases. Protein catalases catalyse hydrogen peroxide decomposition (2 H2O2 → 2 H2O + O2 (g)). The reaction produces molecular oxygen that can form bubbles. Observing bubbles is a straightforward and easy-to-interpret route towards instrument-free visualization of a readout (14) that does not require a scientific background, equipped lab, colour vision proficiency and, therefore, can easily expand towards wide scale applications suitable for everyone ages 2 and up.
With no prior knowledge of a catalase activity by nucleic acids scaffolds, we hypothesize that heme peroxidase mimicking G-quadruplex•hemin constructs may function as catalases. Indeed, in protein world, both heme-peroxidases and catalases belong to the same class of metalloenzymes that operate off heme's catalytic activity and their catalytic cycles are similar (7,15–21) (simplified catalytic cycle is included in Scheme 1). Thus, a critical step in both enzymatic pathways is the formation of Compound I (Scheme 1, process 1). In case of catalases, Compound I reacts with a second molecule of H2O2 yielding O2, H2O, and the regenerated enzyme (process 2) (19). In peroxidases, Compound I reacts with a reducing substrate (RS) to form Compound II (process 3) followed by regenerating resting enzyme (process 4) (15). Recent experimental proofs (21) and molecular dynamic simulations (19) demonstrating the possibility of a direct (i.e. without participation of a protein frame) transfer of two hydrogen atoms from H2O2 to the oxoferryl group (process 2) do support our hypothesis. Moreover, recently reported electrochemical measurements prove G-quadruplex-hemin ability to catalyse H2O2 reduction to water (22,23). Additionally, notoriously promiscuous catalytic behaviour of G-quadruplex DNAzymes (i.e. activity as NADH oxidases (24), NADH peroxidases (24), chelatases (25,26), catalysis of an oxidation of thiols to disulphides (27,28)) gives our hypothesis a further reassurance.
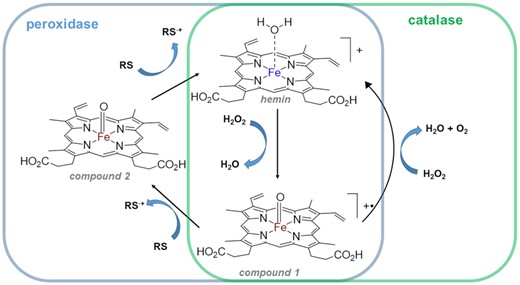
Simplified catalytic cycles of peroxidases and catalases. ‘RS’ = reducing substrate. Iron (III) is blue, iron (IV) is brown.
In this work, we establish the catalase activity of G-quadruplex•hemin scaffolds and demonstrate its utility as a signal transduction platform in biomolecular recognition events on a proof-of-concept example of an oligonucleotide target.
MATERIALS AND METHODS
All the chemical reagents were obtained from established commercial suppliers (i.e. Sigma-Aldrich (St. Louis, MO), Fisher Scientific (Pittsburg, PA) etc.) unless specifically mentioned below. Nuclease-free water used for preparation of DNA solutions was obtained from IDT (Coralville, IA). 10 × PBS Buffer (pH 7.4) was purchased from Thermo Fisher Scientific (Waltham, MA); UltraPure 1M Tris–HCl (pH 7.5) Buffer was purchased from Invitrogen (Grand Island, NY) Single stranded DNAs were obtained from IDT (Coralville, IA) and reconstituted with nuclease-free water. Oligonucleotide sequences are included in Table S1. pH-meter calibration buffers (4.00 ± 0.01, 7.00 ± 0.01 and 10.00 ± 0.02) were purchased from Fisher Scientific. DNA TriDye Ultra Low Range DNA Ladder was purchased from New England Biolabs (Ipswich, MA). SYBR Gold staining dye was purchased from Thermo Fisher Scientific. Acrylamide:bis-Acrylamide (29:1) solution for PAGE was obtained from BioRad (Hercules, CA). Hydrogen Peroxide (30%) was purchased from Fisher Scientific. Hemin was purchased from Thermo Fisher Scientific. TRIS buffers supplemented with NaCl and KCl were prepared in our laboratory.
G-quadruplex preparation
G-quadruplexes were prepared by denaturing an approximately 150 μl of a 10–50 μM oligonucleotide solution in an appropriate buffer at 95.0°C for 5.0 min followed by overnight cooling to room temperature. The actual concentration of G-quadruplexes was established post-equilibration using 260 nm absorption values obtained by UV–vis and extinction coefficients provided by IDT.
UV–vis measurements
Cary 4000 UV-Vis spectrophotometer (Agilent Technologies, Santa Clara, CA) was used for all UV measurements. Typically, measurements were performed on 800-μl solution aliquots using a 10 mm optical path quartz cuvette. For establishing oligonucleotide concentrations, ∼1 μM dilutions were analyzed.
Measuring pH
ThermoOrion pH-meter (Model 420) was used throughout the studies. The pH meter was calibrated against fresh aliquots of pH calibration buffers before each use.
Circular dichroism (CD) spectrometry (Supplementary Figure S1)
CD spectra were collected on AVIV Model 215 Circular Dichroism Spectrometer (Aviv Instruments Inc., Lakewood, NJ) from 200 to 320 nm. Solutions containing equilibrated G-quadruplexes (prepared as described in G-quadruplex preparation subsection above) were diluted to ∼5 μM with PBS buffer at pH of 7.5. Quartz cuvettes with a 10-mm light path were used for all CD measurements. CD spectra for split structures were recorded in the presence of the equivalent amount of target T1. To correct for a signal from DNA duplex in target/G-quadruplex constructs, we subtracted the control ‘duplex’ (target T1 + 9:3 split T control L + 9:3 split T control R) spectrum from the corresponding split G-quadruplexes + target T1 spectra.
The instrument output (θ, mdeg) was converted into molar ellipticity (per strand, (Δϵ, mdeg·M−1·cm−1) using the equation Δϵ = θ/(M × L × 32 980), where M is oligonucleotide strand concentration (mol/L) and L is optical path length (cm).
Hemin solutions
A 2.8 mM hemin's stock was prepared by dissolving 9 mg of hemin in 5 ml of DMSO. A 250 μM working solution was diluted from the stock in DMSO.
G-quadruplex–hemin binding (Figure 1, Supplementary Figure S2)
Hemin stock (in DMSO) was spiked into PBS (pH 7.5) and the initial (t = 0) time point was recorded. Next, equilibrated GI–GVII (prepared as discussed in ‘G-quadruplex preparation’, vide supra) were added to hemin. The final concentration of hemin was 1 μM and of an oligonucleotide—500 nM. Absorption spectra were recorded from 300 to 500 nm every 5 min for 60–75 min.
Catalase activity of full G-quarduplexes GI–GVII (Figure 2, Supplementary Figure S3)
For naked eye observations, we mixed 40 μl of equilibrated 10–50 μM G-quadruplex solution (described above in G-quadruplex preparation) and 8 μl of a hemin working solution (250 μM in DMSO) in a 20-ml glass scintillation vial. The mixture was equilibrated for 20 minutes at ambient conditions to let hemin interact with G-quadruplex. Then 1952 μl of 30% hydrogen peroxide was added (bringing total H2O2 concentration to 29.3%); and bubble observations were started. Addition of hydrogen peroxide is a ‘0 min’ point in Figure 1 and Supplementary Figure S3. After indicated time points, bubbles on the surface of solution were observed either with a naked eye; or some images (view from above) were taken with a cell phone camera (iPhone XR). An equivalent volume of buffer instead of G-quadruplex solution was used in control samples.
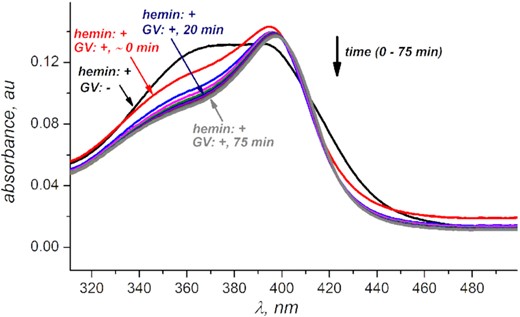
Bathochromic shift and increase in intensity of hemin's Soret band indicates hemin's de-aggregation upon interacting with G-quadruplex V. Data for other quadruplexes indicate to a similar trend (Supplementary Figure S2). Hemin concentration is 1 μM, GV concentration is 500 μM in PBS buffer at pH 7.50.
Gel electrophoresis (Supplementary Figure S4)
Gels for polyacrylamide gel electrophoresis (PAGE) were prepared in our laboratory. Typically, 4 μl of ∼4 μM oligonucleotide solutions mixed with glycerol (to yield ∼17%) were separated in 0.5× TBE running buffer on a 20% gel at 150 V for ∼2 h at 4°C using a VWR (Radnor, PA) vertical PAGE system and a power source. Gels were stained with SYBR Gold (3 μl dye in 200 ml of 0.5× TBE buffer) for 20 min and imaged with Bio-Rad EZ Gel Doc imager (Hercules, CA). Single stranded oligonucleotides with no G-quadruplex forming sequences (21-, 22- and 24-nt) were injected as unfolded controls.
Thermal folding studies (Supplementary Figure S5)
Sequences GI–GVII (at 500 nM) in PBS (pH 7.50) were denatured at 80°C for 30 min in cuvette compartment of UV–vis spectrometer followed by cooling to 1–10°C at a 0.2°C/min rate. An Absorbance at 295 indicative of G-quadruplex folding state was measured each 1°C.
Catalase activity upon target detection (Figure 3, Supplementary Figure S6) and quantitative analysis (Supplementary Figure S7)
Split G-quadruplexes were prepared by denaturing of ∼50 μM solutions (with exact concentrations established by UV–vis) of a left arm strand, a right arm strand, and a target in a PBS buffer (pH 7.5) at 95.0°C for 5.0 min, slowly cooling down to room temperature, and equilibrating for additional 10 h. Further sample preparation proceeded as described above for full G-quadruplexes GI–GVII but with the split G-quadruplex/target hybrids. For controls, the samples with equivalent amounts of left and right arm strands but no target were equilibrated and treated in an identical fashion.
For microscopic observations (Figure 3), images were taken with BZ-X800 Analyzer (Keyance, Japan). The solutions were prepared in a 96-Well Plate and images were taken in Bright Field with a 4× objective. The reaction volumes were scaled down to yield the same concentrations and reagent ratios as indicated above in a total volume of 300 μl. Sample preparation/observation timelines were equivalent to those used for naked eye observations.
RESULTS AND DISCUSSION
G-quadruplex design and environmental conditions
To design activatable catalases, we focus on G-quadruplexes with at least three G-quartets because those are known to form tight complexes with hemin (29–31). Since folding topology may influence catalytic properties of G-quadruplexes (29), as a starting point, we choose structures with enough flexibility to fold either into a parallel or an antiparallel conformation. Thus, our core oligonucleotide GI with a sequence (d(GGGTTA)3GGG) is a human telomere repeat with three G-quartets reported to form a parallel or antiparallel structure under various conditions (32) and to enhance a peroxidase activity of hemin (33). Considering similarities in catalytic cycles of peroxidases and catalases (Scheme 1, vide supra for discussion), we expect the core quadruplex to mimic a catalase. Further, we deliberately introduce some incremental modifications into the core's loops and added overhangs to generate four other three-G-quartet sequences GII–GV. As controls, we designed two G-quadruplex sequences (GVI and GVII) that cannot form ‘canonical’ three-G-quartet structures essential for hemin activation in DNAzymes (29–31). All sequences are included in Table S1.
To select buffer conditions, we take into account that: (i) the hemin-catalyzed hydrogen peroxide decomposition is the most efficient at pHs around 7–8 (34); (ii) G-quadruplex folding requires a presence of Na+ or K+ ions (35,36); (iii) G-quadruplex/hemin DNAzymes show higher peroxidase activity at pH 7–8 (37,38); (iv) optimal activity of catalases is observed over pH range of 7–11 (39). Therefore, for initial evaluations we selected three buffering systems that satisfy the criteria: TRIS + 50 mM KCl (pH 8.0), Tris + 50 mM NaCl (pH 8.0) and PBS (pH 7.5).
Catalase activity of G-quadruplexes
Hemin binding
To ensure that G-quadruplexes bind hemin (a condition for the activation of G-quadruplex DNAzymes (40)), we monitored the Soret band on the hemin adsorption spectra. Increase in intensity and bathochromic shift observed (Figure 1, Supplementary Figure S2) is consistent with hemin binding a G-quadruplex scaffold (38,41–42). We found no significant changes to the Soret band beyond the first 20 minutes of hemin/G-quadruplex interaction (for all quadruplexes except GVII that appears to be binding hemin much slower). Therefore, we incorporate at least 20 min G-quadruplex•hemin equilibration time before introducing hydrogen peroxide into all experimental conditions discussed below.
Catalase activity
To evaluate the catalase activity, we exposed GI–GVII to hydrogen peroxide in the presence of hemin in all three buffering systems (vide supra). To ensure hemin binding, we equilibrated G-quadruplex/hemin mixture for 20 min before adding H2O2. Upon preliminary optimization of H2O2 concentration (over a 3–27.3% range), G-quadruplex concentration (over a 100–2000 nM range), hemin : G-quadruplex ratio (over a 5:1 to 1:2 range), aqueous/DMSO ratio (over a 0.1–1% range), we came up with optimal conditions consisting of 200–500 nM of a G-quadruplex oligonucleotide, below 0.5% of DMSO, 2:1 to 5:1 of hemin/G-quadruplex ratio, and ∼29% of H2O2 (final concentration after all the components are mixed with a conventionally available solution of 30% H2O2). Our observations taken in the optimized conditions (Figures 2 and Supplementary Figure S3) indicate: (i) control experiments (in absence of any G-quadruplex) in both versions of TRIS buffer demonstrate substantially higher background bubbling levels compared to PBS; (ii) quadruplexes GI–GV produce bubbles in amounts exceeding corresponding controls under all buffering conditions evaluated; (iii) GVII (in all conditions) and GVI (in PBS) do not produce bubbles in amounts that observably exceed control levels. Those observations clearly indicate that quadruplexes GI–GV noticeably enhance the catalase activity of hemin with the best signal to background ratio obseved in PBS buffer at pH 7.5.
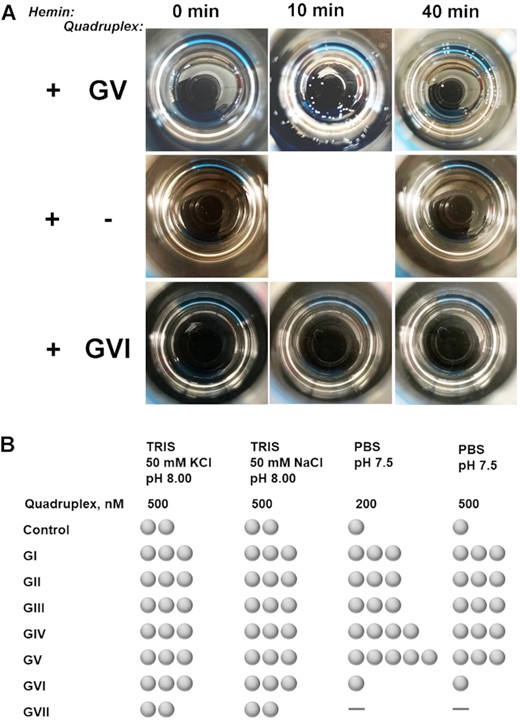
Representative images (A) and summary (B) of bubbles formation in presence of quadruplexes GI–GVII. Samples were prepared in PBS (pH 7.5) and contained equilibrated G-quadruplexes at indicated levels, hemin at 1 μM, and 29.3% of hydrogen peroxide. Control consisted of all the components except a G-quadruplex. Images in (A) are views from above on 20-ml scintillation vials taken with a cell phone camera. Observations in (B) are taken 30 min after adding hydrogen peroxide. One ‘bubble’ symbol in (B) corresponds to 1–3 bubbles observed, two to 4–8 bubbles, three to 8–12 bubbles, four to 12–18 bubbles, five to almost all the surface area covered (more than 18), dash corresponds to zero (0) bubbles.
Correlation between the catalase activity and G-quadruplex folding state and topology
Rational development of G-quadruplex based catalases requires understanding G-quadruplex structure–activity link. To establish the link, we evaluate the folding stability and folding topology of GI–GVII under the selected conditions.
Evaluation of thermal folding profiles (Supplementary Figure S5) indicate mostly folded conformation of GI and GV over ambient temperature range (20–25°C), a mixture of folded and unfolded confirmations for GII–GIV and GVI, and mostly unfolded state of GVII. On non-denaturing gel electropherograms (Supplementary Figure S4), bands for GI, GII, GIV, GV and GVII migrate father than corresponding non-structured strands of identical lengths. Migration of GIII and GVI does not differ from controls. Some variances observed between gels and thermal profiles (e.g. folded state for GVII is evident on gel but no folding appears on thermal profiles or no folding of GIII and GVI on gel but partial folding follows from the corresponding thermal profiles) may originate from low temperature of gel separations (4°C) and/or the presence of glycerol in samples. Importantly, both experiments point towards consistently stable folded state of GV, a sequence that shows the highest catalase enhancement in PBS (Figure 3).
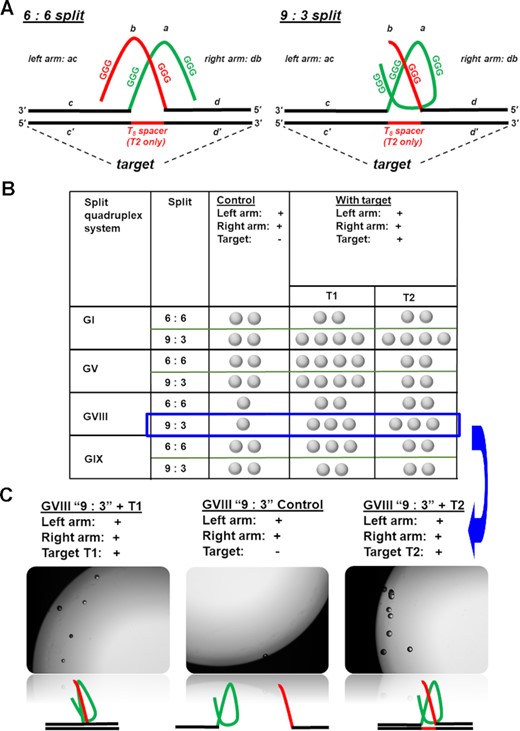
(A) Activation of catalase activity of quadruplexes GVIII and GIX in presence of an oligonucleotide target requires splitting guanine-rich sequences in two parts. We evaluate two different split patterns: a symmetric (6:6) and an asymmetric (9:3). (B) Results over different split and target configurations. Each sample in PBS buffer (pH 7.50) consisted of two quadruplex ‘arms’ (R and L) and target (all at 500 nM concentrations), hemin at 1 μM, and hydrogen peroxide at 29.3%. Control consisted of all the components except an oligonucleotide target. Observations were taken 30 min after adding hydrogen peroxide. One ‘bubble’ symbol in the table correspond to 1–3 bubbles observed, two to 4–8 bubbles, three to 8–12 bubbles. (C) Representative image of response to the target T1 (left) and T2 (right) against a control (no target, centre) taken with 4× objective using Keyance microscope. The samples were prepared in a 96-well plate. The total sample volume was 300 μl. Additionally, conventional cell phone images for GV ‘9:3’ splits are included in Figure S6.
To get a preliminary insight into a correlation between the folding topology and catalase activity, we interrogate CD spectra of all the G-quadruplexes (Supplementary Figure S1). Importantly, the CD spectra for GI, GII, GIV and GV are consistent with spectra reported for telomeric G-quadrplex sequences in phosphate buffer: maximum between 290 and 295 nm, minimum around 265 nm, and maximum around 240–250 nm (32). The features agree well with antiparallel folding topology (43,44). The quadruplexes do not indicate substantial folded confirmation either on gel (GIII and GVI) or on thermal folding (GVII) have features indicating the presence of single stranded species (higher signal or even maximum around 260 nm). The CD spectra of GIII and GVII do not correlate with any the three clearly defined topological systems (parallel, antiparallel or hybrid/3 + 1) (43,44) indicating either to a mixture of conformations or a substantial fraction of nonstructured strands;); CD spectrum of GVI has some features consistent with hybrid topology.
Overall, on example of sequence GV, we conclude that the stability at ambient conditions and antiparallel folding topology correlate with catalase activity enhancement. However, considering the complexity in G-quadruplexes’ folding topology and its contingency on oligonucleotide sequence and ionic environments (45), we are currently designing more thorough studies to understand the structure/property correlation.
Activatable G-quadruplex catalases
To enable a target-triggered bubble production, we engineer a target-templated G-quadruplex assembly. We split G-quadruplexes into two fragments with a vision that the fragments that are separated from each other are unlikely to form a quadruplex. When deliberately brought into proximity, the fragments assemble into a quadruplex structure. We make the quadruplex assembly contingent on the presence of a target oligonucleotide strand. For the proof-of-concept demonstrations, we derive a target from a rat prolactin promoter sequence (used as a model in (46) and our prior publications (47,48)). We split G-quadruplexes into left (L) and right R ‘arms’ (Figure 3, domains a and b) and extend the ‘arms’ with target recognition domains (c and d) that are complementary to fragments c’ and d’ on a target. Therefore, in the presence of target, c/c’ and d/d’ hybridization brings domains a and b in a close proximity triggering a G-quadruplex assembly. It is known that split design may influence the effectiveness G-quadruplex reassembly (49). Therefore, as a starting point, we evaluate different split types, symmetric (6:6) and assymetric (9:3). In the symmetric (6:6) split both arms contain two three - guanine stretches while in the assymetric split, the right arm contains one three - guanine stretch while the left arm contains three such stretches (Figure 3A). The type of assymetric split (9:3) has been demonstrated as beneficial against one with one three – guanine stretch on left arm and three stretches on the right arm (50). To follow the design considerations, we obtained four split G-quadruplex systems: two based on GI and GV (as most stable in ambient conditions) and two other systems, GVIII and GIX (Table S1, Figure 3A). We evaluate two target conformations: target T1 with domains c’ and d’ adjacent to each other and target T2 with domains c’ and d’ separated by a 8-nucleotide ‘spacer’.
Evaluation of the activatable setups shows that asymmetric 9:3 splits perform better (GI and GVIII) or similarly (GV) when tested against symmetrical 6:6 splits (Figures 3 and Supplementary Figure S6). The finding is consistent with previous reports that asymmetrically split G-quadruplexes demonstrate higher peroxidase activity and form tighter complexes with protoporphyrin IX (a compound structurally similar to hemin) (49–52). Also, we did not observe a significant difference in target configuration except for GV demonstrating clear preference for T1 with adjacent hybridization domains over T2 with a spacer between hybridization arms spaced.
Activatable catalases, their limitations, and future studies
The G-quadruplex/hemin based activatable catalases promise to evolve into a new equipment-free detection platform. Currently, we established their utility at 200 nM concentrations. While many nucleic acid biomarkers are relevant at lower concentrations (53), recent progress in isothermal amplification techniques (54) (including ones that are ASSURED-compliant) will enable effective equipment-free detection of many relevant biomolecular targets.
Further, we hypothesize that lower detection levels can be achieved even without upfront amplification. Indeed, we demonstrated a link between an increased stability of G-quadruplexes (on an example of GV) and catalase activation. Considering that GII–GIV appear to be partially folded at ambient conditions (based on thermal profiles in Figure S5) but still yield a noticeable signal to background ratios (Figure 2), we assume that lower concentrations of quadruplexes may be sufficient (only fractions of GII–GIV are folded). Therefore, we expect that stable quadruplexes may be effective at much lower concentration.
Moreover, we are very enthusiastic about extending the catalase system into a field of quantitative analysis. The target-assembly activatable system (split) is compatible with a stoichiometric approach for quantitative analysis of biomolecules we developed earlier (47,48). We have preliminary demonstrated that the approach enables naked eye quantitative analysis of a target T1 at 500 nM level (Supplementary Figure S7). Further refinements of the detection scheme are currently under way in our lab.
CONCLUSIONS
In conclusion, we demonstrate that G-quadruplex•hemin constructs are capable of mimicking protein catalases and, as such, to activate hydrogen peroxide decomposition. The reaction produces molecular oxygen that, when released in form of bubbles, serves as a promising visual signal readout platform for molecular recognition events.
We design activatable G-quadruplex•hemin-based catalases that produce an easily observable signal readout in the presence of an oligonucleotide target. However, considering a wide range of target recognition possibilities by nucleic acid scaffolds (55), our work expands the reach of DNAzymes as sensor design platforms (56); we specifically target ASSURED-compliant applications.
Overall, the significance of activatable catalases can extend beyond pure analytical applications into therapeutic area where reactive oxygen species scavengers are in a high demand (57).
We preliminarily establish structure–function correlation for G-quadruplex•hemin catalase mimics and found that enhancement of hemin's catalase activity requires stable G-quadruplex structures with at least three G-quartets. Further, we demonstrate that the catalase activity of G-quadruplex•hemin scaffolds correlate with antiparallel folding topology. However, the work in this direction is to be continued considering that in the world of G-quadruplex•hemin peroxidases parallel folds are the ones that yield the most efficient DNAzymes (40).
DATA AVAILABILITY
All the data that support this article are included within the main text and Supplementary Information available online.
SUPPLEMENTARY DATA
Supplementary Data are available at NAR Online.
ACKNOWLEDGEMENTS
We acknowledge College of Liberal Arts and Sciences of Northern Illinois University for providing research infrastructure and start-up funds for IVN. We thank Professor James Horn from NIU for help with CD measurements.
FUNDING
National Institutes of Health [1R15GM135796-01 to I.V.N.]. Funding for open access charge: National Institutes of Health.
Conflict of interest statement. None declared.
Comments