-
PDF
- Split View
-
Views
-
Cite
Cite
Shinichiro Ando, Nicholas J. Sarlis, Jay Krishnan, Xu Feng, Samuel Refetoff, Michael Q. Zhang, Edward H. Oldfield, Paul M. Yen, Aberrant Alternative Splicing of Thyroid Hormone Receptor in a TSH-Secreting Pituitary Tumor Is A Mechanism for Hormone Resistance, Molecular Endocrinology, Volume 15, Issue 9, 1 September 2001, Pages 1529–1538, https://doi.org/10.1210/mend.15.9.0687
- Share Icon Share
Abstract
Patients with TSH-secreting pituitary tumors (TSHomas) have high serum TSH levels despite elevated thyroid hormone levels. The mechanism for this defect in the negative regulation of TSH secretion is not known. We performed RT-PCR to detect mutations in TRβ from a surgically resected TSHoma. Analyses of the RT-PCR products revealed a 135-bp deletion within the sixth exon that encodes the ligand-binding domain of TRβ2. This deletion was caused by alternative splicing of TRβ2 mRNA, as near-consensus splice sequences were found at the junction site and no deletion or mutations were detected in the tumoral genomic DNA. This TRβ variant (TRβ2spl) lacked thyroid hormone binding and had impaired T3-dependent negative regulation of both TSHβ and glycoprotein hormone α-subunit genes in cotransfection studies. Furthermore, TRβ2spl showed dominant negative activity against the wild-type TRβ2. These findings strongly suggest that aberrant alternative splicing of TRβ2 mRNA generated an abnormal TR protein that accounted for the defective negative regulation of TSH in the TSHoma. This is the first example of aberrant alternative splicing of a nuclear hormone receptor causing hormonal dysregulation. This novel posttranscriptional mechanism for generating abnormal receptors may occur in other hormone-resistant states or tumors in which no receptor mutation is detected in genomic DNA.
THYROID HORMONE SYNTHESIS is tightly regulated by a negative feedback system involving the hypothalamus, pituitary, and thyroid gland. In most forms of hyperthyroidism, elevation of T3 and T4 represses secretion of TSH by the pituitary gland. However, in patients with TSH-secreting pituitary tumors (TSHomas), this negative feedback is disrupted, as TSHomas overproduce TSH in the face of elevated serum T3 and T4 levels (1, 2). Furthermore, TSH secretion cannot be stimulated by TRH. These patients typically have physical signs and symptoms of thyroid hormone excess. Additionally, TSHomas present almost invariably as macroadenomas and can cause visual and vascular complications. The mechanism for this loss of negative regulation of TSH secretion by thyroid hormone in TSHomas is not known but may involve a defect in signaling via thyroid hormone receptors (TRs).
TRs are members of a family of nuclear hormone receptors that include the steroid hormone, vitamin D, and retinoic acid receptors (3, 4). These receptors have a variable amino terminus, a central DNA-binding domain, and a carboxy-terminal ligand-binding domain. TRs bind to their thyroid hormone response elements, usually located in the promoter regions of target genes, and regulate transcription by interacting with coactivators and corepressors (5). In positively regulated target genes, coactivators mediate hormone-induced activation, while corepressors, nuclear receptor corepressor (NCoR) and silencing mediator of retinoic acid and thyroid hormone receptor (SMRT), mediate transcriptional silencing. In negatively regulated target genes, which are not as well characterized, corepressors activate the glycoprotein hormone α-subunit and TSHβ genes, whereas coactivators such as steroid receptor coactivator-1 (SRC-1), glucocorticoid receptor interacting protein 1, thyroid receptor activator molecule 1, and activator of thyroid and retinoic acid receptors enhance the T3-dependent negative regulation of glycoprotein hormone α-subunit gene (6, 7).
There are two distinct TR genes, TRα and TRβ. The TRα gene generates two proteins, TRα1 and a carboxy-terminal splice variant, c-erbAα2, that does not bind thyroid hormone. The TRβ gene generates two isoforms, TRβ1 and TRβ2, via promoter choice (8). TRβ1 and TRβ2 proteins have identical DNA- and ligand-binding domains, but differ at their amino termini. Although TRα1, TRβ1, and c-erbAα2 have widespread expression, TRβ2 has tissue-selective expression in the anterior pituitary gland, hypothalamus, and developing brain (9, 10). Recently, TRβ2-selective knockout mice have been generated, which manifest elevated serum TSH and thyroid hormone levels (11). This finding suggests that TRβ2 may be the critical TR isoform that negatively regulates TSH secretion.
Patients with the inherited syndrome of resistance to thyroid hormone (RTH) also have elevated serum T3 and T4 concentrations and normal or elevated TSH level (12–14). These patients generally have point mutations in one of the alleles of the TRβ gene that results in a mutant TR that cannot bind T3 or regulate transcription but can nonetheless bind to thyroid hormone response elements of target genes. The dominant negative activity of the mutant TRβ on wild-type TR reduces thyroid hormone suppression of the two genes that generate TSH subunits: glycoprotein hormone α-subunit and TSHβ (15, 16). Given the precedence for TRβ mutations in RTH patients, we performed mutational analysis of TRβ isolated from a surgically resected TSHoma. Surprisingly, we detected a splice variant of TRβ mRNA that translates into an abnormal TRβ2 protein that is unable to bind T3. This abnormal TRβ2 was transcriptionally inactive and disrupted the negative regulation of TSH by T3 in cotransfection studies. These findings suggest that a novel, posttranscriptional mechanism caused central thyroid hormone resistance in this patient with a TSHoma. Similar posttranscriptional mechanisms may explain other hormone-resistant states or tumors in which no hormone receptor mutations can be detected from genomic DNA.
RESULTS
Clinical Studies
A 79-yr-old Caucasian female presented at NIH with goiter and palpitations due to hyperthyroidism. The patient had a serum TSH concentration of 10.5 μU/ml (normal, 0.43–4.60), free T4 concentration of 7.4 ng/dl (normal, 0.9–1.6), and glycoprotein hormone α-subunit concentration of 34.1 μg/liter (normal, <5). Serum GH and PRL levels were normal. A magnetic resonance image of pituitary revealed an 18-mm pituitary adenoma with extension into the left cavernous sinus. Transsphenoidal surgery was performed, and immunohistochemical analysis of the tumor showed strongly positive staining for TSH and glycoprotein hormoneα -subunit, as well as staining for both GH and PRL. Postoperatively, the patient’s serum TSH decreased to 3.1 μU/ml. However, the tumor could not be completely removed due to dural invasion, and the patient was started on octreotide therapy. The patient’s symptoms abated and her thyroid function tests normalized. A TRH stimulation test showed normal TSH secretory response (data not shown). A T3 suppression test performed 4 yr after surgery (Fig. 1) failed to suppress the patient’s serum TSH to less than 10% of the baseline, suggesting that at least part of the patient’s TSH secretion was due to residual tumor (1). Annual pituitary magnetic resonance imagings have shown a persistent 7-mm residual adenoma with no further growth. The patient’s clinical status has remained unchanged.
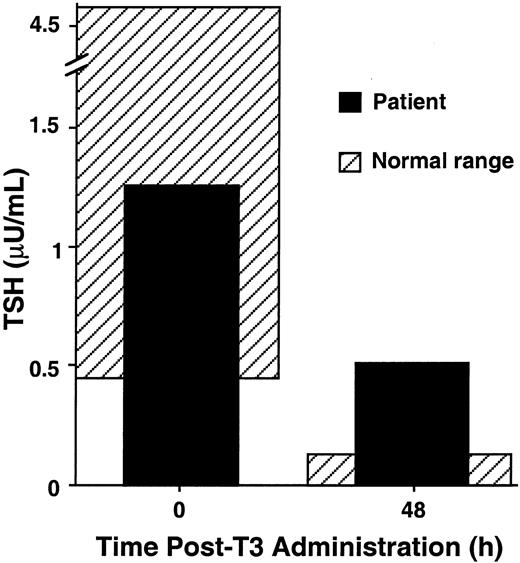
T3 Suppression Test of the Patient T3 (300 μg) was administered orally to the patient, and serum samples were obtained just before, and 48 h after, T3 administration for measurement of serum TSH. Normal basal serum TSH ranges from 0.43 to 4.60 μU/liter. Normal controls suppress to less than 10% of the baseline 48 h after T3 administration. Shaded areas represent normal ranges for baseline serum TSH and expected ranges for T3-suppressed serum TSH 48 h after T3 administration, respectively.
Finding of TRβ2 mRNA Splice Variant from a TSHoma
RNA from the patient’s TSHoma and pooled normal pituitaries were used to amplify full-length TRβ1, TRβ2, and an internal control, glyceraldehyde-3-phosphate dehydrogenase (GAPDH) mRNA by RT-PCR. Similar amounts of GAPDH mRNA were detected in the samples, suggesting that similar amounts of RNA were analyzed from the TSHoma and the pooled normal pituitaries (Fig. 2A, lanes 5 and 6). Additionally, similar amounts of TRβ1 mRNA were measured in the TSHoma and pooled normal pituitaries (Fig. 2A, lanes 1 and 2). Surprisingly, a short variant TRβ2 mRNA (TRβ2spl) was the major amplified product from the TSHoma, as only a small amount of amplified wild-type TRβ2 product was observed (Fig. 2A, lane 4). In contrast, only the wild-type TRβ2 product was amplified from the pooled normal pituitary RNA (Fig. 2A, lane 3). TRβ2spl mRNA was detected only in the TSHoma RNA when RT-PCR was performed using TRβ2 exon 6 primers (data not shown). Taken together, these findings suggested that TRβ2spl mRNA was specific for the TSHoma and contained a deletion within exon 6. Furthermore, since the primers used for amplification detected full-length TRβ2 cDNA, they enabled measurement of the relative expression of both wild-type TRβ2 and TRβ2spl mRNA within the same PCR reaction. These semiquantitative RT-PCR results showed that there is much higher expression of TRβ2spl mRNA than wild-type TRβ2 mRNA in the TSHoma, although the total amount of TRβ2 mRNA is not significantly different than TRβ2mRNA from the pooled normal pituitaries (Fig. 2B). Similar findings were observed in three repeat experiments. The intensities of the bands in Fig. 2A, lanes 3 and 4, corrected for GADPH mRNA expression, are shown in Fig. 2B.
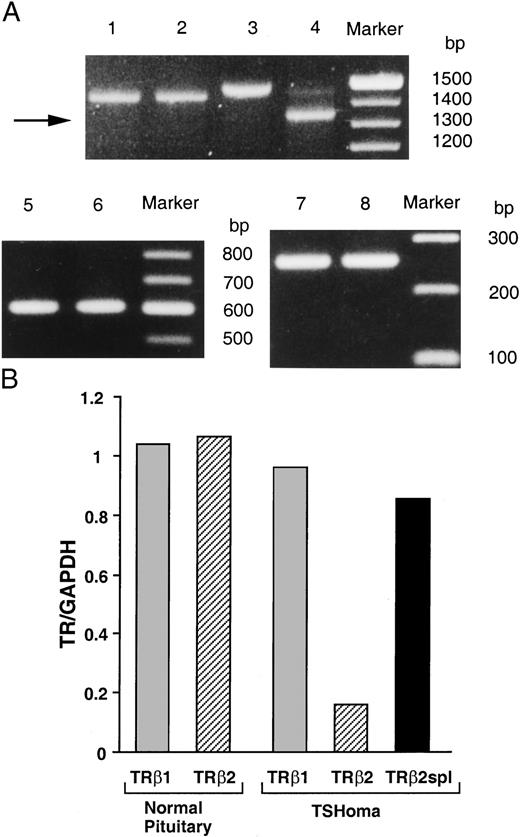
TRβ2spl Detection in a TSHoma A, RT-PCR was performed using mRNA from a TSHoma (lanes 2, 4, and 6) or pooled normal pituitary RNA from 87 individuals (lanes 1, 3, and 5). Primers were specific for the amplification of TRβ1 (lanes 1 and 2), TRβ2 (lanes 3 and 4), and GAPDH (lanes 5 and 6). Lanes 7 and 8 are products of exon 6 amplification of genomic DNA of TSHoma or circulating peripheral leukocytes of the same patient. B, Ratio of TR isoforms mRNA expression normalized with respect to GAPDH mRNA expression.
The RT-PCR products then were sequenced, and the TRβ2spl mRNA was found to have a 135 base in-frame deletion in exon 6 (Fig. 3). This deleted region was identical in TRβ1 and TRβ2 and encoded a part of the ligand-binding domain. Additionally, TRβ2spl protein had a newly created isoleucine in place of the deleted 46 amino acids in the ligand-binding domain (Fig. 3). PCR was performed using genomic DNA from the patient’s TSHoma and peripheral leukocytes and did not show any deletion in exon 6 (Fig. 2A, lanes 7 and 8).
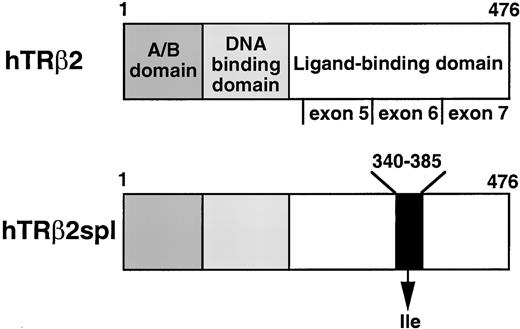
Structure of TRβ2 and Location of the Deletion of TRβ2spl Receptor domains and the three exons encoding the ligand-binding domain of TRβ2 are indicated. TRβ2spl has a deletion from amino acids 340 to 385 substituted by an isoleucine in the ligand-binding domain. Note that exons 5, 6, and 7 of TRβ2 are the same as exons 8, 9, and 10 of TRβ1; amino acids 340–385 of TRβ2 are the same as amino acids 325–340 of TRβ1.
Analysis of Splice Sites
Examination of both 5′- and 3′-sequences flanking the junction site of the deletion of TRβ2spl mRNA showed near-consensus splicing sequences that were located within exon 6 of wild-type TRβ2 (Fig. 4 A). These sequences conformed to several rules that are important for efficient splicing (17, 18). Most splice junctions are bordered by GT/AG at the 5′- and 3′-ends of the spliced sequences, as was the case for TRβ2spl mRNA. Additionally, there are consensus sequences for the 5′-splice site (ss), 3′-ss, and branch sequence, which are located 20 to 50 bases upstream of the 3′-ss. TRβ2 mRNA had near-consensus 5′- and 3′-splice sequences, as well as a consensus branch sequence within exon 6 (Fig. 4B). Using an internal coding exon predicting program, Michael Zhang’s Exon Finder (19), the 5′-ss and 3′-ss used by TRβ2spl had scores indicating their high potential for alternative splicing (AAAGTGAGA, 5′-ss score = 46.77; TGGATGACACTGAAGTA, 3′-ss score= 75.47), although their scores were slightly lower than those of the wild-type TRβ ss (CAGGTGAGT, 5′-ss score = 49.93; ACTGGTTCTTTTCAGCT, 3′-ss score =83.75).
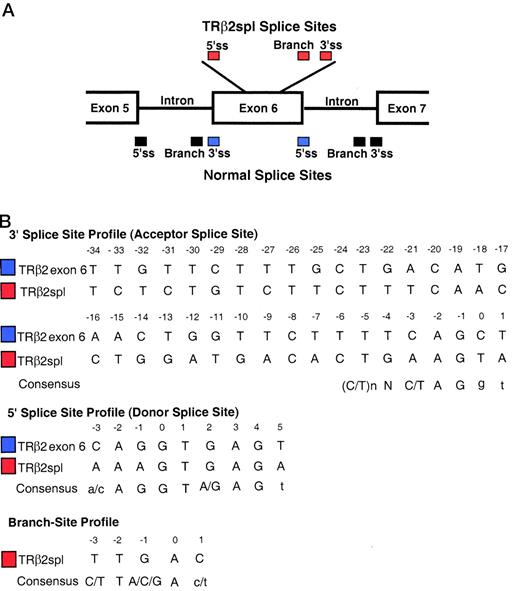
Analysis of Splice Sites A, Splice sites used for TRβ2spl (red) and normal splice sites (blue and black) are depicted. B, 3′-ss, 5′-ss, and branch site of TRβ2spl were compared with naturally occurring splice sequences flanking exon 6 of TRβ2, and consensus sequences. The branch site of the deletion of TRβ2spl mRNA is located 48 bases upstream of the 3′-ss of the deletion. Underlined nucleotides are absolutely required for splicing. Capitalized nucleotides of the consensus splice sequences are important, whereas lower case nucleotides are less important. Note that TRβ2 exon 6 is the same as TRβ1 exon 9.
Aberrant alternative splicing theoretically could be due to somatic mutations in the genomic DNA of the TSHoma, which, in turn, might generate a new cryptic splice site or create an unfavorable splice sequence in the intron/exon junctions of exon 6. However, sequencing of the PCR products from genomic DNA of the TSHoma showed no mutation in exon 6 and or in the flanking intronic sequences (data not shown). These findings demonstrate that TRβ2spl was created by aberrant splicing via latent splice sites that existed within exon 6 of wild-type TRβ2 mRNA.
Functional Properties of TRβ2 Splice Variant
Most mutations identified in patients with RTH are located in three clusters of the ligand-binding domain of TRβ (cluster 1: TRβ1 234–282, cluster 2: TRβ1 310–383, cluster 3: TRβ1 429–460) (20). The deletion of TRβ2spl is located within cluster 2 of the ligand-binding domain of TRβ2. Since most RTH mutants reduced binding to T3, the T3-binding activity of TRβ2spl was measured and compared with the T3-binding activities of wild-type TRβ2 and TRβ2G360R (TRβ2Mf), a cluster 2 mutant from a patient with RTH previously shown to have minimal T3 binding (20A ). As expected from the location of the deletion in the ligand-binding domain, both TRβ2spl and TRβ2Mf showed minimal T3 binding (Fig. 5). We then examined the functional activity of the wild-type TRβ2 and TRβ2spl on the regulation of the genes encoding the two subunits of TSH: glycoprotein hormone α-subunit and TSHβ. It has been previously shown that wild-type TR can cause T3-independent activation and T3-dependent negative regulation of transcription for these two genes (6, 15). As shown in Fig. 6, TRβ2spl lost both T3-independent activation and T3-dependent negative regulation on glycoprotein hormone α-subunit and TSHβ genes. In the presence of T3, TRβ2spl also showed higher transcriptional activity of the glycoprotein hormone α-subunit and TSHβ genes than wild-type TRβ2, consistent with the clinical observation of nonsuppressible TSH in the face of elevated T3 observed in the patient (Fig. 1). Cotransfection of the wild-type TRβ2 with TRβ2spl, as well as TRβ2Mf, resulted in diminished T3-dependent negative regulation of both glycoprotein hormone α-subunit and TSHβ genes (Fig. 7). These results show that both TRβ2spl and TRβ2Mf have strong dominant negative activity against the wild-type TRβ2 on the transcription of glycoprotein hormoneα -subunit and TSHβ genes.
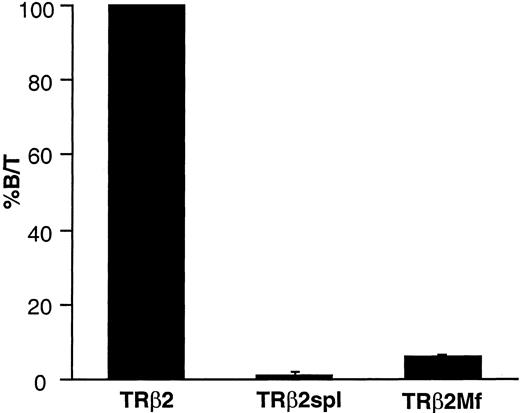
T3-Binding Activity of the Wild-Type TRβ2, TRβ2spl, and TRβ2Mf Wild-type TRβ2, TRβ2spl, or TRβ2Mf was transfected into CV-1 cells. Nuclear extracts were prepared and incubated with 125I-T3 as described in Materials and Methods. Bound and free 125I-T3 were separated, and the ratio between the bound and total T3 (B/T) was calculated. The specific T3 binding was obtained by subtracting the nonspecific binding determined in the incubation mixture containing a 10,000-fold excess of nonlabeled T3. The value of T3-binding activity of the wild-type TRβ2 is expressed as 100%. The data are represented as the mean ± sd of four samples.
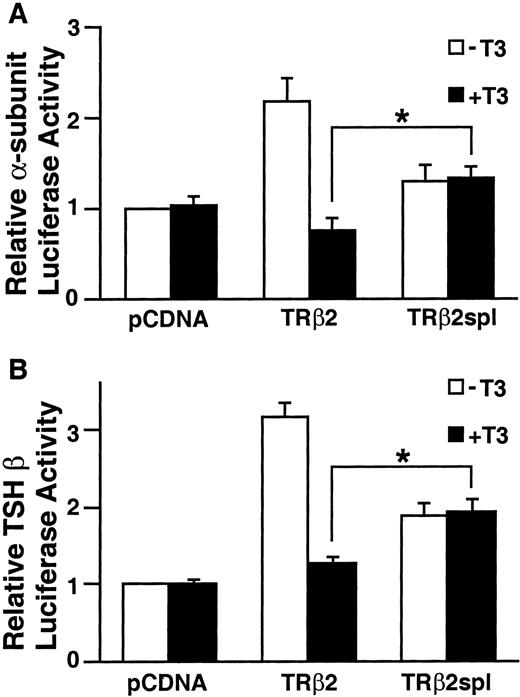
Transcriptional Activity of TRβ2 and TRβ2spl on Genes Encoding TSH Subunits Equal amounts of pcDNA expression vectors for TRβ2 and TRβ2spl were cotransfected with glycoprotein α-subunit or TSHβ luciferase reporter vectors into TSA 201 cells as described in Materials and Methods. The cells were incubated with 50 nm T3 for 40 h and harvested, and luciferase activity was measured. Data are represented as the mean ± sd from nine individual samples. The asterisk indicates significant difference between TRβ2spl-dependent and TRβ2-dependent luciferase activity in the presence of T3 (P < 0.01) determined by unpaired t tests. A, Glycoprotein hormone α-subunit luciferase activity. B, TSHβ luciferase activity.
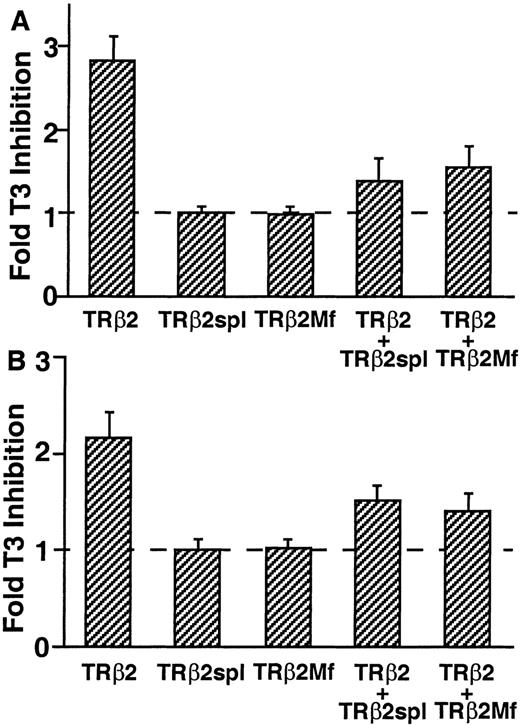
Dominant Negative Activity of TRβ2spl and TRβ2Mf on TRβ2 Regulation of Genes Encoding TSH Subunits TSA 201 cells were transfected with equal amounts of TRβ2 and TRβ2spl or TRβ2Mf with glycoprotein hormone α-subunit or TSHβ luciferase vector. The cells were incubated with 50 nm T3 and harvested, and luciferase activity was measured. Fold T3 inhibition is calculated as luciferase activity in the absence of T3 divided by luciferase activity in the presence of T3. Data are represented as the mean ± sd from nine individual samples. A, Glycoprotein hormoneα -subunit luciferase activity. B, TSHβ luciferase activity.
To study the interaction between TRβ2spl and transcriptional cofactors, the LexA yeast two-hybrid system was used. cDNAs of the ligand-binding domains of wild-type TRβ and TRβ2spl were subcloned into LexA expression vector, and coactivators, SRC-1 and transcription intermediary factor 2 (TIF2), and corepressors (NCoR and SMRT) were subcloned into B42AD expression vector. As expected, the ligand-binding domain of wild-type TRβ interacted with both SRC-1 and TIF2 in the presence of ligand and interacted with both NCoR and SMRT in the absence of ligand (Table 1). In contrast, the ligand-binding domain of TRβ2spl did not interact with any of these cofactors either in the presence or absence of ligand.
. | . | B42AD . | B42AD-SRC1 . | B42AD-TIF2 . | B42AD-SMRT . | B42AD-NCoR . |
---|---|---|---|---|---|---|
LexA | −T3 | − | − | − | − | − |
+T3 | − | − | − | − | − | |
LexA-TRβLBD | −T3 | − | − | − | + | + |
+T3 | − | + | + | − | − | |
LexA-TRβ2splLBD | −T3 | − | − | − | − | − |
+T3 | − | − | − | − | − |
. | . | B42AD . | B42AD-SRC1 . | B42AD-TIF2 . | B42AD-SMRT . | B42AD-NCoR . |
---|---|---|---|---|---|---|
LexA | −T3 | − | − | − | − | − |
+T3 | − | − | − | − | − | |
LexA-TRβLBD | −T3 | − | − | − | + | + |
+T3 | − | + | + | − | − | |
LexA-TRβ2splLBD | −T3 | − | − | − | − | − |
+T3 | − | − | − | − | − |
The expression plasmids producing either LexA DNA-binding-domain fusion proteins (listed at left) or pB42AD that encode B42 transactivation-domain fusion proteins (listed at top) were introduced into EGY48 cells, and the resulting transformants were grown on plates lacking leucine and containing X-Gal in the presence (+T3) or absence (−T3) of T3 (1μ m). Two-hybrid plasmids that resulted in production of blue colored colonies are indicated as (+).
. | . | B42AD . | B42AD-SRC1 . | B42AD-TIF2 . | B42AD-SMRT . | B42AD-NCoR . |
---|---|---|---|---|---|---|
LexA | −T3 | − | − | − | − | − |
+T3 | − | − | − | − | − | |
LexA-TRβLBD | −T3 | − | − | − | + | + |
+T3 | − | + | + | − | − | |
LexA-TRβ2splLBD | −T3 | − | − | − | − | − |
+T3 | − | − | − | − | − |
. | . | B42AD . | B42AD-SRC1 . | B42AD-TIF2 . | B42AD-SMRT . | B42AD-NCoR . |
---|---|---|---|---|---|---|
LexA | −T3 | − | − | − | − | − |
+T3 | − | − | − | − | − | |
LexA-TRβLBD | −T3 | − | − | − | + | + |
+T3 | − | + | + | − | − | |
LexA-TRβ2splLBD | −T3 | − | − | − | − | − |
+T3 | − | − | − | − | − |
The expression plasmids producing either LexA DNA-binding-domain fusion proteins (listed at left) or pB42AD that encode B42 transactivation-domain fusion proteins (listed at top) were introduced into EGY48 cells, and the resulting transformants were grown on plates lacking leucine and containing X-Gal in the presence (+T3) or absence (−T3) of T3 (1μ m). Two-hybrid plasmids that resulted in production of blue colored colonies are indicated as (+).
DISCUSSION
We have found a novel alternatively spliced variant of thyroid hormone receptor, TRβ2spl, from a TSHoma. This is also the first example of abnormal TR in TSHomas. TRβ2spl lacked T3-binding activity and was unable to mediate T3-dependent negative regulation of glycoproteinα -subunit and TSHβ genes. Furthermore, TRβ2spl showed dominant negative activity, as it blocked T3-dependent regulation of both these genes by wild-type TRβ2. These findings strongly implicate TRβ2spl in the defective negative regulation of TSH secretion exhibited by the tumor. This dysregulation in TSH secretion is similar to that observed in patients with RTH who have germline mutations in one of their TRβ alleles (12). Additionally, transgenic mice that overexpress dominant negative mutant TRβs in the pituitary have similar defects in the negative regulation of TSH (21–23). In this connection, we recently examined TRβ in five other TSHomas. Although we did not find another example of aberrant alternative splicing of TRs, we identified a somatic mutation in the ligand-binding domain of TRβ1 in one TSHoma (24). Gittoes et al. (25) recently reported decreased expression of TRα and TRβ in two TSHomas; therefore, down-regulation of TRs may be an additional mechanism for defective negative regulation of TSH by thyroid hormone. Furthermore, unlike GH-secreting adenomas, which can harbor G proteinα chain (Gsα) mutations that inhibit GTPase activity (26), no such mutations or TRH receptor mutations have been implicated in the constitutive expression of TSH in TSHomas (27). Taken together with our study, these findings suggest that defective negative regulation by the TR signaling pathway, rather than overactivity of the TRH signaling pathway, may be operative in some TSHomas.
TRβ2spl had a 46-amino acid deletion replaced by a newly created isoleucine, which maintained the original sequence of the remaining amino acids. The deletion is located in a region of the TRβ ligand-binding domain where a cluster of mutations has been reported for RTH patients (12). This region also is known to contribute to the formation of helices 7, 8, and 9 of TRβ, which compose part of the ligand-binding pocket of TR (28). Thus, the lack of T3 binding and transcriptional activity, as well as the dominant negative activity observed for TRβ2spl, are fully consistent with a deletion in this important region of the TRβ2 ligand-binding domain. The inability of the ligand-binding domain of TRβ2spl to interact with corepressors and coactivators in the yeast two-hybrid system is consistent with the loss of both T3-independent activation and T3-dependent negative regulation on glycoprotein hormone α-subunit and TSHβ genes observed in our cotransfection studies. Notably, helices 3, 4, 5, and 6 of TRβ and helices 3, 5, 6, and 12 of TRβ have been reported to contact surfaces with corepressors and coactivators, respectively (29–31). Although the deletion of TRβ2spl is not within these areas, tertiary structure changes, presumably due to the large conformational changes caused by the deletion, are likely responsible for the loss of interaction with corepressors. Tertiary structure changes also likely affected T3-binding activity and/or coactivator interactions of TRβ2spl.
In our study, only a small amount of wild-type TRβ2 mRNA was detected in the TSHoma, suggesting that TRβ2spl mRNA may have been spliced after wild-type TRβ2 mRNA was generated. Indeed, the 5′-ss score and 3′-ss score of the TRβ2spl ss were weaker than those of the naturally occurring ss flanking exon 6 of TRβ2. It is interesting that the variant form of TRβ1 mRNA was not observed in the TSHoma, particularly since TRβ1 and TRβ2 have identical sequences coding for the ligand-binding domain. It is possible that TRβ1 and TRβ2 mRNA have different higher order structures or differences in the turnover and/or export rates from the splicesome to account for this difference in splicing of the RNA between the two TRβ isoforms. Moreover, although TRβ2 and TRα1 have high homology in the mRNA sequences encoding their respective ligand-binding domains, it is unlikely that TRα1 has a corresponding splice variant, since TRα1 has GC/AG instead of GT/AG in the critical sequences that flank the splice junction of TRβ2spl. TRα mRNA can undergo alternative splicing to generate mRNA for a non-T3 binding protein, c-erbAα-2 (3). However, the location of the ss of TRβ2spl mRNA is more upstream than the 5′-ss of c-erbAα-2 mRNA; therefore, the generation of TRβ2spl mRNA is distinct from this naturally occurring process.
There are several potential mechanisms by which abnormal alternative splicing could generate TRβ2spl mRNA (32, 33). One possibility is the creation of a new cryptic splice site by a mutation(s) in the genomic DNA. However, this was not observed, as genomic DNA from the patient’s TSHoma did not have any mutations in the sequences flanking the splice junction or within the spliced sequence. Another possibility is that a mutation(s) in the natural ss of the flanking introns might create a less favorable splicing site, and thus allow splicing via the intraexonic ss. However, the intraexonic splicing pattern, which maintains portions of exon 6, and the absence of mutations in the naturally occurring ss contained in the intronic sequences immediately flanking exon 6, argue against this possibility. Thus, the most likely explanation for the aberrant splicing is increased activity of splicing factors in the TSHoma. The basis for this phenomenon could be a mutation in a splicing factor(s), or increased splicing activity as a consequence of overexpression or altered function (perhaps by phosphorylation or other regulatory events) of splicing factor(s) (34). Of note, aberrant alternative splicing and overactivity of splicing factors has been recently reported in breast cancer (35–38). Additionally, aberrant splicing has been reported for ER mRNA in breast cancer (39, 40). Given the aberrant splicing of TRβ2 mRNA in the TSHoma, it is possible that other mRNAs may be aberrantly spliced in the tumor and perhaps contribute to the growth of the tumor. Recently, an incidental pituitary adenoma in a patient with RTH was reported (41). In addition, there is one case report of pituitary enlargement in a patient with RTH in whom pituitary regression occurred after thyroid hormone treatment (42). However, TRβ knockout mice and transgenic mice overexpressing mutant TRβ showed defective TSH regulation but no increase in tendency to form TSHomas (11, 21, 22, 43). Additionally, there have been no reported cases of TSHomas in RTH patients who have TRβ mutations (12, 14), and patients with RTH do not seem to have a higher risk for TSHomas. Mutant TRβs per se may not be sufficient for the abnormal growth characteristics of TSHomas. Hence, the defective TRβ splicing could be a consequence rather than a cause of tumorigenesis.
Several families with RTH have been described who do not have mutations in their TRα and TRβ genes (44). Although knockout mice that lack a coactivator have mild central thyroid hormone resistance (45), no such postreceptor defects have been detected in these patients. Thus, it is possible that aberrantly spliced TRs may account for thyroid hormone resistance in some of these families. Hormone resistance in humans has been described for estrogen, glucocorticoid, androgen, and vitamin D, as well as peptide hormones, such as insulin, vasopressin, and PTH (46–52). In many cases, mutations in the nuclear hormone receptors or peptide hormone receptors were detected in genomic DNA and accounted for the clinical picture of hormone resistance. However, in rare instances in which no receptor mutation was found, receptor down-regulation or postreceptor defects have been described or hypothesized (44, 51, 53, 54). Our findings suggest that aberrant alternative splicing is another mechanism for hormone resistance in signaling pathways in which no receptor mutation is found in the genomic DNA.
In conclusion, we describe a splicing variant of TR from a TSH-secreting pituitary tumor that caused defective T3-dependent negative regulation of glycoprotein hormone α-subunit and TSHβ genes in a transient transfection system. Since the TRβ gene did not contain any mutations that accounted for the deletion, this abnormal TR was caused by aberrant alternative splicing of TRβ2 mRNA. This example enlarges our understanding of the possible mechanisms for hormone resistance and suggests that posttranscriptional causes need to be considered whenever genomic mutations are not detected in hormone receptors.
MATERIALS AND METHODS
Analyses of RNA and Genomic DNA
Genomic DNA was isolated from the patient’s TSHoma and peripheral blood leukocytes. Total RNA was extracted from the TSHoma by Trizol reagent (Life Technologies, Inc., Gaithersburg, MD). Pituitary gland polyA RNA (pool of 87 normal tissue specimens) was purchased from CLONTECH Laboratories, Inc. (Palo Alto, CA). cDNA was synthesized by Moloney murine leukemia virus-reverse transcriptase. DNA was amplified by PCR with the following specific oligonucleotide primers: TRβ1 sense primer: 5′-GGATCCAGAATGATTACTAACCTATGACTC-3′; TRβ2 sense primer: 5′-ACCAGGGAAACAAAATGAACTACTGTATGC-3′; TRβ1 and TRβ2 common antisense primer: 5′-GGAATTATAGGAAGGAATCCAGTCAGTCTA-3′; TRβ2 exon 6 sense primer (corresponding to exon 9 of TRβ1): 5′-CTGCCATGTGAAGACCAGATCATCCT-3′; TRβ2 exon 6 antisense primer: 5′-CTGAAGACATCAGCAGGACGGCCTGA-3′; Sense primer sequence for TRβ2 exon 6 and flanking introns: 5′-TCACAGAAGGTTATTCCTATT-3′; antisense primer sequence for TRβ2 exon 6 and flanking introns: 5′-ACTCAAGTGATTGGAATTAG-3′; GAPDH sense primer: 5′-CATCACGCCACAGTTTCCCGGAGG-3′; GAPDH antisense primer: 5′-TTTCTAGACGGCAGGTCAGGTCCACC-3′. For semiquantitative RT-PCR, PCR conditions for temperature were optimized for each primer pair, and comparative kinetic analyses were performed to determine that the selected PCR cycle number for each primer pair was within the exponential phase of product generation. PCR products were visualized on 1% Tris-borate-EDTA agarose gels stained with ethidium bromide. Ethidium bromide-stained gels were digitized, and densitometry was performed on the product bands using Image Quant (Molecular Dynamics, Inc., Sunnyvale, CA) (55, 56). Quantification of RT-PCR by analysis of ethidium-stained gels yielded consistent results. PCR products were subcloned into pCR4TOPO plasmid (Invitrogen, Carlsbad, CA), and then sequenced.
ss Profile Analysis
ss Profiles were analyzed by Michael Zhang’s Exon Finder, a software program designed to predict ss sequences (19).
Construction of TR cDNA Expression Vectors
The full-length human wild-type TRβ2 cDNA was cloned into pcDNA. The DNA fragment carrying the deletion mutation identified in the patient’s TSHoma was digested with Tth111I and BglII and then subcloned into the corresponding TRβ2 region. A natural mutant TRβ1G345R from a patient with RTH was also digested with Tth111I and BglII and replaced with the corresponding TRβ2 region to create TRβ2G360R (TRβ2Mf). The final constructs were verified by sequencing.
T3 Binding Assay
T3 binding affinity of wild-type TRβ2 and TRβ2spl protein was measured in transfected CV-1 cells as described previously (57). CV-1 cells were transiently transfected with wild-type TRβ2, TRβ2spl, or TRβ2Mf using Lipofectamine Plus (Life Technologies, Inc.). Cells were homogenized in 0.25 m sucrose and 3 mm MgCl2 (SM). The crude nuclear pellet was suspended once with and once without 0.5% Triton X-100 in SM. Nuclear proteins were extracted by stirring isolated nuclei at 4 C for 1 h in 0.4 m KCl, 5 mm MgCl2, 1 mm dithiothreitol, and 0.05 m Tris-glycine HCl (pH 8.5). After nuclear extracts were centrifuged at 117,000× g for 45 min, T3-binding activities in the supernatants were assayed. Nuclear proteins (125 μg) were incubated with 1 nm125I-T3 (NEN Life Science Products, Boston, MA) and 4 nm non-labeled T3 at 4 C overnight. 125I-T3 was separated from the bound form using AG1-X8 resin (Bio-Rad Laboratories, Inc., Richmond, CA). Nonspecific binding was determined in the incubation mixture containing a 10,000-fold amount of nonlabeled T3.
Cotransfection Studies of TR
TSA 201 cells (a strain of HEK293 cells transformed with T antigen) were maintained in Opti-MEM (Life Technologies, Inc.) containing 4% FCS pretreated with AG1-X8 resin to remove endogenous thyroid hormones. The cells were plated in six-well dishes and transfected 24 h later by the calcium phosphate precipitation method with 250 ng of TR expression vector and 500 ng of human glycoprotein hormone α-subunit promoter (−846 to +44) linked to pA3 luciferase reporter gene (6). Additionally, TSA201 cells were transfected with 400 ng of TR expression vector, 200 ng of human TSHβ promoter (−1,192 to +37) gene linked to pA3 luciferase reporter gene (15), and 200 ng of human thyrotroph embryonic factor expression vector (obtained by RT-PCR) with Lipofectamine Plus. Eight hours after transfection, the cells were washed and incubated for 40 h with Opti-MEM media containing 1% of resin-treated FCS with and without 50 nm T3. The cells were lysed and assayed for luciferase activity. Luciferase activity was normalized according to protein concentration.
Yeast Two-Hybrid System
EGY48 yeast cells, p8op-lacZ, and LexA-parental vectors (pGilda) and B42-parental vectors (pB42AD) were purchased from CLONTECH Laboratories, Inc. Fragments of human wild-type TRβ2 and TRβ2spl ligand-binding domain (amino acids 189–476) were subcloned into EcoRI and SalI sites of pGilda to make LexA-TRβLBD and LexATRb2spl LBD. Fragments of human F-SRC-1 (1–1440), human TIF2 (1–1464), human NCoR (982–1495), and human SMRT (982–1495) were subcloned into pB42AD to make B42AD-SRC-1, B42AD-TIF2, B42AD-NCoR, and B42AD-SMRT. LexA fusion vectors, B42AD fusion vectors, and p8op-lacZ were transformed into EGY48 according to the manufacturer’s manual. Interactions of fusion proteins were tested using selection for leucine auxotrophy and Lac Z reporter gene on plates in the presence and absence of 1 μm T3.
Acknowledgments
We thank Dr. Frederic Wondisford (University of Chicago, Chicago, IL) for human TRβ2 expression and TSHβ reporter vectors, Dr. Laird Madison, Dr. Larry Jameson, Mr. Kevin Long (Northwestern University, Chicago, IL) for glycoprotein hormone α-subunit reporter vector and TSA 201 cells, Dr. Ronald Evans (Salk Institute, La Jolla, CA) for human SMRT expression vector, Dr. Anthony Hollenberg (Beth Israel Hospital, Boston, MA) for human NCoR (NCoRi) expression vector, and Dr. Pierre Chambon (INSERM, Strasbourg, France) for TIF2 expression vector. We also thank Drs. Thomas Misteli and Carl Baker (National Cancer Institute, Bethesda, MD) for helpful discussions on splicing mechanisms.
This work was supported in part by NIH Grants HG-01696 (to M.Q.Z.) and DK-15070 (to S.R.).
Abbreviations:
- GAPDH,
Glyceraldehyde-3-phosphate dehydrogenase;
- NCoR,
nuclear receptor corepressor;
- RTH,
resistance to thyroid hormone;
- SMRT,
silencing mediator of retinoic acid and thyroid hormone receptor;
- SRC-1,
steroid receptor coactivator-1;
- ss,
splice site;
- TIF2,
transcription intermediary factor 2.