-
PDF
- Split View
-
Views
-
Cite
Cite
Michael W Guernsey, Henri van Kruistum, David N Reznick, Bart J A Pollux, Julie C Baker, Molecular Signatures of Placentation and Secretion Uncovered in Poeciliopsis Maternal Follicles, Molecular Biology and Evolution, Volume 37, Issue 9, September 2020, Pages 2679–2690, https://doi.org/10.1093/molbev/msaa121
- Share Icon Share
Abstract
Placentation evolved many times independently in vertebrates. Although the core functions of all placentas are similar, we know less about how this similarity extends to the molecular level. Here, we study Poeciliopsis, a unique genus of live-bearing fish that have independently evolved complex placental structures at least three times. The maternal follicle is a key component of these structures. It envelops yolk-rich eggs and is morphologically simple in lecithotrophic species but has elaborate villous structures in matrotrophic species. Through sequencing, the follicle transcriptome of a matrotrophic, Poeciliopsis retropinna, and lecithotrophic, P. turrubarensis, species we found genes known to be critical for placenta function expressed in both species despite their difference in complexity. Additionally, when we compare the transcriptome of different river populations of P. retropinna, known to vary in maternal provisioning, we find differential expression of secretory genes expressed specifically in the top layer of villi cells in the maternal follicle. This provides some of the first evidence that the placental structures of Poeciliopsis function using a secretory mechanism rather than direct contact with maternal circulation. Finally, when we look at the expression of placenta proteins at the maternal–fetal interface of a larger sampling of Poeciliopsis species, we find expression of key maternal and fetal placenta proteins in their cognate tissue types of all species, but follicle expression of prolactin is restricted to only matrotrophic species. Taken together, we suggest that all Poeciliopsis follicles are poised for placenta function but require expression of key genes to form secretory villi.
Introduction
The placenta is a complex extra embryonic organ that facilitates offspring growth and development in some live-bearing animals. This organ has been most thoroughly studied in mammals, where it displays a considerable amount of morphological diversity between species (Enders and Carter 2004). The placentas of mice and humans are incredibly complex organs composed of many different cell and tissue types that actively invade maternal tissues to establish direct contact between the fetal and maternal bloodstreams (Leiser and Kaufmann 2009). By contrast, the placentas of marsupials, like the tammar wallaby, are quite simple being composed of a few cell layers without active invasion into the uterus (Freyer et al. 2002; Freyer and Renfree 2009). Despite this diversity, the placentas of mammals share a single evolutionary origin. This is not the only origin of placentation, however, as many different vertebrate lineages including, squamate reptiles (Van Dyke et al. 2014), cartilaginous fishes (Haines et al. 2006), and teleost fishes (Pollux et al. 2009) have also evolved placentas. These placentas vary greatly in both complexity and morphology. Some lizard species have evolved chorioallantoic placentas that closely resemble the complex eutherian placenta (Blackburn et al. 1984). Atlantic sharpnose sharks have evolved to modify the vessels of its yolk sac to form an umbilical cord to facilitate maternal provisioning (Hamlett 1993). Sygnathid fishes are the only group to exhibit male pregnancy, where the brood pouch differentiates to become placenta-like by thickening, increasing vasculature, and forming epithelial microridges (Laksanawimol et al. 2006; Stölting and Wilson 2007). Despite this diversity of form, these placentas must coordinate a common set of functions including nutrient provisioning, gas exchange, waste removal, and maintenance of pregnancy (Cross 2006). Given these striking examples of placentation, it raises the question of whether independently evolved vertebrate placentas are molecularly similar to that of the mammal, which would suggest the same processes were co-opted in multiple distinct species to evolve a placenta.
The fish family Poeciliidae represents one of the most remarkable examples of convergent placenta evolution. Nearly, all species in this family give live birth, but some species are matrotrophic, meaning the mother actively provides nutrition for its offspring throughout pregnancy (Thibault and Schultz 1978). Other species are lecithotrophic, meaning the mother packs the eggs with enough yolk to sustain its offspring throughout pregnancy without active maternal provisioning (Thibault and Schultz 1978). These strategies have been quantified using the matrotrophic index (MI), a measure that assesses how much weight an offspring gains from fertilization to birth. Simply, the offspring of a matrotrophic species will gain weight and have an MI > 1, indicating that the mother provided a lot of nutrition throughout pregnancy (Wourms et al. 1988; Reznick et al. 2002). Conversely, the offspring of a lecithotrophic species loses weight and corresponds to an MI < 1, indicating that the mother provided the required nutrition before fertilization (Wourms et al. 1988; Reznick et al. 2002). The reproductive strategy of matrotrophy implies the presence of a structure that can provide for an offspring throughout pregnancy, this is the placenta. The placental structures of Poeciliid fish were first described as a “follicular pseudoplacenta,” because the villi observed closely resembled the intimate villous connections in the mammalian placenta (Turner 1940). These structures were further characterized by electron microscopy using the matrotrophic species Heterandria formosa as a model (Grove and Wourms 1991, 1994). The villi previously described were a portion of the maternal follicle, the maternal component of the Poeciliid placenta. In matrotrophic species, the inner epithelium of this structure folds in on itself to form elaborate villi that directly interface with the embryo (Grove and Wourms 1994). In turn, the embryonic portion of the Poeciliid placenta was observed as a dense coating of microvilli covering the embryonic surface that becomes restricted to the pericardial sac as development proceeds (Grove and Wourms 1991). The interaction between these structures is thought to allow placental exchange in a manner similar to the interactions of the maternal decidua and fetal trophoblast in mammalian placentation.
The genus Poeciliopsis is unique among Poeciliid fish because complex placental structures have independently evolved at least three times in this lineage (Reznick et al. 2002). This means that complex placental structures (Kwan et al. 2015; Panhuis et al. 2017) have evolved on the order of tens to hundreds of thousands of years (Reznick et al. 2002), rather than the tens of millions of years since the mammalian placenta arose and diversified. Matrotrophic Poeciliopsis species have lecithotrophic sister taxa in which placental structures are rudimentary (Reznick et al. 2002), making this an ideal system to study how a placenta emerges and diversifies. Recent microscopy studies of the Poeciliopsis placenta highlight the utility of this system (Kwan et al. 2015; Panhuis et al. 2017). The Poeciliopsis maternal follicle shares some key features with the previously studied H. formosa (Grove and Wourms 1994), namely the presence of villous structures on the inner epithelial surface to increase surface area for exchange (Kwan et al. 2015). However, this varies greatly between the different origins of placentation from robust fingerlike villi in Poeciliopsis retropinna, to shorter more stub-like villi in P. turneri, to small waves on the inner epithelial layer in P. prolifica (Kwan et al. 2015). This variation is highlighted further when observing the embryonic component of the Poeciliopsis placenta. Although P. prolifica exhibits microvilli at the embryonic surface like H. formosa (Grove and Wourms 1991), both P. retropinna and P. turneri have no such microvilli at the embryonic surface and look instead more like their lecithotrophic counterparts (Panhuis et al. 2017). Further research needs to be completed to understand the fetal contribution to the placenta in these species. However, the diversity in placenta form and the closely related matrotrophic and lecithotrophic species makes Poeciliopsis an ideal system to investigate the molecular basis of placenta evolution.
To better understand the transition from lecithotrophy to matrotrophy in live birth, we chose to study the maternal follicle of P. turrubarensis and P. retropinna. We find that the morphology of the maternal follicles of these two species differs greatly (fig. 1), which led us to sequence and analyze their transcriptomes. We find that both species express many genes that are critical for mammalian placenta biology, despite their difference in reproduction. We also propose that different populations of P. retropinna are able to rapidly shift MI by changing the expression of key secretory genes, hinting at a key mode of Poeciliopsis placenta function. Additionally, we find that highly conserved mammalian placenta proteins used to distinguish the maternal decidua from the fetal trophoblast also distinguish the maternal and fetal components of the Poeciliopsis placenta. Moreover, we find that prolactin is a strong candidate for a signal that mediates the transition to matrotrophy. Taken together, our data add much needed molecular and developmental components to our understanding of placenta evolution in Poeciliopsis.
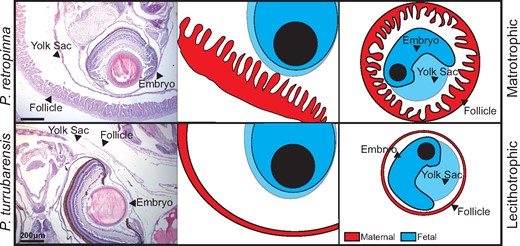
Maternal–fetal interface in Poeciliopsis. Schematic comparing the maternal–fetal interface of a matrotrophic (Poeciliopsis retropinna, top) and lecithotrophic (P. turrubarensis, bottom) species. Left panels are H&E stains showing the maternal follicle, yolk sac, and embryo. Scale bars represent 200 μm. Middle panels are a graphical representation of the H&E with maternal tissues depicted in red and fetal tissues depicted in blue. Right panels represent a zoom out to depict the entire embryo and maternal follicle for context.
Results
Maternal Follicle Morphology and Transcriptomes Reveal Key Differences and Similarities in Placenta Evolution
Variation in the MI corresponds to dramatic differences in maternal follicle morphology (Kwan et al. 2015). To confirm these differences, we collected pregnant ovaries, containing many embryos where the placenta would be active, from three matrotrophic (P. retropinna, P. prolifica, and P. turneri) and three lecithotrophic (P. turrubarensis, P. gracilis, and P. infans) Poeciliopsis species. We mounted the ovaries in paraffin blocks, took sections, and performed hematoxylin and eosin (H&E) staining. When we compared the same developmental stage (Haynes 1995) between matrotrophic and lecithotrophic species, we observed differences in the maternal follicles consistent with previous work, including the presence of villi in matrotrophic species and apparent differences in follicle thickness between matrotrophic and lecithotrophic species (Kwan et al. 2015) (fig. 2). The maternal follicle of matrotrophic species is composed of two major layers: the first being a thick mesenchymal layer on the outside of the follicle and the second a dense layer a villi facing the embryo (fig. 2). The follicles of lecithotrophic species are comparatively simple, being composed of a single cell layer with no obvious villi facing the embryo (fig. 2).
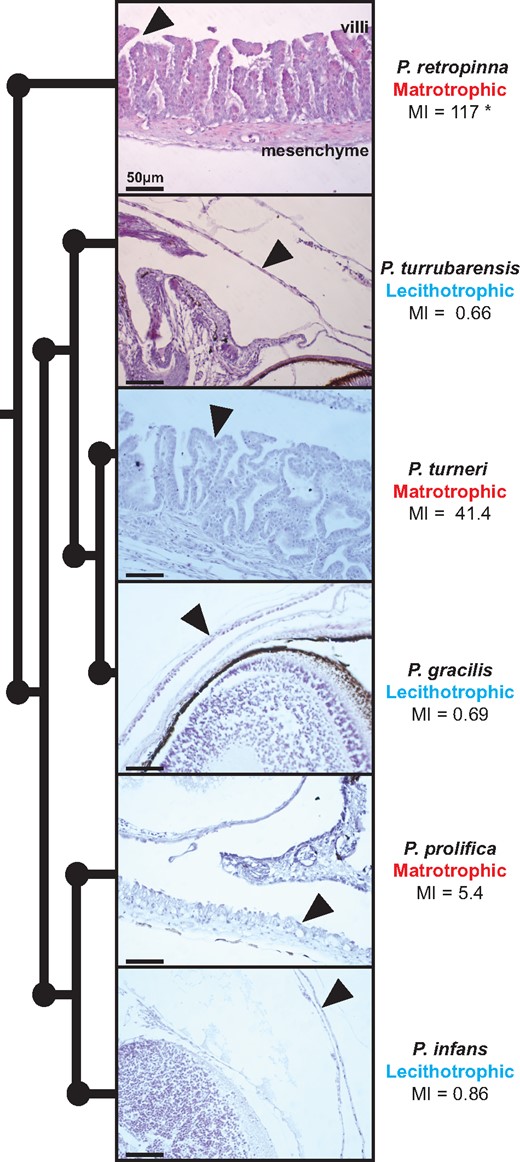
Maternal follicle exhibits great morphological diversity within Poeciliopsis. Phylogeny depicting the relationship of six Poeciliopsis species with a corresponding H&E stain for late-stage embryos with arrows indicating the position of the maternal follicle. The MI for each species was obtained from Reznick et al. (2002). *The MI of this species is variable (Hagmayer et al. 2018). Scale bars represent 50 μm.
To identify genes that might be critical to the transition from lecithotrophic to matrotrophic live birth, we focused on two species we were able to collect in the wild, P. retropinna and P. turrubarensis. We sequenced the late-stage (Haynes 1995) maternal follicle transcriptome of each, averaged the counts for P. retropinna (12 replicates) and P. turrubarensis (3 replicates), and compared the transcripts expressed. The P. retropinna follicle expresses 7,784 genes, whereas P. turrubarensis expresses 7,683 genes. Expression of 6,144 (fig. 3, red points) of these genes is shared between P. retropinna and P. turrubarensis, whereas 1,640 (fig. 3, blue points) and 1,539 (fig. 3, yellow points) genes are expressed uniquely in each species. To more rigorously compare the genes with shared expression, we performed differential expression analysis using DEseq2. We identified 357 genes (fig. 3, purple points) more highly expressed in P. retropinna and 1,016 genes (fig. 3, orange points) more highly expressed in P. turrubarensis. The genes uniquely and more highly expressed and in P. retropinna had ontology related to various biosynthetic processes, transport, and cellular localization (P-adj = 0.000344, 0.0401, and 0.00136) (supplementary file 1, Supplementary Material online). This suggests the P. retropinna follicle actively synthesizes compounds that it transports to its developing offspring. The genes uniquely and more highly expressed in P. turrubarensis had ontology related to blood biology and epithelial development (P-adj = 0.0111, 0.046) (supplementary file 2, Supplementary Material online). This is consistent with previous work indicating that the lecithotrophic follicle plays a key role in gas exchange (Jollie WP and Jollie LG 1964; Blackburn et al. 2010).
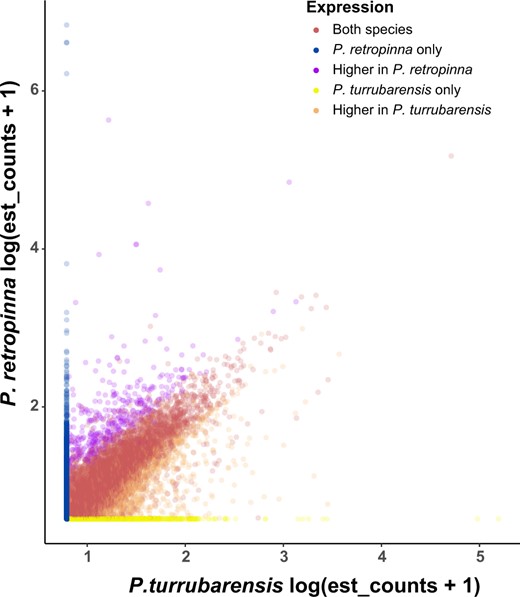
Placental genes expressed in matrotrophic and lecithotrophic Poeciliopsis species. Comparison of the genes expressed in the Poeciliopsis retropinna maternal follicle (y axis) and the P. turrubarensis maternal follicle (x axis) by log-transformed estimated counts. Blue points are genes expressed only in the P. retropinna maternal follicle (n = 1,640), purple points are genes with significantly higher expression in the P. retropinna maternal follicle (n = 357), yellow points are genes expressed only in the P. turrubarensis maternal follicle (n = 1,539), orange points are genes with significantly higher expression in the P. turrubarensis maternal follicle (n = 1,016), and red points are genes with similar expression in the maternal follicle of both species (n = 4,771).
The mammalian placenta expresses many key genes that are essential for the proper growth and development of offspring. Interestingly, we found expression of many of these genes including: Vim (Can et al. 1995), Cebpb (Lynch et al. 2011), Syna (Dupressoir et al. 2011), Bmp7 (Monsivais et al. 2017), Bmpr2 (Nagashima et al. 2013), Notch2 (Hamada et al. 2007), Ctsb (Song et al. 2007), Phlda2 (Tunster et al. 2016), Akt1 (Kent et al. 2012), E2f8 (Qi et al. 2015), Itgav (Frank et al. 2017), Snai1 (Butler et al. 2017), Flt1 (Ji et al. 2018), Tmed2 (Jerome-Majewska et al. 2010), and Dnajb6 (Watson et al. 2007) in the transcriptome of both P. retropinna and P. turrubarensis. We also find expression of Igf2, an imprinted gene in the mammalian placenta (Reik et al. 2003; Stringer et al. 2012) with critical roles in the growth of offspring that has been previously identified in the placenta of P. prolifica (Lawton et al. 2005). To more quantitatively assess the similarity of our follicle transcriptomes to the mammalian placenta, we compared our data with the core placenta transcriptome. These are 115 nonhousekeeping genes expressed in the placenta of all eutherian mammals (Armstrong et al. 2017). We uncovered the expression of 87/115 of these genes in both of our Poeciliopsis transcriptomes, which is on the same order as the marsupial placenta that expresses 95/115 genes (Armstrong et al. 2017), despite being incredibly similar to the eutherian placenta (Guernsey et al. 2017). Overall, our sequencing unveils that the P. retropinna follicle has a unique biosynthesis role allowing it to consistently provide for developing offspring, whereas the P. turrubarensis follicle expresses more genes related to blood and epithelial development. Interestingly, both species express key transcripts that are essential for the proper growth and development of the mammalian placenta.
Secretory Gene Expression Varies between P. retropinna Populations
Previous work has demonstrated that maternal provisioning in P. retropinna varies based on river of origin (Hagmayer et al. 2018). We wondered if the dramatic morphological differences in Poeciliopsis might be reflected in these closely related populations. We compared the maternal follicle histology, using H&E staining, from two previously studied populations (Hagmayer et al. 2018) and two additional populations, but find no obvious morphological differences (supplementary fig. 1, Supplementary Material online). This led us to believe that these differences must arise from changes at the molecular level. To determine what these changes are, we performed additional analyses on the maternal follicle transcriptome of P. retropinna which includes replicates from Rio Ceibo (three replicates), Rio Potrero Grande (three replicates), Rio Sucio (three replicates), and Rio Seco (three replicates). We performed pairwise comparisons of differential expression in all four populations using DEseq2. We identified 101 genes that were differentially expressed in at least one of these comparisons (fig. 4A). Secretion is the only ontology significantly enriched (P-adj = 0.0493) (supplementary file 3, Supplementary Material online), related to 16 of these genes (fig. 4A). This result suggests that secretory differences drive differences in maternal provisioning and that Poeciliopsis placentas function via secretion rather than bathing the placenta in maternal blood.
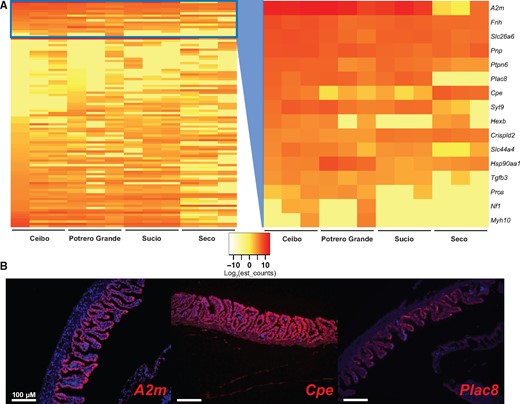
Secretory gene expression differs in different Poeciliopsis retropinna river populations. (A) Genes differentially expressed in the maternal follicle of P. retropinna collected from different river populations (n = 101). Sixteen of these genes, contained in the blue box and expanded in the second panel, have ontology related to secretion. Red indicates high expression and yellow indicates lower expression as measured by log-transformed estimated counts (see insert between heatmaps). (B) RNA-FISH of the secretion genes A2m, Cpe, and Plac8 in late-stage P. retropinna maternal follicles. Red indicates the expression of the mRNA and blue is a DAPI counterstain. Scale bars represent 100 μm.
To identify the location of secretory transcripts differentially expressed between rivers (fig. 4A), we performed RNA-FISH on A2m (Gu et al. 1992), Cpe (Singh et al. 2006), and Plac8 (Chang et al. 2018), which are highly expressed and have documented expression in the mammalian placenta. The expression of all three transcripts was the strongest at the surface of the follicle villi (fig. 4B), suggesting for the first time that these cells possess secretory function. Cpe was also weakly expressed in the follicle mesenchyme (fig. 4B). When we compared the expression of Plac8 and Cpe in all four river populations, we could not detect differences in Plac8 expression and could only detect that Cpe is most highly expressed in Rio Seco (supplementary fig. 2, Supplementary Material online). Although this does not perfectly match the RNA-seq data, we believe that the detection and location of secretion transcripts is still revealing. This may also be related to sample-to-sample variation, as we did not have multiple samples from each population to perform RNA-FISH. Overall, this analysis suggests that secretion from cells at the surface of villi is key for Poeciliopsis placenta function and that changes in secretory gene expression can rapidly alter maternal provisioning.
Convergent Placenta Protein Expression Underlies the Evolution of Live Birth
We next sought to expand our observation of molecular convergence in the placentas of mammals and Poeciliopsis. To accomplish this, we visualized the expression of mammalian placenta proteins by performing immunofluorescence on late-stage maternal follicles and embryos from the six Poeciliopsis species previously analyzed (fig. 2). We first tested the expression of Vimentin (VIM), an intermediate filament protein commonly expressed in mesenchymal cells that is strongly expressed in the maternal placenta (decidua) of eutherian mammals (Can et al. 1995). The mRNA of this gene is expressed in the transcriptome of both P. retropinna and P. turrubarensis. We observe VIM to be strongly expressed in the maternal follicle of all Poeciliopsis species assayed (fig. 5). In matrotrophic species, the expression is in both the villi and mesenchymal layers (fig. 5). Given that VIM is expressed in the maternal component of the placenta of both eutherian mammals and Poeciliopsis, we next tested whether that trend continues with fetal placenta (trophoblast) proteins. To this end, we tested the expression of Pan-Cytokeratin, an epithelial marker that is commonly used to distinguish fetal placenta tissues in a wide variety of eutherian species (Gauster et al. 2013). We find Pan-Cytokeratin is most strongly expressed in a thin layer along the surface of the embryo of all species assayed (fig. 5). The embryo surface is assumed to be the fetal component of the Poeciliopsis placenta, and microvilli have even been observed on the surface of developing P. prolifica embryos (Panhuis et al. 2017). The expression of these two proteins suggests that similar genes have been co-opted in the evolution of live birth in both fish and mammals but may also represent the ancestral condition, as these are broadly expressed mesenchymal and epithelial markers.
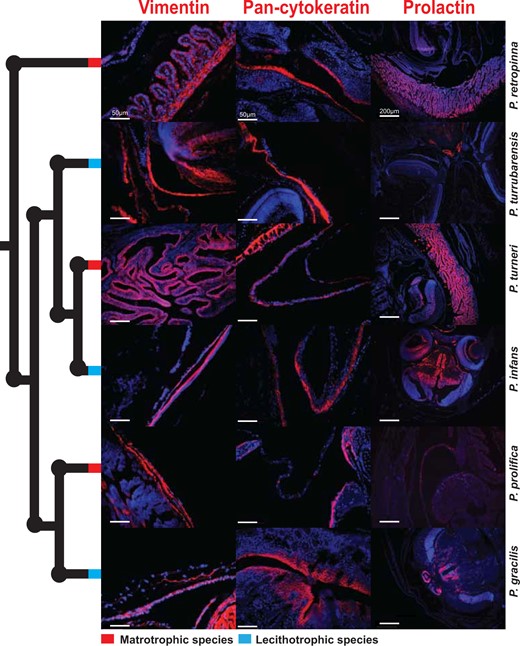
Mammalian placenta proteins expressed in cognate Poeciliopsis tissues. Immunofluorescence of mammalian placenta proteins: Vimentin, Pan-Cytokeratin, and prolactin in late-stage embryos and maternal follicles of three matrotrophic (red nodes) and three lecithotrophic (blue nodes) Poeciliopsis species. Red indicates the expression of the protein and blue is a DAPI counterstain. Scale bars represent 50 μm for the Vimentin and Pan-cytokeratin images and 200 μm for the prolactin images.
We finally sought to find a protein that varies in expression between matrotrophic and lecithotrophic Poeciliopsis species that would provide stronger evidence of convergence. From our analysis of P. retropinna river populations, we found that A2m was differentially expressed. Interestingly, A2m also had exceptionally high expression in P. retropinna (matrotrophic) follicles but was not expressed in P. turrubarensis (lecithotrophic) follicles. Since A2M is known to be regulated by prolactin(Gu et al. 1992), we hypothesized that prolactin expression itself might differentiate between matrotrophic and lecithotrophic species. Prolactin is a pituitary hormone with critical roles in the formation and maintenance of the decidua (Ben-Jonathan et al. 1996). Furthermore, expression of Prl has been co-opted by the endometrium of many different mammalian species independently by utilizing transposon-derived promoters (Emera et al. 2012). This suggests that its regulation is critical for evolutionary variation in reproductive structures. To this end, we examined the expression of prolactin in both matrotrophic and lecithotrophic species using immunofluorescence. We find prolactin to be expressed in the follicles of matrotrophic species but absent in the follicles of lecithotrophic species (fig. 5). We also find prolactin expression in the brain of all species suggesting the antibody works as expected (fig. 5). We do not find Prl expression in the transcriptome of either species we sequenced but do observe weak expression of Prlr, suggesting the source of prolactin protein is not the maternal follicle itself. These immunofluorescence assays unveil the expression of mammalian maternal and fetal placenta proteins in the cognate tissue types of both matrotrophic and lecithotrophic Poeciliopsis and identify prolactin as a key candidate that moderates the emergence of matrotrophy.
Discussion
Placental Genes in the Evolution of the Maternal Follicle
We find that mammalian placenta genes are shared between the maternal follicle of P. retropinna and P. turrubarensis, which is intriguing as one is structurally complex and the other relatively simple. We believe that this means that lecithotrophic Poeciliopsis species are poised to have complex placental structures but are missing a small number of key signals. As studying variation within a single species can be used to identify genes with large impacts on morphology (Steiner et al. 2007; Shapiro et al. 2013), we hoped our interpopulation comparisons of P. retropinna might shed light on what these missing signals might be. The identification of secretion transcripts in this analysis tells us that the amount of material secreted by the maternal follicle is key for altering MI. Plac8 is particularly intriguing as it is expressed only in the follicles of P. retropinna. Furthermore, Plac8 was initially identified in placental tissues (Galaviz-Hernandez et al. 2003) but has since been found to have key roles in regulating proliferation, differentiation, and apoptosis in a wide variety of tissues and organisms (Cabreira-Cagliari et al. 2018; Chang et al. 2018). It is therefore conceivable that Plac8 could promote the differentiation of the simple lecithotrophic follicle into the complex villous follicle seen in matrotrophic species.
A second possibility that we cannot ignore is that the Poeciliopsis placenta develops by using a novel gene regulatory network completely different from the mammalian placenta. This idea was recently proposed, because the maternal follicle of P. prolifica expresses a large proportion of transcripts with open reading frames, but no BLAST annotation (Jue et al. 2018). However, studies in live-bearing lizards (Brandley et al. 2012) and sygnathid fishes (Whittington et al. 2015) have demonstrated similar gene expression profiles to mammals. Additionally, previous studies in Poeciliopsis fishes have suggested some degree of convergence with mammalian placenta gene expression both using candidate gene expression (Lawton et al. 2005) and transcriptome profiling techniques (Panhuis et al. 2011). Although it may seem improbable that such a great degree of molecular convergence exists in such a wide variety of taxa, it has been suggested that live-bearing and placentation may simply co-opt gene expression from nearby abdominal or reproductive cell types that have conserved functions (Arendt 2008). Our finding that the commonly used mammalian placental markers Vimentin and Cytokeratin are expressed in the cognate tissue types of Poeciliopsis supports this idea, as these proteins are important in many tissue types.
Prolactin in the Evolution of Placenta
The hormone prolactin has well documented expression in the uterine and placenta tissues of therian mammals (Menzies et al. 2011). Although its exact function in pregnancy is unclear, the genetic landscape of the Prl locus hints at a critical role. In mice, Prl has undergone a massive expansion and the locus exhibits endopolyploidy in trophoblast giant cells (Hannibal and Baker 2016), a key placental cell type. Furthermore, Prl expression has been co-opted by the endometrium of several eutherian mammals using independent insertion of transposon-derived promoters (Emera et al. 2012). As we observe prolactin protein expression in the maternal follicles of only matrotrophic Poeciliopsis species, we believe this hormone is critical for complex placentation. Intriguingly, we do not observe Prl mRNA expression in our transcriptome but do observe expression of its cognate receptor, Prlr. This suggests to us that the source of prolactin is somewhere outside the maternal follicle. One intriguing possibility is that the fetal portion of the placenta produces prolactin in an effort to promote differentiation of the maternal follicle so it can receive additional provisions. This is in line with previous work in H. formosa that suggests that offspring genotype influences maternal provisioning (Schrader and Travis 2009). As prolactin is a pituitary hormone, it is also possible that the pituitary gland is the source of maternal follicle prolactin. In this case, the Poeciliopsis pituitary may have evolved to support higher expression of Prl during pregnancy to maintain the maternal follicle. Future experiments should seek to identify the source of follicle prolactin and evaluate the function of Prl in Poeciliopsis pregnancy. This will require the development of new molecular tools, but similar experiments have tested gene function in a new species by leveraging a closely related model organism (Kamberov et al. 2013) and CRISPR transgenic tools are actively being developed for nontraditional model vertebrates (Rasys et al. 2019) making these plausible next steps.
Implications for Maternal–Fetal Conflict
It has been hypothesized that the advent of live birth creates a new arena for postfertilization genomic conflict, which drives rapid antagonistic coevolution (Haig 1993; Zeh DW and Zeh JA 2000; Crespi and Semeniuk 2004). The developing offspring has a selective advantage in selfishly taking as many resources as possible, whereas the pregnant mother has to finely regulate provisions to promote offspring survival and also ensures her own ability to survive and reproduce again. Although this phenomenon has been most thoroughly studied in the context of genomic imprinting in mammals (Reik et al. 2003; Stringer et al. 2012), there is mounting evidence that this conflict has consequences for many live-bearing vertebrates (Crespi and Semeniuk 2004; Schrader and Travis 2008, 2009; Chuong et al. 2013). One gene that has been central to this work is Igf2, a paternally imprinted gene in mammals that promotes embryonic resource acquisition (Reik et al. 2003). Although Igf2 does not appear to be imprinted in Poeciliopsis (Lawton et al. 2005), it is under positive selection in fish lineages where placentation has evolved, including Poeciliopsis (O’Neill et al. 2007). Interestingly, Igf2, and its downstream target Atk1 (Kent et al. 2012), are expressed in the follicle transcriptome of P. retropinna and P. turrubarensis. We also find expression of Phlda2, a maternally imprinted gene in mammals, which acts to restrict the amount of resources provided to the placenta (Tunster et al. 2016). The expression of these genes in matrotrophic and lecithotrophic follicles suggests that the groundwork for conflict at the maternal–fetal interface is already in place before the emergence of complex placentation and may be a phenomenon to consider more broadly in all live-bearing species. This is consistent with the recent finding that fish eggs are capable of active uptake of organic molecules, suggesting that they are poised for maternal provisioning (Morrison et al. 2017).
The maternal immune system is also at the center of maternal–fetal conflict (Haig 1993). The foreign genome of the developing offspring could cause the mother to launch an antagonistic immune response; as such immunity must be tightly regulated. Surprisingly, we find that only the genes uniquely and more highly expressed in P. retropinna are enriched for ontology related to an adaptive immune response (P-adj = 0.000399), whereas no immunity ontology is associated with genes uniquely and more highly expressed in P. turrubarensis. We also find expression of A2m only in the transcriptome of P. retropinna and, interestingly, expression of this gene has been detected in two other matrotrophic Poeciliopsis species (Panhuis et al. 2011). In the rodent placenta, A2m is expressed in the decidua where it prevents excessive invasion by fetal trophoblast tissue (Gu et al. 1992). As there is no active invasion of the maternal follicle by the fetus, we do not believe that this is its function in Poeciliopsis. However, A2m has also been shown to play a role in immunosuppression (Hubbard et al. 1983), a function critical for mediating conflict at the maternal interface. Additionally, the expression of A2m in rodent decidual tissue is directly regulated by prolactin (Gu et al. 1992). Interestingly, A2m transcripts overlap in the P. retropinna maternal follicle with prolactin protein. This suggests that prolactin is directly promoting A2m expression leading to immunosuppression in the maternal follicle, which prevents maternal immune responses that might harm the developing offspring. This adds intrigue to the hypothesis previously presented, that the fetus might be the source of prolactin that ultimately immunosuppresses its mother to promote its own survival. Taken together, these immunological genes expressed exclusively in matrotrophic Poeciliopsis suggest that conflict is more active when there is more extensive maternal provisioning.
Conclusion
Our findings demonstrate that placentation in Poeciliopsis shares a molecular signature with mammal placentas. This is evident in both matrotrophic and lecithotrophic species, which we suggest, indicates that lecithotrophic Poeciliopsis are poised for the formation of complex placental structures. We also identify potential key molecules that may facilitate the transition to maternal provisioning. Prolactin is especially intriguing as it is uniquely expressed in three independently evolved Poeciliopsis complex placentas. As we identified this difference in all three origins, two of which have lecithotrophic sister species (P. prolifica and P. infans) and (P. turneri and P. gracilis), this provides strong evidence that common elements are being co-opted repeatedly in the evolution of placentation. Overall, our study provides the first direct transcriptome comparison between matrotrophic and lecithotrophic Poeciliopsis species, which highlights molecular convergence, both with the mammalian placenta and in each of the three complex placenta origins in Poeciliopsis.
Materials and Methods
Sample Collection and Preparation
Pregnant female P. retropinna and P. turrubarensis were collected in Costa Rica, under permit number R-SINAC-ACLAP-011-2018, at Rio Ceibo (N09°12′, W83°18′), Rio Potrero Grande (N09°01′, W83°09′), Rio Seco (N08°39′, W82°56′), Rio Sucio (N08°49′, W82°55′). Ovaries were dissected in Costa Rica, where three from each site were placed in RNA later for fine dissection of the maternal follicle. At least one additional ovary from each site was placed in a solution of 4% paraformaldehyde to be used in imaging studies. All samples were stored in a cooler with ice until they could be returned to the United States for further processing.
Pregnant female P. gracilis, P. infans, P. prolifica, and P. turneri were supplied from existing stocks at the University of California, Riverside. Ovaries were dissected from these fish and placed in 4% paraformaldehyde to fix overnight at 4 °C.
Ovaries for imaging were then processed rocking at room temperature as follows: five 10-min washes in 1× phosphate buffered saline (PBS), 1-h wash in each 70%, 85%, 90%, and 95% ethanol, three 20-min washes in 100% ethanol, and three 10-min washes in xylenes. Samples were then placed in molten paraffin at 65 °C for 1 h after which the paraffin was replaced and samples were left overnight. All ovaries were embedded into paraffin blocks the next day. Sections were subsequently taken from each of these blocks at a 5-μm thickness on a Leica RM 2155, slides were left to dry overnight at 37 °C, and then slides were stored at room temperature for future use.
H&E Staining
Slides for H&E were processed as follows: two 5-min washes in xylenes, two 5-min washes in 100% ethanol, 5-min washes in each 95%, 85%, 70%, 50%, and 30% ethanol, one 5-min wash in tap water, one 2-min wash in hematoxylin, rinse slides for 5 min in running tap water, 5-min washes in each 30%, 50%, and 70% ethanol, one 2-min wash in eosin, 5-min washes in each 70%, 85%, and 95% ethanol, two 5-min washes in 100% ethanol, and two 5-min washes in xylenes. Slides are then mounted using permount, and slides are left to dry overnight at room temperature. Slides were visualized on a Leica DMRX A2 light microscope, and photographs were taken using Leica application suite software v4.2.0.
RNA Sequencing
Ovaries from Costa Rica stored in RNAlater were returned to the lab, where the maternal follicles from late stage (I and J) (Haynes 1995) were dissected and transferred to TriZol. The follicles from all late-stage embryos in each ovary were combined in TriZol to obtain adequate RNA. RNA was extracted from follicles using the Direct-zol RNA miniprep kit. Total RNA was depleted using the protocol described by Adiconis et al. (2013), though this depletion was only partial because Poeciliopsis DNA oligos tilling rRNAs are not available, so human DNA oligos were used. Libraries were prepared using NEBNext Ultra II Directional RNA Library Prep Kit for Illumina for use with rRNA depleted RNA. Briefly, 100 ng of each RNA sample was fragmented for 7 min, primed using random primers, then cDNA synthesis was performed, samples were end-prepped, and NEBNext adapters were ligated to the resulting cDNA. All samples were enriched for adapter-ligated DNA using a seven-cycle polymerase chain reaction, where each sample was given a unique index primer. Samples were then purified with Ampure XP beads, library quality was assessed with Bioanalyzer and Qubit, libraries were multiplexed, and finally libraries were sequenced on an Illumina NextSeq using the 150-cycle High Output v2 kit.
Sequence Analysis
A transcriptome mapping reference was made in Kallisto using the Kallisto index function (Bray et al. 2016) on the coding sequence FASTA files from the P. retropinna (PRJNA555005) and P. turrubarensis (PRJNA555006) genomes (van Kruistum et al. 2020). The reads were then aligned to the index, and gene expression was quantified using the Kallisto quant function (Bray et al. 2016). The est_counts from Kallisto were than normalized using the bioconductor package DESeq2 in R (Love et al. 2014). Data processed in this study are deposited at the Gene Expression Omnibus, accession number GSE138615.
To compare the expression of transcripts between P. retropinna and P. turrubarensis, we first established a gene expression cutoff. Because these libraries were so deeply sequenced, many very lowly expressed transcripts were present in both transcriptomes. To prevent these transcripts from complicating our analysis, we established a cutoff at the median normalized est_counts for each transcriptome, 3.2877 for P. retropinna and 6.1751 for P. turrubarensis. Transcripts below these cutoffs were excluded from further analysis, and dubbed not expressed. The highest est-counts for any duplicate genes were used in subsequent analyses. The gene lists from each species were compared to identify genes expressed only in one species. We then compared the genes expressed in both species using DESeq2 (Love et al. 2014). We combined all replicates of P. retropinna and compared them with all replicates of P. turrubarensis using differential expression analysis. We included any transcripts significantly up- or downregulated (Benjamini adjusted P < 0.05) in subsequent analysis. Gene Ontology analysis of biological processes was performed in the Gene Ontology Consortium (Ashburner et al. 2000; Anon 2019) using the Bonferroni correction for multiple testing to identify meaningful differences between matrotrophic and lecithotrophic Poeciliopsis.
To compare expression of genes between different river populations of P. retropinna, we averaged the counts between replicates from each river population and performed pairwise differential expression analyses in DESeq2. We compared Ceibo with Potrero Grande, Ceibo with Sucio, Ceibo with Seco, Potrero Grande with Sucio, Potrero Grande with Seco, and Sucio with Seco. We included any transcripts significantly up- or down-regulated (Benjamini adjusted P < 0.05) in any of these comparisons in subsequent analysis. Gene Ontology analysis was completed as described above to identify genes that might be driving rapid changes in maternal provisioning. Gene lists for all analyses are contained in supplementary file 4, Supplementary Material online.
RNA Fluorescent In Situ Hybridization
Poeciliopsis retropinna probes for the genes A2m, Cpe, and Plac8 were designed spanning intron junctions. The probes were ordered as g-blocks from Integrated DNA Technologies with leading T7 polymerase sequence. Sequences for A2m, Cpe, and Plac8 probes are contained in supplementary file 5, Supplementary Material online. RNA probes were then synthesized using the Roche Dig Kit with an input of 200 ng of each g-block. Probes were purified on mini Quick Spin RNA columns and quantified using nanodrop.
Slides for in situ were processed as follows: Slides were rehydrated, antigen retrieval was performed in a pressure cooker using sodium citrate buffer, three 10-min xylenes washes, two 10-min 100% ethanol washes, 5-min washes each of 95%, 70%, and 50% ethanol, and one 5-min wash in deionized water. Slides were then placed in sodium citrate buffer (pH 6.0) for a 13-min antigen retrieval in a pressure cooker. Slides are then treated as follows: one 5-min wash in deionized water, two 5-min washes in 1× PBS, one 15-min wash in 1× PBS containing 0.3% TritonX, and three 5-min washes in 1× PBS. Slides are then washed in a 4 μg/ml proteinase K solution at 37 °C for 10 min and rinsed three times for 5 min with 1× PBS. Slides are then acetylated for 10 min and rinsed three times for 5 min with 1× PBS. Slides were than incubated in a prehybridization solution for 3 h. Probes were denatured at 80 °C for 5 min, chilled, and added to prehybridization solution at a final concentration of 600 ng/ml. The probes were then hybridized to slides overnight at 60 °C. The following day slides are rinsed with 5× saline sodium citrate (SSC) and two 30-min washes in 1× SSC are completed at 37 °C. Slides are then moved to room temperature where one 5-min wash in 1× SSC, three 10-min washes in 1× maelic acid buffer (MAB)–3% Tween, and two 10-min washes in 1× MAB (pH 7.5) are completed. Slides are blocked in a sheep serum based blocking solution for 30 min at room temperature and the anti-DIG POD antibody is diluted 1:10,000 in sheep serum block and applied to the slides overnight at 4 °C. The following day complete two 10-min washes in 1× MAB–3% Tween, three 10-min washes in 1× PBS–0.1% Tween, and then apply Perkin Elmer Tyramide signal amplification Cyanine-3 reagent to slides for 6 min. Finally, complete three 10-min washes in 1× PBS–0.1% Tween and then mount slides with ProLong gold antifade mount with 4′,6-Diamidino-2-Phenylindole, Dihydrochloride (DAPI). Slides were visualized on a Leica DMRX A2 light microscope and photographs were taken using Leica application suite software v4.2.0.
Immunofluorescence
All slides for immunofluorescence were processed and visualized as previously described (Guernsey et al. 2017). The primary antibodies used for experiments were a mouse monoclonal anti-Vimentin (sigma v5255) at a 1:200 dilution, mouse monoclonal anti-Pan-Cytokeratins AE1/AE3 (BioLegend, 914201) at a 1:7,500 dilution, and rabbit monoclonal anti-prolactin (Abcam, EPR19386) at a 1:200 dilution. Secondary antibodies used in experiments were biotinylated goat anti mouse IgG (Jackson Immunoresearch, 111-065-166) at a 1:1000 dilution for Vimentin and Pan-Cytokeratin and biotinylated goat anti-rabbit IgG (Jackson Immunoresearch, 111-065-144) at a 1:5,000 dilution for prolactin.
Supplementary Material
Supplementary data are available at Molecular Biology and Evolution online.
Acknowledgments
We thank Guillaume Cornelis and Jessica Chang for advice on data analysis, Kelly McGowan for aide in learning IF and RNA-FISH, Andres Hagmayer and Andrew Furness for aide collecting Poeciliopsis samples in Costa Rica, Yuridia Reynoso for help gathering samples at UCR, and Tami Panhuis for teaching Poeciliopsis dissection techniques. This work was supported by a National Science Foundation Graduate Research Fellowship (2014185511 to M.W.G.), a Stanford VPGE Diversifying Academia, Recruiting Excellence (DARE) Fellowship (to M.W.G.), and an NSF Evo-Devo-Eco Network (EDEN) Research Exchange Grant to work in the Reznick lab at UCR (IOS-0955517 to M.W.G.).
RNA sequencing data generated in this study are deposited at the Gene Expression Omnibus under the accession GSE138615.
References
Anon.