-
PDF
- Split View
-
Views
-
Cite
Cite
Arnau Sebé-Pedrós, Alex de Mendoza, B. Franz Lang, Bernard M. Degnan, Iñaki Ruiz-Trillo, Unexpected Repertoire of Metazoan Transcription Factors in the Unicellular Holozoan Capsaspora owczarzaki, Molecular Biology and Evolution, Volume 28, Issue 3, March 2011, Pages 1241–1254, https://doi.org/10.1093/molbev/msq309
- Share Icon Share
Abstract
How animals (metazoans) originated from their single-celled ancestors remains a major question in biology. As transcriptional regulation is crucial to animal development, deciphering the early evolution of associated transcription factors (TFs) is critical to understanding metazoan origins. In this study, we uncovered the repertoire of 17 metazoan TFs in the amoeboid holozoan Capsaspora owczarzaki, a representative of a unicellular lineage that is closely related to choanoflagellates and metazoans. Phylogenetic and comparative genomic analyses with the broadest possible taxonomic sampling allowed us to formulate new hypotheses regarding the origin and evolution of developmental metazoan TFs. We show that the complexity of the TF repertoire in C. owczarzaki is strikingly high, pushing back further the origin of some TFs formerly thought to be metazoan specific, such as T-box or Runx. Nonetheless, TF families whose beginnings antedate the origin of the animal kingdom, such as homeodomain or basic helix-loop-helix, underwent significant expansion and diversification along metazoan and eumetazoan stems.
Introduction
What genomic changes took place at the dawn of the Metazoa remains a major biological question. Transcriptional regulation appears to be one of the most crucial aspects of animal development. Thus, understanding the early evolution of the transcriptional regulatory machinery is critical for drawing a complete picture of metazoan origins. Transcription factors (TFs) act as regulators of cell fate, cell cycle, patterning, proliferation, development, and differentiation in metazoans (Larroux et al. 2008). Previous studies have shown that most TFs that play important roles in bilaterian development originated before the divergence of extant animal phyla (Larroux et al. 2006, 2008; King et al. 2008; Degnan et al. 2009; Srivastava et al. 2010). However, the complexity of most TF families appears to have increased during early eumetazoan evolution, with cnidarians having a TF gene repertoire typically being two to three times larger than that of sponges and placozoans (Putnam et al. 2007; Degnan et al. 2009; Srivastava et al. 2010). Based on comparative analyses, it has been hypothesized that the metazoan TF “toolkit” included members of the basic helix-loop-helix (bHLH), myocite enhancer factors 2 (Mef2), Fox, Sox, T-box, Ets, nuclear receptor (NR), Rel/nuclear factor-kappaB (NF-kappaB), basic-region leucine zipper (bZIP), and Smad families and a range of homeobox-containing classes, including ANTP, Prd-like, Pax, POU, LIM-HD, Six, and three-amino acid-loop extension (TALE) (for a review, see Degnan et al. 2009).
Comparative analyses including the holozoan choanoflagellate Monosiga brevicollis, the putative sister-group to metazoans, are greatly improving our understanding of metazoan TF evolution. The genome of M. brevicollis contains the standard set of TFs observed across eukaryotes but lacks most of the well-known metazoan TFs, except p53, Myc, and a putative Sox (King et al. 2008; Degnan et al. 2009). Under this scenario, metazoan-specific TFs appear to include ANTP, Prd-like, POU, LIM-HD, and six homeobox genes, group I Fox, most bHLH groups (except B), some bZIP families, Ets, Runx, Mef2, and NR families (Degnan et al. 2009).
To gain further insight into the evolution of TFs leading to the metazoan lineage, we characterized and analyzed all the TFs that supposedly constitute the metazoan TF toolkit in another close unicellular relative of animals, the amoeboid holozoan Capsaspora owczarzaki, putatively the sister-group to metazoans and choanoflagellates (Ruiz-Trillo et al. 2004, 2008; Shalchian-Tabrizi et al. 2008; Brown et al. 2009; see fig. 1). The complete genome sequence of C. owczarzaki (hereafter “Capsaspora”) has recently been obtained under the “UNICORN project” at the Broad Institute (Ruiz-Trillo et al. 2007). In addition to the TFs outlined above, our survey of the Capsaspora genome in this study also included other TFs known to be important to animal development, including Churchill, p53, Stat, and LSF/Grainyhead (GRH) (fig. 1). Comparative genomic analyses were performed on holozoan genomes, and in some cases, other recently sequenced opisthokont and apusozoan genomes, namely Allomyces macrogynus, Spizellomyces punctatus, and Thecamonas trahens (see Materials and Methods, fig. 1). These results show that the complexity of TFs in Capsaspora is very high, indicating that some TFs thought to be metazoan specific evolved prior to the metazoan and choanoflagellate divergence and were subsequently lost in the choanoflagellate lineage.
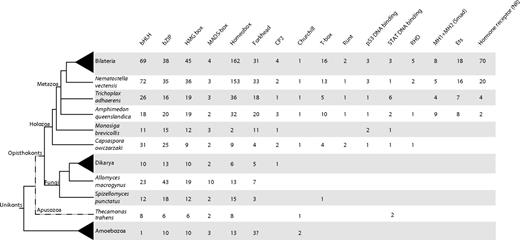
Table of domain presence and number across unikonts. Columns represent all the PFAM domains analyzed in this study. The number of genes in each TF family was inferred from each organisms’s proteome by PfamScan using the PfamScan default parameters. For Monosigabrevicollis and Capsasporaowczarzaki, the analyses were performed by HMMER 3.0 searches. For Smad proteins, containing one MH1 and one MH2 domain, the number shown is the minimal number of either MH1 or MH2. For Bilateria, Dikarya, and Amoebozoa the average number is shown. Bilateria includes Homo sapiens, Ciona intestinalis, Drosophila melanogaster, Anopheles gambiae, Caenorhabditis elegans, Helobdella robusta, and Lottia gigantea. Dikarya includes Saccharomyces cerevisiae, Schizosaccharomyces pombe, Cryptococcus neoformans, Yarrowia lypolitica, Ustilago maydis, Aspergillus niger, Neurospora crassa, and Phanerochaete chrysosporium. Amoebozoa includes Dictyostellium discoideum, Dictyostellium purpureum, Entamoeba histolytica, Entamoeba dispar, and Acanthamoeba castellanii. The phylogenetic relationships are based on several recent phylogenomic studies (Burki et al. 2008; Ruiz-Trillo et al. 2008; Brown et al. 2009; Liu et al. 2009; Minge et al. 2009).
Materials and Methods
Taxonomic Sampling
We surveyed, and characterized, a list of metazoan TFs in Capsapora. In some cases, we extended our searches to the widest possible set of eukaryotic taxa. This was the case for those TF families with specific and unique domains: T-box (T-box DNA-binding domain), Runx (Runt DNA-binding domain), NF-kappaB (Rel homology domain [RHD]), Mef2 (MADS box + Mef2 domain), p53 (p53 DNA-binding domain), Stat (Stat DNA-binding domain), Churchill (Churchill domain), Smad (MH1 + MH2 domains), Ets (Ets domain), and NR. Our extended searches included published and publicly available eukaryotic genomes, and other UNICORN taxa, such as the basal fungi A. macrogynus, S. punctatus, and the apusozoan T. trahens (see http://www.broadinstitute.org/annotation/genome/multicellularity_project/MultiHome.html). For the remaining TF families (i.e., bZIP [bZIP domain], Fox [forkhead domain], Sox [HMG box], homeobox [homeodomain], bHLH [bHLH domain], and LSF/GRH [CP2 domain]), we classified those Capsaspora genes with homology to metazoan genes. To this end, we used published fungal, metazoan, and choanoflagellate homologs. We also characterized bZIPs and Mef2 in M. brevicollis.
Gene Searches
A primary search was performed using the basic local alignment sequence tool (BLAST: BlastP and TBlastN) using Homo sapiens proteins as queries against Protein and Genome databases with the default BLAST parameters and an e value threshold of 10 × 10−5 at the National Center for Biotechnology Information (NCBI) and against completed or on-going genome project databases at the Joint Genome Institute (JGI), the Broad Institute, as well as the A. queenslandica genome database (www.metazome.net/amphimedon). In the case of T. trahens, A. macrogynus, and Acanthamoeba castellanii, we assembled the trace data using the WGS assembler (“http://sourceforge.net/apps/mediawiki/wgs-assembler/index.php?title=Main_Page”http://sourceforge.net/apps/mediawiki/wgs-assembler/index.php?title=Main_Page). We then annotated the genes of interest using both Genescan (Burge and Karlin 1997) and Augustus (Stanke and Morgenstern 2005) and performed local BLAST searches. When the BLAST searches of the genome data described above returned significant “hits,” the sequences obtained were then reciprocally searched against the NCBI protein database by BLAST in order to confirm the validity of the sequences retrieved with the initial search. Hmmer searches using HMMER3.0b2 (Eddy 1998) were also performed, with standard PFAM profiles in the case of widespread domains or with home-made profiles in the case of specific domains.
Protein Domain Arrangements
For all proteins, the presence of specific protein domains was further checked by searching the Pfam (“http://pfam.sanger.ac.uk/search”http://pfam.sanger.ac.uk/search) and SMART (“http://smart.embl-heidelberg.de/”http://smart.embl-heidelberg.de/) databases.
Polymerase Chain Reaction confirmation of C. owczarzaki T-box, Runx, and NF-kappaB Genes
We confirmed the presence of the three Capsaspora TFs that were formerly considered to be metazoan-specific TFs now identified in Capsaspora (Runx, T-box, and NF-kappaB), using gene-specific oligonucleotide primers. The mRNA was extracted using a Dynabeads mRNA purification kit (Invitrogen, Carlsbad, CA) and subsequent reverse transcriptase-polymerase chain reaction (RT-PCR) was performed using a Superscript III First Strand Synthesis kit (Invitrogen). The full sequence of the 5' and 3' ends of the cited Capsaspora TF cDNAs were obtained by RACE, using a nested PCR and with specific oligonucleotide primers designed from the original genome data. Both coding and noncoding strands were sequenced using an ABI PRISM BigDye Termination Cycle Sequencing Kit (Applied Biosystems, Foster City, CA). New sequences were deposited in GenBank under the following accession numbers: GU985459 (Capsaspora Bra-like), GU985460 (Capsaspora double-tbox), GU985461 (Capsaspora Tbox3), GU985462 (Capsaspora Runx1), GU985463 (Capsaspora Runx2), and GU985464 (Capsaspora NF-kappaB).
Phylogenetic Analyses
Alignments were constructed for the following gene families and classes: T-box, homeobox, Fox, Sox, bHLH, bZIP, LAG, signal transducer and activator of transcription (STAT), Mef2, p53, NF-kappaB, Churchill, HMG box, GRH/LSF, and Runx. Alignments were obtained using the MAFFT v.6 online server (Katoh, Kuma, Miyata, and Toh 2005; Katoh, Kuma, Toh, and Miyata 2005) and then manually inspected and edited in Geneious. Only those species and those positions that were unambiguously aligned were included in the final analyses. Maximum likelihood (ML) phylogenetic trees were estimated by RaxML (Stamatakis 2006) using the PROTGAMMAWAGI model, which uses the Whelan and Goldman (WAG) amino acid exchangeabilities and accounts for among-site rate variation with a four category discrete gamma approximation and a proportion of invariable sites (WAG + Γ + I). Statistical support for bipartitions was estimated by performing 100-bootstrap replicates using RaxML with the same model. Bayesian analyses were performed with MrBayes 3.1 (Ronquist and Huelsenbeck 2003), using the WAG + Γ + I model of evolution, with four chains, a subsampling frequency of 100 and two parallel runs. Runs were stopped when the average standard deviation of split frequencies of the two parallel runs was <0.01, usually at around 1,000,000 generations. The two LnL graphs were checked and an appropriate burn-in length established; stationarity of the chain typically occurred after ∼15% of the generations. Bayesian posterior probabilities (BPP) were used to assess the confidence values of each bipartition.
Homeodomain Gene Assignment
An alignment with members of the ANTP, Paired-like, POU, and LIM homeodomain classes was constructed using published data from Amphimedon, Drosophila, and Nematostella and other already classified sequences (Larroux et al. 2008). A RaxML best tree resulting from this phylogeny was produced to obtain the fixed topology, which recovered monophyly for all four classes. From this tree, we manually created constrained topologies that represented all the possible positions of Capsaspora non-TALE homeodomains. Site-wise log-likelihoods were calculated for all the generated topologies with RaxML. Best-scoring ML trees were chosen using the likelihood-based approximately unbiased (AU) test as implemented in CONSEL (Shimodaira and Hasegawa 2001). The positions of Capsaspora homeodomain genes that could not be statistically excluded (P ≥ 0.05) were taken into account. Whenever the significant positions fell in the branches that connect the different classes of homeodomains (POU, LIM, … ), the homeodomain was not classified. When Capsaspora hits fell inside just one cluster (e.g., Capsaspora6 inside paired-like), they were classified accordingly.
Quantitative TF analyses in Unikont Taxa
To quantify the number of genes in each TF family, we used PfamScan using the PfamScan default parameters. The predicted proteomes used for PfamScan analysis were Amphimedon queenslandica (JGI), Trichoplax adhaerens (JGI), Nematostella vectensis (JGI), H. sapiens (NCBI), Ciona intestinalis (JGI), Drosophila melanogaster (NCBI), Anopheles gambiae (NCBI), Caenorhabditis elegans (NCBI), Helobdella robusta (JGI), Lottia gigantea (JGI), Saccharomyces cerevisiae (NCBI), Schizosaccharomyces pombe (NCBI), Cryptococcus neoformans (JGI), Yarrowia lypolitica (NCBI), Ustilago maydis (JGI), Aspergillus niger (JGI), Neurospora crassa (NCBI), Phanerochaete chrysosporium (JGI), A. macrogynus (Broad Institute), S. punctatus (Broad Institute), Dictyostellium discoideum (NCBI), Dictyostellium purpureum (JGI), Entamoeba histolytica (NCBI), Entamoeba dispar (NCBI), and A. castellanii (home-made prediction). For M. brevicollis and Capsaspora, the analyses were performed by HMMER 3.0 searches. For Smad proteins, containing one MH1 and one MH2 domain, the number was inferred by taking the minimal number of either MH1 or MH2 present in the proteomes.
Results and Discussion
Rel/NF-kappaB
The RHD is a conserved DNA binding and dimerization domain that is present in the N-terminal region of two protein families: nuclear factor activated T-cells (NFAT) and Rel/NF-kappaB. NFAT and Rel/NF-kappaB are involved in immune system processes in metazoans (Macian 2005). Rel/NF-kappaB also plays different roles in development and cell differentiation, receiving inputs from several signaling pathways (Hayden and Ghosh 2004). Until now, the RHD domain has not been identified outside metazoans and was thus considered a metazoan innovation (Gauthier and Degnan 2008).
However, we identified a single RHD domain in Capsaspora but failed to recover RHD from any other sequenced nonmetazoan taxa (fig. 1). Our phylogenetic analysis of the RHD domain shows the Capsaspora homolog branching off as sister-group of all metazoan Rel/NF-kappaB (supplementary fig. S1, Supplementary Material online). Furthermore, the Capsaspora RHD-domain-containing protein shares several key features with metazoan Rel and NF-kappaB homologs, such as 1) a highly conserved and specific recognition loop located within the RHD domain, which is involved in dimerization; 2) an IPTG or RHD2 domain, which confers binding specificity; 3) a basic nuclear-localization sequence; 4) a glycine–serine rich region; and 5) several ankyrin repeats, which are exclusive to metazoan NF-kappaB proteins (supplementary fig. S2, Supplementary Material online).
Thus, our data show that the RHD domain is not exclusive to metazoans as previously thought but rather it originated prior to the divergence of Capsaspora from choanoflagellates and metazoans. This implies that the RHD domain was subsequently lost in the choanoflagellate lineage.
Runx
The Runt DNA-binding domain defines a family of metazoan TFs (Runx) with essential roles in animal development (Coffman 2003; Robertson et al. 2009). They can act as transcriptional activators or repressors, in the latter case usually via corepressors of the Groucho/TLE family (Wheeler et al. 2000). Runx genes encode the Runt DNA-binding domain and heterodimerization domain and a C-terminal WRPY motif that interacts with the Groucho/TLE corepressor (Coffman 2003), except in the demosponge A. queenslandica and some bilaterian paralogs (specifically one of the two leech and planarian paralogs), which all lack the C-terminal WRPY motif (Robertson et al. 2009). A single Runx gene is present in A. queenslandica, N. vectensis, and T. adhaerens, although most bilaterians have several copies as a result of independent duplications (Rennert et al. 2003). Runx was previously considered to be metazoan specific (Robertson et al. 2009).
We failed to recover Runx genes from any other sequenced nonmetazoan genome except Capsaspora, which has two genes (fig. 1). Both Capsaspora Runxs possess key DNA-binding amino acids in the Runt motif (Wheeler et al. 2000; Sullivan et al. 2008), although only one of the paralogs (Co_Runx1) has the two Cys residues involved in redox regulation (Akamatsu et al. 1997) (supplementary fig. S3, Supplementary Material online). Interestingly, as in A. queenslandica and one of the two leech and planarian paralogs, both Capsaspora Runx lack the specific C-terminal WRPY Groucho-interacting motif. In contrast to A. queenslandica, however, Capsaspora does not encode Groucho in its genome. Neither does Capsaspora encode CBFβ, the heterodimeric-binding partner of the Runt domain that enhances its DNA affinity (Sullivan et al. 2008). This suggests that the Runt domain acts independently from CBFβ in Capsaspora. Our results show that Runx originated prior to the divergence of Capsaspora from choanoflagellates and metazoans, being secondarily lost in the choanoflagellate lineage. We hypothesize that Runx originally functioned independently of Groucho and CBFβ proteins and that the WRPY Groucho-interacting motif appeared in the eumetazoan lineage, as previously suggested (Robertson et al. 2009).
T-box
T-box TFs are characterized by an evolutionary conserved DNA-binding motif of 180–200 amino acids, the T-box domain (Smith 1999). They are key regulators of metazoan development (Muller and Herrmann 1997). The most well-known type of T-box is Brachyury, which has a key role in mesoderm specification (Marcellini et al. 2003), although its ancestral function may have been blastopore determination and gastrulation (Scholz and Technau 2003). T-box genes were previously generally considered to be metazoan specific (King et al. 2008; Larroux et al. 2008; Rokas 2008).
Here, we report the discovery of T-box genes in two nonmetazoan species. Three T-box genes are present in Capsaspora (one containing two consecutive T-box domains), and one gene exists in the basal chytrid fungus S. punctatus (fig. 1). Our searches, however, failed to recover T-box homologs from any other fungi (including the chytrids A. macrogynus and Batrachochytrium dendrobatidis) or other eukaryote (including the choanoflagellate M. brevicollis). Remarkably, all the T-box homologs from both Capsaspora and S. punctatus contain most of the key DNA-binding and dimerization amino acids of the metazoan T-box (Muller and Herrmann 1997; Bielen et al. 2007) (supplementary fig. S4, Supplementary Material online). The phylogenetic analysis of T-box domains (fig. 2) places one Capsaspora homolog (Co-Bra) inside the Brachyury family (bootstrap value [BV] = 50%). The two T-box domains in the Capsaspora “double-tbox” (Co-Dtbx1 and Co-Dtbx2) and the S. punctatus T-box clearly cluster together adjacent to the Brachyury family. The third Capsaspora homolog (Co-Tbx3) clusters within a group of unclassified T-box genes from the sponge A. queenslandica that may represent an independent and novel class of T-box genes. Our general topology supports the hypothesis that Brachyury is probably the ancestral class within the T-box family (Adell et al. 2003; Adell and Muller 2005; Larroux et al. 2008). Moreover, our findings imply that T-box genes appeared not in metazoans but in the common ancestor of opisthokonts and were subsequently lost in most fungi and in choanoflagellates.
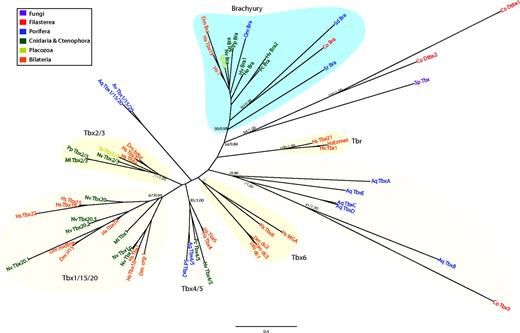
ML tree of T-box domains showing the different T-box families. The tree is rooted using the midpoint-rooted tree option. Statistical support was obtained by RAxML with 1,000 bootstrap replicates (BV) and BPP. Both values are shown on key branches. Colors show different taxonomic assignments. Aq (Amphimedon queenslandica), Av (Axinella verrucosa), Co (Capsaspora owzarzaki), Dm (Drosophila melanogaster), He (Hydractinia echinata), Hs (Homo sapiens), Hv (Hydra vulgaris), Ml (Mnemiopsis leydi), Nv (Nematostella vectensis), Om (Oopsacas minuta), Pc (Podocoryne carnea), Pp (Pleurobrachia pileus), Sd (Suberites domuncula), Sp (Spizellomyces punctatus), Sr (Sycon raphanus), Ta (Trichoplax adhaerens). Co-Dtbx1 and Co-Dtbx2 are the two T-box domains of the same T-box Capsaspora gene (for further details, see main text).
Churchill
Churchill is a zinc-finger TF that is involved in cell movement and cell fate determination (Londin et al. 2007). In Xenopus and chick, Churchill appears to regulate the T-box gene brachyury (Sheng et al. 2003). We have found orthologs of Churchill in Capsaspora, T. trahens, and, interestingly, also in the amoebozoan A. castellanii (fig. 1 and supplementary fig. S5, Supplementary Material online). This finding indicates a deeper origin of this gene than previously thought probably in the common ancestor of unikonts. This suggests that Churchill was secondarily lost in fungi, and choanoflagellates as well as in other amoebozoans. What role the Churchill orthologs play in Capsaspora, T. trahens, or A. castellanii, and whether, in Capsaspora, it is related at all to its T-box genes is unknown.
p53
The p53 tumor suppressor protein is a multifaceted TF that is involved in different cellular responses to DNA damage, such as DNA repair, cell cycle arrest, senescence, and apoptosis (Coutts and La Thangue 2005; Espinosa 2008). The p53 family includes p53, p63, and p73, the last two being more closely related to each other than to p53. The three p53 members have some differences in function and in the protein domain architecture. The p63 and p73 share an additional C-terminal sterile alpha motif (SAM) domain, whereas all three share a transcriptional activation domain, a DNA-binding domain, and C-terminal tetramerization domain (Nedelcu and Tan 2007). Choanoflagellates have both a p53 and a p63/73 classes (Nedelcu and Tan 2007).
Here, we characterize a unique member of the p53 gene family in Capsaspora (fig. 1 and supplementary fig. S6, Supplementary Material online), the gene encodes a SAM domain. The phylogenetic analysis places Capsaspora-p53/63/73 close to the choanoflagellate group (supplementary fig. S7, Supplementary Material online). The tree topology implies that the last common ancestor of holozoans had a single p53/63/73 gene, which followed independent divergences in vertebrates and choanoflagellates. In the absence of DNA damage, p53 appears to be downregulated by ubiquitination, which in vertebrates is carried out by the vertebrate-exclusive Mdm2 protein. However, other mechanisms of regulation have been proposed, such as ubiquitin ligases or CREB-binding protein (CBP)/p300 (Shi et al. 2009). Interestingly, we identified CBP/p300 both in Capsaspora and M. brevicollis (see below), although whether CBP/p300 downregulates p53 in these holozoans remains unknown.
Stat
STAT proteins are TFs that, in response to a wide variety of extracellular signaling proteins, regulate the action of several genes that are involved in cell growth and homeostasis (Bromberg 2002; Levy and Darnell 2002). Structurally, STAT proteins have a N-terminal interacting domain, a STAT alpha domain with a coiled-coil structure involved in protein–protein interactions (e.g., it recruits HATs, specially CBP/p300), a STAT DNA-binding domain, a SH2 domain, and a C-terminal transactivation domain (Levy and Darnell 2002) (supplementary fig. S8, Supplementary Material online). The activation of STAT is mediated by the phosphorylation of a key tyrosine residue located after the SH2 domain (Levy and Darnell 2002). Our searches identified well-conserved STAT proteins in Capsaspora, M. brevicollis, and the apusozoan T. trahens (fig. 1). The STAT proteins from the latter two taxa appear, however, to be slightly truncated at the 5' end (see supplementary fig. S8, Supplementary Material online). STAT proteins had previously been identified in amoebozoans (Kawata et al. 1997; Lee et al. 2008; Araki et al. 2010), but the protein domain analysis clearly showed that amoebozoan STAT are quite different from metazoan STAT proteins (supplementary fig. S8, Supplementary Material online). In contrast, the homologs from M. brevicollis, Capsaspora, and T. trahens are very similar to metazoan STATs. Moreover, a phylogenetic analysis of STATs using amoebozoan CudA proteins as outgroup (Yamada et al. 2008) showed amoebozoan-specific STATs as a sister-group to the holozoan + apusozoan clade (BV = 92%) (supplementary fig. S9, Supplementary Material online). As STAT proteins are present in extant apusozoans, these are likely to have been lost early in the fungal lineage.
Metazoan STAT proteins form part of the JAK signaling pathway, which is absent in nonmetazoan lineages (King et al. 2008). However, STAT proteins can interact with other receptor and nonreceptor tyrosine kinases (Kawata et al. 1997; Levy and Darnell 2002). Indeed, the distribution of STATs coincides with the distribution of tyrosine kinases among eukaryotes, being present in amoebozoans (Kawata et al. 1997; Goldberg et al. 2006), apusozoans and Capsaspora (Ruiz-Trillo I, unpublished data), choanoflagellates (King et al. 2008; Manning et al. 2008; Suga et al. 2008), and metazoans (Mayer 2008), all of which also have tyrosine kinases.
bZIP
bZIP TFs are named after the highly conserved structure containing a basic region and a leucine zipper (Hurst 1994). The bZIP proteins are ubiquitous among eukaryotes and are involved in several processes, such as environmental sensing and development (Deppmann et al. 2006). We have identified 25 and 15 bZIP proteins in Capsaspora and M.brevicollis, respectively. Amphimedon queenslandica has 20, and the average bilaterian, 38 (fig. 1). Interestingly, the chytrid fungus A. macrogynus has 43 bZIP genes, whereas most Dikarya have approximately 13 (fig. 1). We could only classify unambiguously seven and six of the bZIP proteins present in Capsaspora and M. brevicollis, respectively. A phylogenetic analysis including only the classified proteins showed that Capsaspora bZIPs correspond to PAR, C/EBP, Atf2, Oasis, Atf6, and CREB families, whereas the Monosiga homologs correspond to Atf4/5, Atf2, Oasis, and Atf6 families (fig. 3, see supplementary fig. S10, Supplementary Material online for a tree with all Capsaspora genes). Based on these analyses, we hypothesize that most, if not all, current metazoan bZIP families were present in the holozoan ancestor, with some of them subsequently being lost in choanoflagellates and Capsaspora. Some families, such as CREB, most likely underwent a protein domain rearrangement within metazoans, similarly to that described in other gene families (King et al. 2008; de Mendoza et al. 2010). Interestingly, all bZIP proteins that we identified in the unicellular relatives of metazoans belong to families that act strictly (Atf6, PAR, CREB, Oasis) or facultatively (Atf4/5, Atf2, C/EBP) as homodimers. This suggests that bZIP proteins in unicellular organisms may work mostly as homodimers, as already seen in yeast bZIP interactions (Deppmann et al. 2006). Our data suggest that although bZIP originated before the dawn of the Metazoa, their connectivity and combinatorial interactions may have increased in animals. For example, the Capsaspora homolog of CREB does not have the kinase-inducible activation domain that allows its interaction with p300/CBP (Giebler et al. 2000), even though p300/CBP is present in the Capsaspora genome.

ML tree of bZIP genes including the unambiguously assigned Capsaspora bZIP homologs. The tree is rooted using the midpoint-rooted tree option. Statistical support was obtained by RAxML with 1,000 bootstrap replicates (BV) and BPP. Both values are shown on key branches. Aq (Amphimedon queenslandica), Co (Capsaspora), Mb (Monosiga brevicollis). Metazoan branches depicted in red and fungal branches in green. For each family, the signature sequence for DNA recognition is indicated and only proteins with this conserved motif are included in the family (Fujii et al. 2000). A tree including all Capsaspora bZIP genes is shown in supplementary figure S10 (Supplementary Material online).
bHLH
bHLH is a domain that is present in a large superfamily of TFs that are widespread among eukaryotes. In metazoans, they regulate critical developmental processes, such as neurogenesis, sex determination, myogenesis, and hematopoiesis (Jones 2004). This family of TFs has a DNA-binding basic region followed by two alpha helices separated by a variable loop region. Many bHLH proteins also include other domains that are involved in protein–protein interactions (Simionato et al. 2007). The bHLH proteins can act as homodimers or heterodimers to regulate gene expression. Metazoan bHLH have been grouped into six different higher order clades (A to F) (Simionato et al. 2007; Degnan et al. 2009) (for a general overview, see fig. 4). Group A, which includes genes such as MyoD and neurogenin, has only a bHLH domain and is exclusive to metazoans. Group B, which includes Myc or SREBP, has a leucine zipper 3' to the bHLH domain and is found throughout the eukaryotes. Group C, which includes Clock and ARNT, has two PAS domains (PAS and PAS3) 3' to the bHLH domain and is also thought to be exclusive to metazoans. Group D, which is metazoan specific, lacks the DNA-binding basic region, and hence, their members are unable to bind to DNA, acting as antagonists to Group A members. Most group E proteins include a metazoan-specific orange domain and a WRPW peptide in their carboxyl terminal part. Finally, Group F lacks the DNA-binding basic region but possesses a COE domain, which is involved both in dimerization and binding (Simionato et al. 2007).
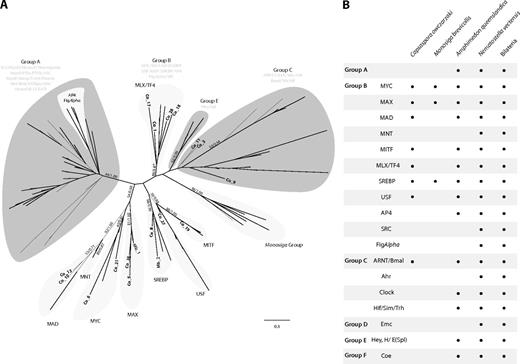
(A) ML tree of the bHLH domain including the unambiguously assigned Capsaspora bHLH homologs. The tree is rooted using the midpoint-rooted tree option. Statistical support was obtained by RAxML with 100 bootstrap replicates (BV) and BPP. Both values are shown on key branches. Groups and families are defined by the classification of Simionato et al. (2007). The taxa used were Homo sapiens, Nematostella vectensis, Amphimedon queenslandica, Monosigabrevicollis (Mb) and Capsaspora (Co). For the sake of clarity, only the last two are specifically shown. (B) Table of the presence/absence of bHLH groups and families in some key taxa. Amphimedon queenslandica, N. vectensis, and Bilateria data obtained from Simionato et al. (2007). A tree including all Capsaspora bHLH genes is shown in supplementary figure S11 (Supplementary Material online).
We identified 31 bHLH proteins in Capsaspora, including orthologs of Myc, Mad, Max, SREBP, Mlx/TF4, MITF, and USF group B families, nonspecific homologs (ARNT-like) of group C, and some unclassifiable proteins (see figs. 1 and 4, supplementary figs. S11–Supplementary Data, Supplementary Material online). This is more than double the bHLH genes found in Monosiga (11) and most fungi (around 10 in Dikarya). Compared with Metazoa, Capsaspora has a wider bHLH repertoire than the sponge A. queenslandica (18), but half what is present in cnidarians (72 in N. vectensis) or bilaterians (average of 69) (fig. 1). The choanoflagellate M. brevicollis contains Max, SREBP, Myc, and a lineage-specific group of bHLH genes. Thus, homologs of group C of bHLH were already present in the common ancestor of Capsaspora, choanoflagellates, and metazoans. Our data reveals that a basic Myc, MAX, Mxd/Mnt network of bHLH TFs was already present in the common ancestor of metazoans and Capsaspora and became more complex in multicellular lifestyles, incorporating, for example, Mnt and Mga. Interestingly, Capsaspora bHLH proteins are all homologs of those implicated in cell cycle and metabolism, and none of those are involved in differentiation. From our survey, we can also corroborate the two expansions periods (of bHLH groups and classes) in bHLH evolution previously inferred by Simionato et al. (2007) and later revised by Degnan et al. (2009), one before the divergence between Capsaspora and choanoflagellates + metazoans and another early in eumetazoan evolution (fig. 1). In contrast to bZIP TFs, there are some putative heterodimeric TF interactions among Capsaspora bHLH. For example, Myc, Mad, MAX, and Mlx can act as heterodimers in metazoans.
Mef2
Mef2 are the metazoan representatives of Type II MADS box genes. They are characterized by the presence of a Mef2 domain following the N-terminal MADS domain (Alvarez-Buylla et al. 2000). Mef2 genes play important roles in metazoan development, especially in the mesoderm (Potthoff and Olson 2007). Some authors considered Mef2 to be metazoan specific (Larroux et al. 2006; Degnan et al. 2009), although other authors had proposed Saccharomyces Smp1 and Rlm1 genes to be fungal homologs of metazoan Mef2 (Dodou and Treisman 1997). We identified canonical metazoan-type Mef2 in Capsaspora and in the cythrid fungi S. punctatus and A. macrogynus (fig. 1). The protein structure of Capsaspora and S. punctatus Mef2 closely resembles the canonical metazoan Mef2 (supplementary fig. S14, Supplementary Material online). We also identified a putative Mef2 homolog in M. brevicollis and in the amoebozoans D. discoideum, D. purpureum, E. histolytica, and A. castellanii as well as in the oomycetes Phytophthora sojae, P. ramorum, P. infestans, and P. caspis, although their sequences are divergent and have little similarity to the canonical metazoan Mef2 (supplementary fig. S14, Supplementary Material online). The fact that Phytophthora species encodes a Mef2 homolog may be explained by a lateral gene transfer (LGT) event because they are the only analyzed eukaryotes outside opisthokonts and amoebozoans to have a mef2 gene. In fact, it has already been shown that some Phytophthora genes have a close relationship with amoebozoans genes (Tyler et al. 2006; Torruella et al. 2009). A phylogenetic analysis using fungal sequences as outgroup yields a clade that comprises all the taxa with a canonical metazoan-type Mef2 domain, that is all metazoans plus Capsaspora, A. macrogynus, and S. punctatus (supplementary fig. S15, Supplementary Material online). Our data show that the canonical metazoan Mef2 domain has a deeper origin than previously thought, with a conserved Mef2 domain present at least in the common ancestor of opisthokonts.
Fox
Fox genes TFs are characterized by the presence of a DNA-binding domain known as Forkhead box. Fox genes play important roles as regulators of both development and metabolism, and they seem to be specific to opisthokonts (Tuteja and Kaestner 2007a, 2007b; Shimeld et al. 2009). We identified four Fox genes in Capsaspora, none of them being part of the metazoan-specific class I but rather present in the supposedly opisthokont specific class II that also includes fungi (Larroux et al. 2008) (fig. 1 and supplementary fig. S16, Supplementary Material online). Interestingly, we identified three putative Fox genes in the amoebozoan A. castellanii (fig. 1), although their sequences are divergent compared with opisthokont ones. Thus, our results show that Fox genes are not specific to opisthokonts and were already present before the divergence of amoebozoans and opisthokonts.
HMG Box Genes
HMG box containing genes are TFs that are involved in genome stability, chromatin structure, and gene regulation (Stros et al. 2007). Metazoan-specific families are Sox and Tcf/Lef (Larroux et al. 2008). We characterized nine HMG box-containing proteins in Capsaspora (fig. 1 and supplementary fig. S17, Supplementary Material online), a similar number as those found in Monosiga (12) and Amoebozoa (average of 10) and significantly less than those found in Bilateria (average of 45). Two of Capsaspora HMG box genes have strong similarities to MATalpha box, typical sex-determinant genes that are present in Ascomycota (Fraser and Heitman 2003; Fraser et al. 2004). Capsaspora also encodes a HMG-B, a SSRP-1, and a SWI/SNF homolog, plus some HMG box containing genes that cannot confidently be assigned to any HMG box class.
Homeobox Genes
Homeobox genes encode an acid helix-turn-helix DNA-binding motif known as the homeodomain. Homeobox genes are known to have key roles in animal, plant, fungal, and amoebozoan development, such as regional patterning, regulation of cell proliferation, differentiation, adhesion, and migration (Gehring et al. 1994; Derelle et al. 2007). There are two large superfamilies, the canonical (non-TALE) class with a 60 amino acids homeodomain and the TALE superclass characterized by an insertion of three amino acids between helix 1 and 2 of the homeodomain (Mukherjee and Burglin 2007). Both TALE and non-TALE superclasses were already present in the ancestor of eukaryotes (Derelle et al. 2007). The two homeobox genes of the choanoflagellate M. brevicollis have already been characterized, both of them belonging to the TALE superclass, although they cannot confidently be assigned to any major metazoan homeobox family (King et al. 2008; Larroux et al. 2008). We identified nine homeodomain-containing genes in Capsaspora: three TALE and six non-TALE (fig. 1 and supplementary figs. S18–Supplementary Data, Supplementary Material online). A phylogenetic analysis of these genes including members of all major families of homeodomains from metazoans, amoebozoans, and fungi failed to confidently assign Capsaspora homeobox genes to any of the major metazoan classes, except for one clear ortholog to the longevity assurance homolog (LAG-1) class. To further improve the resolution and classify the remaining Capsaspora homeobox genes, we performed phylogenetic analyses specific for TALE or non-TALE genes. This allowed us to assign one Capsaspora TALE homeobox gene to the PBC family, although it lacks the PBC N-terminal domain, and support is not very high. The remaining two Capsaspora TALE genes have an unclear phylogenetic relationship to other TALEs, although they appear to be closely related to the two M. brevicollis homeobox genes (supplementary fig. S19, Supplementary Material online). Interestingly, the sponge A. queenslandica appears not to have a homolog of PBC (supplementary fig. S19, Supplementary Material online) (Larroux et al. 2008). Capsaspora non-TALE genes appeared in unclear phylogenetic positions even with a restricted non-TALE only data set, although there is a potential homolog of LIM and two potential homologs of POU (supplementary fig. S20, Supplementary Material online). Thus, in order to classify them, we constructed different phylogenetic trees in which Capsaspora genes were forced to be members of a specific family and then we compared the likelihood values among all possible trees (for further details, see Material and Methods). Four Capsaspora non-TALE homologs appear to be at the root of the tree. Another one (Capsaspora-6) falls within the paired-like (Prd-like) clade with significant statistical support, this gene product possesses five of the six diagnostic amino acids of Prd-like genes (Galliot et al. 1999) (supplementary fig. S21, Supplementary Material online). However, it does not have the typical Q or K amino acid at position 50, and its intron is not located in the typical position (between codons 46 and 47), as consistently observed in metazoans. A specific phylogeny of ANTP, prd-like, LIM, and POU also supports this assignment but is not statistically significant (supplementary fig. S21, Supplementary Material online). The last Capsaspora non-TALE homeobox gene has a C-terminal TRAM LAG1 CLN8 (TLC) domain and a transmembrane domain, the characteristic domain architecture of the lass (longevity assurance homologs of yeast [Lag-1]) genes, which are considered to be homologs to fungal Lag genes. Interestingly, phylogenetic analysis of the TLC domain showed that LAG genes with homeodomain are exclusive to metazoans and Capsaspora, whereas genes with the TLC domains and TRAM1 domain are found in amoebozoans, fungi, and metazoans (supplementary fig. S22, Supplementary Material online). Lass genes, however, are implicated in ceramide synthesis, the function of their homeodomain being unclear and their specific TF activity unknown (Teufel et al. 2009). Our data show that the repertoire of homeobox genes in metazoan unicellular relatives is larger than previously thought (see fig. 1), however, some specific homeobox gene classes, such as ANTP appear to be exclusive to the Metazoa. Genome data from additional unicellular relatives of metazoans will be needed to corroborate this.
CBP/p300
The CBP/p300 is a ubiquitous metazoan transcriptional coactivator that interacts with several TFs, acts as an acetyltransferase (Coutts and La Thangue 2005) and is involved in cell growth and development (Goodman and Smolik 2000). Specifically, CBP/p300 interacts with such TFs such as NF-kappaB (Perkins et al. 1997), Stat (Levy and Darnell 2002; Wojciak et al. 2009), Runx (Jin et al. 2004; Makita et al. 2008), p53 (Grossman 2001), CREB (Manna et al. 2009), and C/EBP (Manna et al. 2009). For example, CBP/p300 acetylates Runx genes (Jin et al. 2004) and ubiquitinates p53 (Shi et al. 2009). We have identified CBP/p300 homologs in both Capsaspora and M. brevicollis. This implies that CBP/p300 originated prior to the divergence of Capsaspora from choanoflagellates and metazoans. It is worth mentioning that this multifunctional cofactor seems to have evolved concomitant to the emergence of several holozoan TFs, such as Runx and NF-kappaB. This suggests that a relatively high level of regulatory complexity was already emerging on early in the holozoan lineage, well before the divergence of metazoan and choanoflagellate lineages.
LSF/GRH
The LSF/GRH family of TFs is characterized by the CP2 domain, and its members play important roles in bilaterians, being involved in vertebrate organogenesis, cell cycle progression, and cell survival and differentiation (Bray and Kafatos 1991; Uv et al. 1997; Veljkovic and Hansen 2004; Traylor-Knowles et al. 2010). LSF/GRH can be divided into two groups, the LSF/CP2 and the GRH subfamilies (Shirra and Hansen 1998; Traylor-Knowles et al. 2010). Members of the LSF/CP2 subfamily act as tetramers and possess an extra SAM domain C-terminal to the specific CP2 DNA-binding domain. Members of the GRH subfamily do not have the SAM domain and act as dimers.
The CP2 domain is present throughout the opisthokonts, including choanoflagellates (Traylor-Knowles et al. 2010) and seems to be a synapomorphy of this group of eukaryotes. Interestingly, it has been hypothesized that the GRH subfamily originated by duplication of an ancestral LSF/GRH-like gene at the origin of the Metazoa and was coopted to epidermal determination in metazoans (Traylor-Knowles et al. 2010). We identified two LSF/GRH genes in Capsaspora, a LSF-like and a GRH-like (fig. 1 and supplementary fig. S23, Supplementary Material online), although the Capaspora LSF-like gene lacks the characteristic C-terminal SAM domain found in metazoan LSF proteins. This domain may have been gained by domain shuffling before the split between metazoans and choanoflagellates, although the loss of this domain in Capsaspora cannot be ruled out. Our findings imply that the duplication of the LSF/GRH gene occurred before Capsaspora diversified from choanoflagellates and metazoans, and that GRH was lost in choanoflagellates. Thus, the presence of a GRH gene antedates the origin of the metazoan epithelium.
NRs, Smad, and Ets
Our data show that these three TFs families remain, at this time, metazoan specific because we did not identify any homologs in nonmetazoan taxa. The complete genome sequences of additional nonmetazoan taxa are needed to corroborate this hypothesis.
Origin and Early Evolution of Metazoan TFs
The repertoire of TFs in the holozoan C. owczarzaki reported here, and its comparison with metazoan, fungal, and choanoflagellate TFs provides important insights into the origin and evolution of TFs that are essential for metazoan multicellularity. This allows us to propose a new hypothesis regarding the origin of key metazoan TFs (see fig. 5). Some metazoan TF domains have deep origins being widespread in eukaryotes, such as HMG box, homeodomain (both TALE and non-TALE), bHLH, bZIP, or Mef2-like (see also Degnan et al. 2009). However, major diversifications of genes encoding some of these domains took place along metazoan and fungal stems (see fig. 1), generating lineage-specific classes and subfamilies. In regards to metazoan-specific TF gene families, there appears to have been two major expansions (fig. 5): one prior to the divergence of Capsaspora, choanoflagellates, and the Metazoa (e.g., in bZIP and bHLH) and another within the metazoan lineage (such as Sox, homeodomains, and further diversification of bZIP and bHLH). Several other TF domains, such as Churchill, STAT, and, most likely, Fox, were already present in the common ancestor of unikonts (i.e., amoebozoans, apusozoans, and opisthokonts). This finding changes previous views in which Churchill was considered exclusive to metazoans and Fox exclusive to opisthokonts (although the assignment of A. castellanii hits to Fox remain contentious). Although STAT domains were present in the common ancestor of unikonts, our data show that canonical metazoan-type STAT seem to be exclusive to apusozoans (T. trahens) and opisthokonts. A major challenge to previous proposals that T-box genes are metazoan innovations is the discovery of T-box genes in S. punctatus and Capsaspora. This means that T-box genes appeared before the divergence of fungi and holozoans. What role are these T-box genes playing in these nonmetazoan lineages remains to be studied.
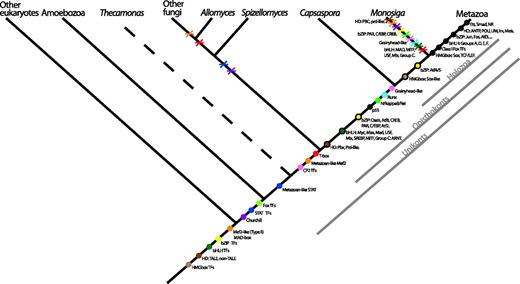
Cladogram representing TF evolution among the analyzed taxa. Colors are unique for each domain class. A colored dot means the hypothetical origin of the domain. A black-circled dot indicates where a specific protein family appears in our taxon sampling. A cross means the loss of the domain or specific protein family in a lineage. Metazoan apomorphies are shown as black dots. The phylogenetic relationships are based on several recent studies (Burki et al. 2008; Ruiz-Trillo et al. 2008; Brown et al. 2009; Liu et al. 2009; Minge et al. 2009).
Interestingly, some TFs appear to have evolved prior to the divergence of Capsaspora from choanoflagellates and metazoans, such as p53, Runx, and NF-kappaB; the latter two previously being considered metazoan specific. This pattern of gene families that are relevant to metazoan multicellularity evolving prior to the emergence of the metazoan stem lineage is not new and has been observed in other cases, such as tyrosine kinases, cadherins, MAGUKs, and integrins (Abedin and King 2008; King et al. 2008; Manning et al. 2008; Suga et al. 2008; Degnan et al. 2009; de Mendoza et al. 2010; Sebe-Pedros et al. 2010). Finally, there are some TF domains that, under the current taxon sampling, appear to be metazoan innovations. These are ETS, Smad, and NRs. Moreover, some specific homeobox genes (ANTP, LIM, POU, Irx, Meis, Tgif, Six), bZIP classes (e.g., Jun, Fos), bHLH classes (A, D–F groups), and HMG box classes (Sox, TCL/lef) appear also to be metazoan specific (fig. 5), although we cannot rule out the possibility that some of these may have a more ancient origin and secondarily lost in nonmetazoan lineages. This new evolutionary scenario implies that significant lineage-specific TFs losses occurred within the choanoflagellate lineage. For example, Runx, T-box, RHD domain, GRH-like, and Churchill appear to have been lost in M. brevicollis. Whether this is specific to one choanoflagellate lineage (that of M. brevicollis) or to choanoflagellates in general remains unknown. Only genomic data from additional choanoflagellate taxa will resolve this issue. A similar pattern of lineage-specific loss in choanoflagellates has recently been shown for the integrin-mediated adhesion machinery (Sebe-Pedros et al. 2010).
A quantitative analysis (fig. 1) of TFs evolution suggests that several expansions occurred in Eumetazoa, such as bHLH and homeobox gene families and to a lesser degree HMG box and bZIP families. Specific domain expansions have already been reported in the Viridiplantae for bHLH and homeodomain proteins (Mukherjee et al. 2009; Pires and Dolan 2010). There are several theories about the correlation of these expansions with the transition to multicellularity (Derelle et al. 2007; Pires and Dolan 2010). On the other hand, some TF domains, such as CP2, Runt, MADSbox, Churchill, p53, and STAT, have similar number of members in unicellular and multicellular holozoans. Capsaspora TF complexity is quite high, with a wider range of bHLH and bZIP domain-containing proteins than in some early-branching metazoans such as A. queenslandica or T. adhaerens. Because Capsaspora has a complex (and not fully understood) life cycle, in which there is a symbiotic stage within the mollusc Biomphalaria glabrata, one may wonder whether the complexity of TFs identified in Capsaspora is due to LGT from the host or even from the trematode flatworm S. mansoni, a metazoan parasite of B. glabrata. Based on our phylogenetic analyses, we do not favor this hypothesis. None of the phylogenetic trees shown (all including bilaterians; some even B. glabrata homologs) show the Capsaspora homolog grouping closer to bilaterians than to other metazoans. Instead, we hypothesize that the common ancestor of Capsaspora, choanoflagellates, and metazoans had a richer TF repertoire than previously believed and that some TFs were subsequently lost in the choanoflagellate lineage (or at least in M. brevicollis).
In summary, our results show that the evolution of metazoan TFs includes the acquisition of new genes (some of them via domain shuffling), gene cooption, and the diversification of ancestral domains increasing the combinatorial complexity. How these metazoan developmental TFs are functioning in unicellular organisms and how they were exapted into new functions in multicellular animals remains to be answered.
The genome sequences of C. owczarzaki, A. macrogynus, S. punctatus, and T. trahens are being determined by the Broad Institute of MIT/Harvard University under the auspices of the National Human Genome Research Institute and within the UNICORN initiative. We thank JGI, BI, and BCM for making data publicly available. We thank Kim C. Worley and the team of the A. castellanii genome project for accession to the genome data. We thank Peter W. H. Holland for help in the assignment of homeodomain-containing proteins and Romain Derelle, Jordi Paps, and Hiroshi Suga for helpful insights. This work was supported by an ICREA contract, an European Research Council Starting Grant (ERC-2007-StG-206883), and a grant (BFU2008-02839/BMC) from Ministerio de Ciencia e Innovación (MICINN) to I.R.-T. A.S.’s salary was supported by a pregraduate FPU grant and A.d.M.’s salary from a FPI grant both from MICINN. B.F.L.’s and B.M.D.’s contributions were supported by the Canadian Research Chair Program and the Australian Research Council, respectively.
References
Author notes
These authors contributed equally to this work.
Associate editor: Billie Swalla