-
PDF
- Split View
-
Views
-
Cite
Cite
James Haile, Richard Holdaway, Karen Oliver, Michael Bunce, M. Thomas P. Gilbert, Rasmus Nielsen, Kasper Munch, Simon Y. W. Ho, Beth Shapiro, Eske Willerslev, Ancient DNA Chronology within Sediment Deposits: Are Paleobiological Reconstructions Possible and Is DNA Leaching a Factor?, Molecular Biology and Evolution, Volume 24, Issue 4, April 2007, Pages 982–989, https://doi.org/10.1093/molbev/msm016
- Share Icon Share
Abstract
In recent years, several studies have reported the successful extraction of ancient DNA (aDNA) from both frozen and nonfrozen sediments (even in the absence of macrofossils) in order to obtain genetic “profiles” from past environments. One of the hazards associated with this approach, particularly in nonfrozen environments, is the potential for vertical migration of aDNA across strata. To assess the extent of this problem, we extracted aDNA from sediments up to 3300 years old at 2 cave sites in the North Island of New Zealand. These sites are ideal for this purpose as the presence or absence of DNA from nonindigenous fauna (such as sheep) in sediments deposited prior to European settlement can serve as an indicator of DNA movement. Additionally, these strata are well defined and dated. DNA from sheep was found in strata that also contained moa DNA, indicating that genetic material had migrated downwards. Quantitative polymerase chain reaction analyses demonstrated that the amount of sheep DNA decreased as the age of sediments increased. Our results suggest that sedimentary aDNA is unlikely to be deposited from wind-borne DNA and that physical remains of organisms or their ejecta need to have been incorporated in the sediments for their DNA to be detected. Our study indicates that DNA from sediments can still offer a rich source of information on past environments, provided that the risk from vertical migration can be controlled for.
Introduction
Ancient DNA (aDNA) from diverse mammals and plants has been obtained directly from minor amounts of permafrost (permanently frozen) sediments many thousands of years old (Willerslev et al. 2003; Lydolph et al. 2005). Likewise, under nonfrozen conditions, trace amounts of sediment have yielded aDNA sequences of diverse vertebrate and plant species, even in the absence of macrofossils (Hofreiter et al. 2003; Willerslev et al. 2003). The immediate sources of this DNA is unclear. A possible source of plant DNA in sedimentary deposits is fine rootlets (Willerslev et al. 2003). A variety of sources have been suggested for animal DNA, including dung, urine, skin, hair, and keratin (Lydolph et al. 2005). Also uncertain is whether the DNA is extracellular and bound to clay minerals or if cellular DNA is released during the extraction procedure (Ogram et al. 1988).
To date, most aDNA sedimentary analyses have examined soil profiles from permafrost regions (Hansen et al. 2001; Willerslev et al. 2003; Willerslev, Hansen, Poinar 2004; Willerslev, Hansen, Rønn, et al. 2004; Lydolph et al. 2005; Mitchell et al. 2005). In this environment, 2 sources of evidence suggest that DNA leaching and redeposition are not significant problems: firstly, changes observed in floral and faunal communities through time agree broadly with those predicted by macrofossil records (Willerslev et al. 2003; Lydolph et al. 2005); secondly, the recovered DNA fragments were damaged in clear age-dependent patterns, despite discontinuous sediment chronology and the presence of free water (Willerslev, Hansen, Poinar 2004; Hansen et al. 2006). These results are encouraging, but the potential for DNA being leached in nonfrozen conditions remains to be examined (Pääbo et al. 2004). This is especially important because temperate and desert cave sites are major sources of aDNA used in reconstructing past environments (Poinar et al. 1996,1998; Hofreiter et al. 2000, 2003; Willerslev et al. 2003). DNA leaching would significantly complicate, or even invalidate, the interpretation of results in some contexts (Poinar et al. 1996, 1998; Hofreiter et al. 2000). If the fidelity of DNA sequences can be established from strata (of defined age), however, then this technology will enable paleofaunal reconstructions spanning thousands of years (Willerslev and Cooper 2005). Apart from leaching, other potential sources of bias in taxon representation include taxon-dependent factors such as body size and the likely presence of remains, such as hair, feathers, eggs, and dung.
In this study, we used sediments from 2 cave sites in New Zealand to gain insights to the origin of sedimentary aDNA and to test for DNA leaching in nonfrozen sediments. New Zealand has an environment ideal for investigating possible DNA leaching because the preservation of bones and other materials is excellent. Most importantly, New Zealand had a limited range of large vertebrates, mostly birds, prior to the arrival of humans; terrestrial mammals were entirely absent, with the exception of 3 bat species. This distinctive faunal composition allows leaching to be readily identified, because the presence of nonindigenous mammal species in presettlement strata is necessarily the result of downward movement of DNA in the sediments. For example, sheep (Ovis aries), whose numbers currently exceed 40 million, were introduced to New Zealand by European settlers only from the 1830s; they did not reach the study area until approximately 1870. It is also well established that the large, indigenous, herbivorous ratite birds known as moa (Aves; Dinornithiformes) became extinct by approximately 550 years ago (Holdaway and Jacomb 2000), and so the presence of moa DNA in European layers would imply upward movement of sedimentary DNA or of the sediment constituents that retained the DNA. These and other distinct changes in the biota provide an opportunity to assess the extent of DNA leaching within nonpermafrost sediments.
Materials and Methods
Sediment samples were taken from freshly excavated sections in 2 dry caves located on the southern face of the Hukanui range, North Island, New Zealand (fig. 1): Hukanui Pool and Hukanui #7a (fig. 2) are approximately 300 m apart and at 860 and 800 m above sea level, respectively (Holdaway and Beavan-Athfield 1999). The sites are beneath large erratic limestone blocks and both contain sediment layers ranging in age from >3000 years old to the present. Importantly, the Hukanui locality itself is well suited for investigating paleogenetic reconstructions because it contains 2 layers of volcanic ejecta from massive eruptions from the Lake Taupo volcano (fig. 1); the Taupo ignimbrite deposited at AD 232 ± 15 (Sparks et al. 1995) and the Waimihia tephra (deposited at 3280 ± 20 yr 14C years before present (Froggatt and Lowe 1990).
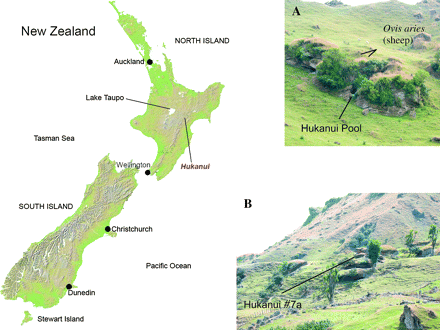
Map of New Zealand, showing location of Hukanui sites: (A) General view of rock pile containing Hukanui Pool site, (B) General view of rock pile containing Hukanui #7a site.
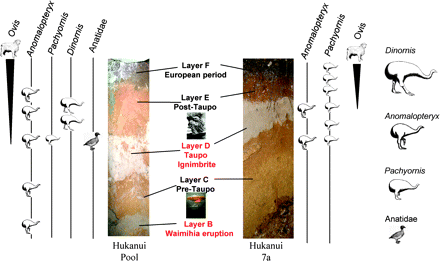
Stratigraphic sections in Hukanui Pool and Hukanui #7a sites showing sampling sites and strata. The Hukanui #7a section is viewed into a corner of an excavation square, and the slope of the interfaces is exaggerated. Note the irregular interface at the base of the Taupo ignimbrite reflecting the disturbance of the sediment surface by the incoming tephra.
The Taupo eruption emplaced the Taupo ignimbrite from a pyroclastic flow, which traveled the 80 km to the sites at high speed and at a relatively high temperature, destroying all vegetation and fauna in its path. It disturbed the sediment surfaces and entrained bones and sediment particles in the sites and now forms a tephra layer up to 600 mm thick, with charcoal, sediment, and (rare) bone inclusions. The Taupo ignimbrite has the consistency of bulk cement and can also support vertical sections; all disturbances in the layers are easily visible.
By contrast, the Waimihia tephra (Froggatt and Lowe 1990) was deposited as a “rain” of pumice lapilli approximately 1–2 mm in diameter, which stripped leaves from trees but did not necessarily kill them. The tephra formed a layer approximately 100 mm thick (Froggatt and Lowe 1990) in the Hukanui Pool site, which was more open, but did not enter Hukanui #7a in any significant amounts.
The sediments in Hukanui Pool are more or less horizontal over most of the floor, but the layers slope downwards toward the western wall. In Hukanui #7a, sediments were incorporated both as horizontal fill amongst and above boulder debris and as a small fan in the northwestern corner of the excavation, where the aDNA samples were collected. The fan had been formed from sediments entering through a small opening from an upper chamber (Holdaway et al. 2002).
Additionally, the sharp, sand-sized particles of fossil barnacle shells and other marine organisms (derived from the enclosing limestone rocks) that compose much of the cave sediments contain variable amounts of clay. The clay binds the sediments, making them firm and cohesive and lending stability to vertical sections, so that macroscopic particles cannot migrate through or between the layers. Any DNA found out of stratigraphic context must therefore have migrated as free molecules or bound to microscopic particles. Significantly, there are no rooted plants or soil horizon development in the sediments of either site, and so they are not soils.
The sediments were moist as a result of condensation in a humid environment, but there is no fall of water onto the surface nor flow across it. These are rock shelters, not true water-carved caves, and there is no free water flowing in the sites (Holdaway and Beavan-Athfield 1999). Both sites are just a few meters from the open air and the sediments grade continuously into the outside soil.
Sampling, DNA Extraction, and Amplification
Contamination with extraneous DNA is an ever-present concern in any aDNA study, and it is the responsibility of the researcher to demonstrate that adequate experimental and authentication procedures are carried out (Cooper and Poinar 2000; Gilbert et al. 2005). Samples were taken from freshly excavated sections in the 2 shelters, beginning at the bottom of each section and proceeding to more recent levels (fig. 2). Disposable tools were used and changed between samples to avoid cross-contamination. All manipulation of ancient samples before polymerase chain reaction (PCR) amplification were performed in dedicated aDNA laboratories at the Henry Wellcome Ancient Biomolecules Centre at the University of Oxford and the Centre for Ancient Genetics at the University of Copenhagen, in areas free from other molecular research. One negative extraction and 1 amplification control was used for every 8 samples extracted, and each positive results cloned a minimum of 8 times in agreement with suggested aDNA criteria (Handt et al. 1994; Willerslev et al. 1999; Hansen et al. 2001; Gilbert et al. 2003, 2005; Willerslev and Cooper 2005).
DNA from a total of 1 g of wet weight sediment per sample was extracted in 2 subsamples of 0.5 g sediment, dissolved in 600 μl lysis buffer (Bulat et al. 2000) 400 μg/ml proteinase K (Roche Applied Science, Mannheim, Germany) disrupted with 4 runs of a FastPrep 120 (BIO 101) at speed 6.5 for 45 s, with 2 min on ice between runs and incubated at 65 °C for at least 4 h under agitation. The solution was adjusted with NaCl to 1.15 M, treated with 1/2 volume of chloroform/octanol (24:1), and agitated slowly overnight at room temperature, and the water phase isolated with centrifugation at 12,000 g for 2 min and transferred to a separate microtube for incubation at 2–3 °C for at least 1 h. The precipitate was centrifuged at 12,000 g for 2 min and the supernatant purified using silica spin columns and binding buffer (Qiagen DNA purification kit II), followed by washes in 0.5 ml Salton wash 1 and 2 (BIO 101) and 0.5 ml AW1 and AW2 (Qiagen tissue kit). The DNA was eluted twice with 100 μl elution buffer (Qiagen purification kit II) and stored at −20 °C.
PCR was used to amplify an 88 bp (moa) and 60 bp (sheep) fragment of control region mitochondrial DNA (mtDNA), avian 153 bp fragment of 12S mtDNA, and plant rbcL and trnL chloroplast DNA using primers listed in table S1 (Supplementary Material online), using 5 μl of DNA extractions, 35–55 cycles of PCR (1.5 min initial denaturation at 94 °C, 45 s at 94 °C, 45 s at 45–60 °C, 1.5 min at 68 °C, and a final cycle of 10 min at 68 °C). PCR products were cleaned using a QIAquick PCR Purification Kit (Qiagen, Crawley, UK). Amplification products from the 2 separate extracts of each sample were pooled, cloned, purified, and sequenced on both strands (Willerslev et al. 1999). Sequences were aligned using ClustalW in BioEdit (Hall 1999) and possible recombination among the clone sequences investigated (Willerslev et al. 1999).
Quantitative Real-Time PCR (qPCR)
A SYBRGreen based qPCR assay was used to determine the relative quantity of sheep DNA within the Hukanui Pool DNA extracts. Amplifications targeted a 71 bp fragment of the sheep mtDNA control region, using qPCR primers Sheep-87F and Sheep-157R (table S1, Supplementary Material online). Before the qPCR analysis, the primers were prescreened on both sheep DNA-positive and -negative soil extracts and blanks, to ensure that they generated a single correct product, with no primer–dimer or nonspecific products that might contribute to erroneous results. The qPCR assay itself was performed in 25 μl reaction volumes, using dilution series of the Hukanui Pool extracts (1 μl, 0.5 μl, 0.25 μl, and 0.125 μl concentration) to ensure replicability of the results and to screen for any inhibitory effects that might complicate interpretation of the results. The reaction contained 300 μM (final concentration) of each primer, used SYBRGreen PCR Mastermix (Applied Biosystems, Warrington, UK), and was performed using an ABI PRISM 7000 instrument (ABI). A dissociation curve was generated at the end of the reaction, to ensure that measured fluorescence was attributable only to the correct amplification product (through assessment of fragment melting temperature). As a further control the amplified products were analyzed using conventional agarose gel electrophoresis to ensure the correct size of the amplified product (table S2, Supplementary Material online).
Sequence Identification
Sequences were assigned to species using a Bayesian approach that allows calculation of probabilities of membership of specific taxonomic groups. This automated procedure combines database searches with alignment algorithms and Bayesian phylogenetic procedures, using information from National Center for Biotechnology Information's taxonomy browser (Benson et al. 2000; Wheeler et al. 2000). First, using database searches, we identified sets of closely related sequences to each query sequence. The 50 best hits defined by E values of a Blast search were chosen, eliminating identical copies and requiring at least 3 families and 2 orders to be sampled for each query sequence. An alignment of the 50 nonredundant sequences was then produced using ClustalW (Thompson et al. 1994). Flanking regions up to 200 bp were included, if available, from the database sequences.
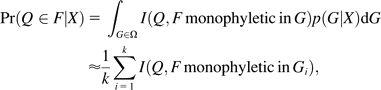
In the vast majority of cases, the query sequences could be assigned to particular species with very high posterior probability (PP > 0.9). The full results of the analyses are available at http://www.binf.ku.dk/∼kasper/pipeline/sediment_deposits/.
Results and Discussion
Avian DNA
Avian DNA was successfully amplified from the layers shown in figure 2 and listed in table 1. The absence of moa DNA from Layer F in Hukanui Pool, the youngest layer in that site, is expected because moa have been extinct in New Zealand for at least 500 years, and this result confirms that upward movement of the DNA in this site is not a significant problem. The presence of amplifiable moa DNA in Layer F in Hukanui #7a is not surprising, however, because the layer had been affected by an earlier (1959) excavation that reached the top of the Taupo ignimbrite (Layer D) over part of the site, and sediment from pre-European levels elsewhere in the shelter had been displaced and mixed with the European layer materials at the sampling point. In addition, the sample section was beneath the opening to an upper chamber in the site complex (Holdaway et al. 2002), through which sediment and other materials have entered. At the lowest, oldest stratigraphic levels, amplifiable moa DNA was absent from Layer A in Hukanui Pool and Layers A and B in Hukanui #7a; this could be the result of either degradation or limited levels of occupation at that time in each site, or both.
Stratigraphic Distribution of Moa and Other Bird Taxa in Hukanui Pool and Hukanui #7a
Strata | Sample Number | Primers Moa CR 262F, 329R | Sample Number | Primers 12SE, 12SH |
Hukanui Pool | ||||
F European | Layer F | No products | No products | |
E Post-Taupo | Layer E-25 | Anomalopteryx (93%) | Anomalopteryx (100%) | |
E Post-Taupo | Layer E-150 | Anomalopteryx (97%) | Layer E-150 | Anomalopteryx (97%) |
Dinornis (79%) | ||||
E Post-Taupo | Layer E-290 | Dinornis (81%)a | ||
D Taupo | Layer D-20 | Anomalopteryx (98%) Pachyornis (99%) Pachyornis (100%)a | Layer D-20 | Anomalopteryx (98%) Anatidae (100%) |
C Pre-Taupo | Layer C-10 | No products | Layer C-10 | Anomalopteryx (97%) |
B Waimihia | Layer B-10 | Anomalopteryx (92%) | Layer B-10 | Dinornis (52%) |
A Pre-Waimihia | No products | No products | ||
Hukanui #7a | ||||
F European | Layer F | Pachyornis (100%)a | No products | |
E Post-Taupo | Layer E-10 | Pachyornis (100%)a | Layer E-10 | No products |
E Post-Taupo | Layer E-80 | Pachyornis (91%) | Layer E-80 | No products |
D Taupo | Layer D-100 | Pachyornis (100%) | Layer D-80 | Anomalopteryx (96%) |
C Pre-Taupo | Layer C-10 | Pachyornis (95%) | Layer C-10 | |
Layer C-80 | Pachyornis (100%) | Layer C-80 | Anomalopteryx (98%) | |
B Waimihia | Not present | Not present | ||
A Pre-Waimihia | Not present | Not present |
Strata | Sample Number | Primers Moa CR 262F, 329R | Sample Number | Primers 12SE, 12SH |
Hukanui Pool | ||||
F European | Layer F | No products | No products | |
E Post-Taupo | Layer E-25 | Anomalopteryx (93%) | Anomalopteryx (100%) | |
E Post-Taupo | Layer E-150 | Anomalopteryx (97%) | Layer E-150 | Anomalopteryx (97%) |
Dinornis (79%) | ||||
E Post-Taupo | Layer E-290 | Dinornis (81%)a | ||
D Taupo | Layer D-20 | Anomalopteryx (98%) Pachyornis (99%) Pachyornis (100%)a | Layer D-20 | Anomalopteryx (98%) Anatidae (100%) |
C Pre-Taupo | Layer C-10 | No products | Layer C-10 | Anomalopteryx (97%) |
B Waimihia | Layer B-10 | Anomalopteryx (92%) | Layer B-10 | Dinornis (52%) |
A Pre-Waimihia | No products | No products | ||
Hukanui #7a | ||||
F European | Layer F | Pachyornis (100%)a | No products | |
E Post-Taupo | Layer E-10 | Pachyornis (100%)a | Layer E-10 | No products |
E Post-Taupo | Layer E-80 | Pachyornis (91%) | Layer E-80 | No products |
D Taupo | Layer D-100 | Pachyornis (100%) | Layer D-80 | Anomalopteryx (96%) |
C Pre-Taupo | Layer C-10 | Pachyornis (95%) | Layer C-10 | |
Layer C-80 | Pachyornis (100%) | Layer C-80 | Anomalopteryx (98%) | |
B Waimihia | Not present | Not present | ||
A Pre-Waimihia | Not present | Not present |
NOTE.—Numbers in brackets represent the highest posterior probabilities overobtained for that genus (or for Anatidae, for that family). Layer numbers represent distance in mm below strata interface (e.g., Layer B-10 refers to sample in Layer B 10 mm below B/C boundary).
Independently produced results.
Stratigraphic Distribution of Moa and Other Bird Taxa in Hukanui Pool and Hukanui #7a
Strata | Sample Number | Primers Moa CR 262F, 329R | Sample Number | Primers 12SE, 12SH |
Hukanui Pool | ||||
F European | Layer F | No products | No products | |
E Post-Taupo | Layer E-25 | Anomalopteryx (93%) | Anomalopteryx (100%) | |
E Post-Taupo | Layer E-150 | Anomalopteryx (97%) | Layer E-150 | Anomalopteryx (97%) |
Dinornis (79%) | ||||
E Post-Taupo | Layer E-290 | Dinornis (81%)a | ||
D Taupo | Layer D-20 | Anomalopteryx (98%) Pachyornis (99%) Pachyornis (100%)a | Layer D-20 | Anomalopteryx (98%) Anatidae (100%) |
C Pre-Taupo | Layer C-10 | No products | Layer C-10 | Anomalopteryx (97%) |
B Waimihia | Layer B-10 | Anomalopteryx (92%) | Layer B-10 | Dinornis (52%) |
A Pre-Waimihia | No products | No products | ||
Hukanui #7a | ||||
F European | Layer F | Pachyornis (100%)a | No products | |
E Post-Taupo | Layer E-10 | Pachyornis (100%)a | Layer E-10 | No products |
E Post-Taupo | Layer E-80 | Pachyornis (91%) | Layer E-80 | No products |
D Taupo | Layer D-100 | Pachyornis (100%) | Layer D-80 | Anomalopteryx (96%) |
C Pre-Taupo | Layer C-10 | Pachyornis (95%) | Layer C-10 | |
Layer C-80 | Pachyornis (100%) | Layer C-80 | Anomalopteryx (98%) | |
B Waimihia | Not present | Not present | ||
A Pre-Waimihia | Not present | Not present |
Strata | Sample Number | Primers Moa CR 262F, 329R | Sample Number | Primers 12SE, 12SH |
Hukanui Pool | ||||
F European | Layer F | No products | No products | |
E Post-Taupo | Layer E-25 | Anomalopteryx (93%) | Anomalopteryx (100%) | |
E Post-Taupo | Layer E-150 | Anomalopteryx (97%) | Layer E-150 | Anomalopteryx (97%) |
Dinornis (79%) | ||||
E Post-Taupo | Layer E-290 | Dinornis (81%)a | ||
D Taupo | Layer D-20 | Anomalopteryx (98%) Pachyornis (99%) Pachyornis (100%)a | Layer D-20 | Anomalopteryx (98%) Anatidae (100%) |
C Pre-Taupo | Layer C-10 | No products | Layer C-10 | Anomalopteryx (97%) |
B Waimihia | Layer B-10 | Anomalopteryx (92%) | Layer B-10 | Dinornis (52%) |
A Pre-Waimihia | No products | No products | ||
Hukanui #7a | ||||
F European | Layer F | Pachyornis (100%)a | No products | |
E Post-Taupo | Layer E-10 | Pachyornis (100%)a | Layer E-10 | No products |
E Post-Taupo | Layer E-80 | Pachyornis (91%) | Layer E-80 | No products |
D Taupo | Layer D-100 | Pachyornis (100%) | Layer D-80 | Anomalopteryx (96%) |
C Pre-Taupo | Layer C-10 | Pachyornis (95%) | Layer C-10 | |
Layer C-80 | Pachyornis (100%) | Layer C-80 | Anomalopteryx (98%) | |
B Waimihia | Not present | Not present | ||
A Pre-Waimihia | Not present | Not present |
NOTE.—Numbers in brackets represent the highest posterior probabilities overobtained for that genus (or for Anatidae, for that family). Layer numbers represent distance in mm below strata interface (e.g., Layer B-10 refers to sample in Layer B 10 mm below B/C boundary).
Independently produced results.
The absence of moa DNA from the youngest and oldest intact layers, and the restriction of certain moa genera to specific strata (table 1), suggest that vertical movement of avian DNA is not a significant factor in these sites, thereby permitting an environmental interpretation of the results.
The pattern of occurrence of moa taxa in Layers B–E of the 2 sites (table 1) appears to be related to the physical constraints of both study sites (fig. 3), the vegetation surrounding the sites, and possibly to interactions between the moa taxa that used the sites for nesting. The lower chamber of Hukanui #7a has always had a very low ceiling (Holdaway et al. 2002), and bones (Worthy and Holdaway 2000) and DNA (table 1) of only the 2 smaller taxa were found in the sediments. However, although their macroscopic remains and DNA have not been identified yet in the deposit, very small juvenile Dinornis could have entered both the upper and lower chambers. Although Hukanui Pool was always large enough for all 3 taxa to enter (fig. 3), only the 2 larger taxa are represented there by macrofossils (Holdaway RN, unpublished data). DNA of all 2 taxa was identified from Hukanui Pool (table 1), but DNA of the smallest moa (Pachyornis) was recorded only from near the surface of Layer D (Taupo ignimbrite). The apparent absence, except for 1 brief period, of Pachyornis from Hukanui Pool, which it physically could have entered (fig. 3), may be explained by factors, such as habitat preference, environmental disturbance resulting from the most recent Taupo eruption, and interaction between the species.
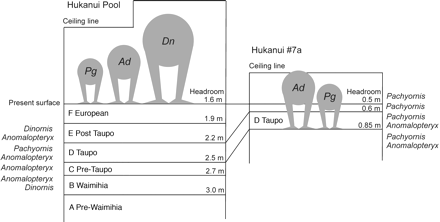
Relative size of moa species and the different sediment levels at both sites. Pg, Pachyornis; Ad, Anomalopteryx; and Dn, Dinornis.
The Taupo ignimbrite completely devegetated the slopes around the sites during its emplacement (Worthy and Holdaway 2000). The DNA evidence suggests that Pachyornis used the Hukanui Pool site only for a brief period following this eruption, when the surrounding vegetation would have been recovering via stages including seral shrubland. If, shrubland did not provide suitable habitat for the 2 larger moa taxa then, they would not have been present to exclude the small Pachyornis. Consequently, Pachyornis could have occupied the site (as it continued to do downslope in Hukanui #7a) until the forest developed sufficiently for the larger taxa to recolonize the area. If the larger moa had indeed excluded Pachyornis from Hukanui Pool, it would imply competition for secure nest sites based on body size. The large (c. 1.5–2 kg) extinct harrier (Circus sp.), whose own bones and prey remains were present in both sites (Worthy and Holdaway 2000), could well have preyed on the smaller moa and led to the latter's preference for enclosed nest sites. There is some evidence for harrier predation on small moa in the form of characteristic damage to at least 1 pelvis from Hukanui #7a (Holdaway RN, unpublished data).
The absence of Dinornis DNA from all sediments in Hukanui #7a, a site from which its larger juveniles and adults would have been physically excluded (fig. 3), and of Pachyornis from all but 1 level in Hukanui Pool, even though both species were present in the area, suggests that the sites record for the most part only those taxa that either physically entered the sites, alive or dead. Occasional deaths of very young Dinornis in Hukanui #7a could complicate the record, but do not appear to have done so. Hence, interpretation of presence–absence data based on DNA preserved in sediments has to take into account the physical and biological contexts.
Four species of moa have been identified from skeletal remains in the area of the sites: Dinornis (80–120 kg live mass), Euryapteryx gravis (40–70 kg), Anomalopteryx didiformis (30–40 kg), and Pachyornis geranoides (10–15 kg). Two of these genera (Dinornis and Anomalopteryx) are believed to have been associated with tall forest, although Dinornis was found also in areas with more open vegetation (Worthy and Holdaway 1993; Worthy and Swabey 2002). Pachyornis geranoides probably preferred wetland vegetation (Worthy and Holdaway 2002: 196), the shrubland ecotones provided by wetlands or the productive forest edge (Worthy 1990). There were no wetlands near the 2 sites, so the species seems also to have been able to occupy forest with an understorey of smaller angiosperms, which was the normal vegetation around the sites during the Holocene (Worthy and Holdaway 2000).
The mtDNA of Pachyornis identified in a sample (E-10) taken 10 mm below the “pre-European” surface in Hukanui #7a and of Anomalopteryx in a sample taken 25 mm below the “pre-European surface” in Hukanui Pool (sample E-25) are the stratigraphically highest occurrences of DNA of any extinct taxa in intact sediments in the 2 sites. Allowing for some disturbance of the floor of the shelter by the birds themselves, these occurrences represent the last stages of the presence of moa before their extinction in the area. Some deflation or erosion of the surface in each site may have occurred after deposition of the E-10 and E-25 material, but it is also possible that the sedimentation regime altered with the deforestation that accompanied Polynesian settlement of the area, at about the time of moa extinction. As noted above, the presence of Pachyornis DNA in Layer F of Hukanui #7a can be attributed to the mixing of debris from older layers with post-European debris during earlier excavations, and there is direct evidence that moa material and sediment originally emplaced in lower strata were mechanically transported by burrowing.
The lack of amplifiable avian DNA from the deep European layer in Hukanui Pool, where no previous excavations had disturbed the main deposit, indicates that birds have not inhabited the rock shelters in modern times, perhaps with the exception of occasional visits by foraging songbirds. It is perhaps more surprising that only 2 nonmoa avian DNA sequences have amplified from New Zealand sediments, that of ducks (Anatidae, Hukanui Pool) and a parakeet at another site (Willerslev et al. 2003). However, the recovery of significant amounts of moa eggshells and bones from the Hukanui sites indicate that these were used by moa for nesting, so it is possible that the sheer volume of moa DNA has swamped that of any other species. Bones of nonmoa taxa were rare and thinly distributed in Hukanui Pool (Holdaway RN, unpublished data). Hukanui #7a, however, contained a rich deposit of bones (Worthy and Holdaway 2000; Holdaway et al. 2002) but these were concentrated 1–2 m from where the DNA sediment samples were taken later. The data indicate that relative biomass may need to be taken into account when using nonspecific primers to retrieve different elements of past ecosystems. Alternatively, it may be necessary to employ primers that preferentially amplify less common DNA. The Hukanui #7a results suggest also that more than 1 chronoseries of samples may be required to explore fully the diversity of DNA preserved in even a small site.
The Waimihia tephra is a volcanic airfall deposit, so it is not surprising that no DNA was retrieved from it. The presence of moa DNA in the Taupo ignimbrite is surprising, but could result from moa trampling faeces or feathers into the ash. However, given the particularly violent way the ignimbrite was deposited, it is likely that the DNA was attached to loose floor sediment that was torn up and incorporated into the tephra as it was deposited. The irregular interface between the pre-Taupo sediment and the ignimbrite indicates that the emplacement disturbed and deformed the surface of the cave floor.
A small degree of sequence variation within species of moa was observed. It is impossible to attribute this variation to postmortem damage, polymerase errors, or genuine polymorphism because sediments contain genetic material from an unknown number of individuals. This is one of the limitations of studying aDNA in sediments. A measure of damage could be obtained by Uracil-N-Glycosylase (UNG)–treating extracts before PCR amplification (Hofreiter et al. 2001) and comparing the cloned results with those obtained without UNG treatment, but this is beyond the scope of this paper. Regardless of the cause of the sequence variation, the taxonomic affiliations of the sequences were unambiguous.
Plant DNA
Amplifications, cloning, and sequencing of plant DNA demonstrated the presence in the sediments of DNA from a diverse range of plant families (table 2), all of which are still present in or near the study area. Although the plants could be identified to family level only, with species-specific primers, and it should be possible to test for the presence of particular species in temperate sediments.
Strata | Sample Number | |
Hukanui Pool | ||
F European | HPLF-70 | Araliaceae (92%)a, (100%)b, Asteraceae (100%)b, Fabaceae (99%)a, Griseliniaceae (75%)b, Plantaginaceae (100%)b, Poaceae (100%)b, Rosaceae (100%)b |
E Post-Taupo | HPLE-150 | Poaceae (100%)a, Polygonaceae (96%)a |
D Taupo | HPLD-90 | Asteraceae (99%)a, Coriariaceae (92%)a |
C Pre-Taupo | HPLC-10 | Onagraceae (94%)b, Plantaginaceae (100%)b, Polygonaceae (99%)a, Ruscaceae (99%)b |
B Waimihia | HPLB-10 | Onagraceae (71%)a |
A Pre-Waimihia | ||
Hukanui #7a | ||
F European/mixed | H#7a Layer F-3 | Poaceae (100%)c, Asteraceae (100%)c, Lamiaceae (74%)c, Onagraceae (100%)c, Fabaceae (100%)c, Juncaceae (100%)c |
E Post-Taupo | H#7a Layer E-10H#7a Layer E-80 | Asteraceae (100%)c, Onagraceae (100%)c, Polygonaceae (90%)b |
D Taupo | H#7a Layer D-100 | |
C Pre-Taupo | H#7a Layer C-160 | Ranunculaceae (100%)c |
B Waimihia | Not present | |
A Pre-Waimihia | Not present |
Strata | Sample Number | |
Hukanui Pool | ||
F European | HPLF-70 | Araliaceae (92%)a, (100%)b, Asteraceae (100%)b, Fabaceae (99%)a, Griseliniaceae (75%)b, Plantaginaceae (100%)b, Poaceae (100%)b, Rosaceae (100%)b |
E Post-Taupo | HPLE-150 | Poaceae (100%)a, Polygonaceae (96%)a |
D Taupo | HPLD-90 | Asteraceae (99%)a, Coriariaceae (92%)a |
C Pre-Taupo | HPLC-10 | Onagraceae (94%)b, Plantaginaceae (100%)b, Polygonaceae (99%)a, Ruscaceae (99%)b |
B Waimihia | HPLB-10 | Onagraceae (71%)a |
A Pre-Waimihia | ||
Hukanui #7a | ||
F European/mixed | H#7a Layer F-3 | Poaceae (100%)c, Asteraceae (100%)c, Lamiaceae (74%)c, Onagraceae (100%)c, Fabaceae (100%)c, Juncaceae (100%)c |
E Post-Taupo | H#7a Layer E-10H#7a Layer E-80 | Asteraceae (100%)c, Onagraceae (100%)c, Polygonaceae (90%)b |
D Taupo | H#7a Layer D-100 | |
C Pre-Taupo | H#7a Layer C-160 | Ranunculaceae (100%)c |
B Waimihia | Not present | |
A Pre-Waimihia | Not present |
NOTE.—Numbers in brackets represent the highest posterior probabilities over 70% obtained for that family.
rbcL H1af, rbcL H2ar.
Upper Carex 18-mer, Lower Carex 18-mer.
TrnLg, TrnLgh.
Strata | Sample Number | |
Hukanui Pool | ||
F European | HPLF-70 | Araliaceae (92%)a, (100%)b, Asteraceae (100%)b, Fabaceae (99%)a, Griseliniaceae (75%)b, Plantaginaceae (100%)b, Poaceae (100%)b, Rosaceae (100%)b |
E Post-Taupo | HPLE-150 | Poaceae (100%)a, Polygonaceae (96%)a |
D Taupo | HPLD-90 | Asteraceae (99%)a, Coriariaceae (92%)a |
C Pre-Taupo | HPLC-10 | Onagraceae (94%)b, Plantaginaceae (100%)b, Polygonaceae (99%)a, Ruscaceae (99%)b |
B Waimihia | HPLB-10 | Onagraceae (71%)a |
A Pre-Waimihia | ||
Hukanui #7a | ||
F European/mixed | H#7a Layer F-3 | Poaceae (100%)c, Asteraceae (100%)c, Lamiaceae (74%)c, Onagraceae (100%)c, Fabaceae (100%)c, Juncaceae (100%)c |
E Post-Taupo | H#7a Layer E-10H#7a Layer E-80 | Asteraceae (100%)c, Onagraceae (100%)c, Polygonaceae (90%)b |
D Taupo | H#7a Layer D-100 | |
C Pre-Taupo | H#7a Layer C-160 | Ranunculaceae (100%)c |
B Waimihia | Not present | |
A Pre-Waimihia | Not present |
Strata | Sample Number | |
Hukanui Pool | ||
F European | HPLF-70 | Araliaceae (92%)a, (100%)b, Asteraceae (100%)b, Fabaceae (99%)a, Griseliniaceae (75%)b, Plantaginaceae (100%)b, Poaceae (100%)b, Rosaceae (100%)b |
E Post-Taupo | HPLE-150 | Poaceae (100%)a, Polygonaceae (96%)a |
D Taupo | HPLD-90 | Asteraceae (99%)a, Coriariaceae (92%)a |
C Pre-Taupo | HPLC-10 | Onagraceae (94%)b, Plantaginaceae (100%)b, Polygonaceae (99%)a, Ruscaceae (99%)b |
B Waimihia | HPLB-10 | Onagraceae (71%)a |
A Pre-Waimihia | ||
Hukanui #7a | ||
F European/mixed | H#7a Layer F-3 | Poaceae (100%)c, Asteraceae (100%)c, Lamiaceae (74%)c, Onagraceae (100%)c, Fabaceae (100%)c, Juncaceae (100%)c |
E Post-Taupo | H#7a Layer E-10H#7a Layer E-80 | Asteraceae (100%)c, Onagraceae (100%)c, Polygonaceae (90%)b |
D Taupo | H#7a Layer D-100 | |
C Pre-Taupo | H#7a Layer C-160 | Ranunculaceae (100%)c |
B Waimihia | Not present | |
A Pre-Waimihia | Not present |
NOTE.—Numbers in brackets represent the highest posterior probabilities over 70% obtained for that family.
rbcL H1af, rbcL H2ar.
Upper Carex 18-mer, Lower Carex 18-mer.
TrnLg, TrnLgh.
Sheep DNA
The apparent chronological fidelity of the sheep genetic profile contrasts with that of the moa. Sheep DNA was successfully amplified from European and post-Taupo layers in both sites and also from the Taupo ignimbrite in Hukanui Pool at 350 mm below the base of the European layer. These data clearly show that sheep DNA has migrated down through the sediments and the genetic chronology has been, at least in part, disrupted. One possible reason for the difference in apparent mobility between the DNA of moa and sheep is that birds, unlike sheep, do not generate large volumes of DNA-containing urine (Valiere and Taberlet 2000). It is likely that the copious amounts of sheep urine deposited on the surface carried sheep DNA downwards, rather than sediment particles themselves moving, for which there is no corroborating geological evidence. Quantitative PCR of the sheep DNA in the sediments showed that there was approximately 8 times (3 cycle shifts) more sheep DNA in the European Layer than in the underlying Taupo ignimbrite in Hukanui Pool, which supports the hypothesis of migration by transport in urine from above (table S2, Supplementary Material online).
Studies of modern sedimentary bacterial DNA show that a major part of the nucleic acids released from their cellular matrices after cell death quickly binds to the surfaces of quartz, feldspar, clay, humic acids, and other soil components, which reduces leaching and degradation of DNA by nucleases (Lorenz and Wackernagel 1994). Thus, it could be that the mineral surfaces that bind free DNA had been saturated, and an excess of DNA would be able to pass. It is also possible that a relatively great volume of urine would simply carry its DNA load through the sediment until the flow reached its limit. Even if one or both mechanisms have functioned, DNA from nonpermafrost sediments may still be useful in reconstructing past processes, particularly where similar levels of soil DNA saturation have not been reached. However, each site will need to be assessed individually, and success may depend on the sediment composition, the biota occupying the site, and events in the site's history (such as flooding).
Conclusion
Our results suggest that the distribution of amplifiable DNA preserved directly in the noncryogenic sediments of these sites corresponds to sequences in the faunal and floral histories of the sites and their local environments reconstructed from other information. However, the results also suggest that considerable caution must be exercised in interpreting DNA profiles from sediments both because of downward movement of DNA in some circumstances and of other factors that can govern the presence of DNA from a locally occurring organism in a site or stratigraphic layer. Further studies need to be conducted on these sites to investigate sample-to-sample variation of DNA within strata and on other localities and sediment types to investigate whether DNA from upper layers regularly penetrates the layers below the occurrence of a taxon and, if so, to what extent this problem exists. The evidence presented here for organisms that do not produce copious amounts of liquid urine, suggests that most, if not all, of the DNA from such taxa is stratigraphically localized in the sediments. If the methodological challenges can be overcome, aDNA from nonfrozen sediments may prove to be a powerful molecular tool in the reconstruction of past environments.
Supplementary Material
Supplementary tables S1 and S2 are available here.
The authors thank Joergen Dissing of the University of Copenhagen for use of the ABI Prism machine; Craige Wiggins and Paul Nation, the successive farm managers of “Hukanui”; Tom Couper, the owner of “Hukanui”; and Deborah Turner, Jeanette Winn, Reg Cotter, Toni Atkinson, Rachel Vavasour, Vanessa Reid, and Trevor Worthy for assistance in the field. Deborah Turner, Dr Sam Turvey, and Polly Hall assisted R.N.H. in taking the DNA samples. This work was supported by the following funding bodies: the Arts and Humanities Research Council, United Kingdom (J.H.), the New Zealand Foundation for Research, Science and Technology Contract PLCX02 (R.N.H.), the Marsden Fund, New Zealand (R.N.H., M.B., E.W.), the Natural Environmental Research Council, United Kingdom (K.O.), Marie Curie Actions ‘FORMAPLEX’ grant, FP6-2005-025002 (M.T.P.G.), the Australian Research Council (M.B.), the Wellcome Trust, United Kingdom (E.W.), the Carlsberg Foundation, Denmark (E.W.), and the National Science Foundation, Denmark (E.W.).
References
Author notes
Peter Lockhart, Associate Editor