-
PDF
- Split View
-
Views
-
Cite
Cite
María Rodríguez-Serrano, María C. Romero-Puertas, Gabriela M. Pastori, Francisco J. Corpas, Luisa M. Sandalio, Luis A. del Río, José M. Palma, Peroxisomal membrane manganese superoxide dismutase: characterization of the isozyme from watermelon (Citrullus lanatus Schrad.) cotyledons, Journal of Experimental Botany, Volume 58, Issue 10, July 2007, Pages 2417–2427, https://doi.org/10.1093/jxb/erm095
- Share Icon Share
Abstract
In this work the manganese superoxide dismutase (Mn-SOD) bound to peroxisomal membranes of watermelon cotyledons (Citrullus lanatus Schrad.) was purified to homogeneity and some of its molecular properties were determined. The stepwise purification procedure consisted of ammonium sulphate fractionation, batch anion-exchange chromatography, and anion-exchange and gel-filtration column chromatography using a fast protein liquid chromatography system. Peroxisomal membrane Mn-SOD (perMn-SOD; EC 1.15.1.1) was purified 5600-fold with a yield of 2.6 μg of enzyme g−1 of cotyledons, and had a specific activity of 480 U mg−1 of protein. The native molecular mass determined for perMn-SOD was 108 000 Da, and it was composed of four equal subunits of 27 kDa, which indicates that perMn-SOD is a homotetramer. Ultraviolet and visible absorption spectra of the enzyme showed a shoulder at 275 nm and two absorption maxima at 448 nm and 555 nm, respectively. By isoelectric focusing, a pI of 5.75 was determined for perMn-SOD. In immunoblot assays, purified perMn-SOD was recognized by a polyclonal antibody against Mn-SOD from pea leaves, and the peroxisomal enzyme rapidly dissociated in the presence of dithiothreitol and SDS. The potential binding of the Mn-SOD isozyme to the peroxisomal membrane was confirmed by immunoelectron microscopy analysis. The properties of perMn-SOD and the mitMn-SOD are compared and the possible function in peroxisomal membranes of the peripheral protein Mn-SOD is discussed.
Introduction
Glyoxysomes are a type of specialized peroxisomes that during the early stages of oilseed germination carry out the degradation of lipids towards the synthesis of sugars in a process that involves lipid β-oxidation co-ordinated with the glyoxylate cycle (Eastmond and Graham, 2001; Baker and Graham, 2002; Cornah and Smith, 2002; Hooks, 2002). In the late stages of germination and seedling growth in the light, glyoxysomes are converted into leaf peroxisomes. This conversion implies important metabolic changes in these organelles, characterized by the loss of the glyoxylate cycle enzymes and the appearance of the newly synthesized enzymes of the photorespiration pathway (Nishimura et al., 1996; Cornah and Smith, 2002). During germination, as in other differentiation processes, there is an increase in the generation of reactive oxygen species (ROS) (Sauer et al., 2001; Bhattacharjee and Mukherjee, 2003; Clerkx et al., 2004; Sasaki et al., 2005), and the cell responds by enhancing its antioxidant capacity with the synthesis of small antioxidant molecules such as ascorbate and glutathione, and ROS-scavenging enzymes such as superoxide dismutases, catalase, ascorbate peroxidase, and glutathione reductase, among others (Stacy et al., 1999; Tommasi et al., 2001; Bailly et al., 2002; Ogawa, 2005).
Superoxide dismutases (SODs; EC 1.15.1.1) are a group of metalloenzymes that catalyse the disproportionation of superoxide radicals (O2·–), generated in different cellular compartments, to H2O2 and O2 (Fridovich, 1989). SODs are generally classified into three types depending on their different prosthetic metals: copperzinc-, iron-, and manganese-containing SODs. In higher plants, CuZn-SODs are mainly located in chloroplasts (Salin, 1988; Ogawa et al., 1995; Asada, 2000) and the cytosol (Duke and Salin, 1983; Bowler et al., 1994), and also in peroxisomes and apoplast (Sandalio and del Río, 1987; Ogawa et al., 1996; Sandalio et al., 1997; Corpas et al., 1998). Iron-containing SODs are localized in chloroplasts (Salin, 1988; Bowler et al., 1994; Asada, 2000), peroxisomes (Droillard and Paulin, 1990), and the cytosolic fraction of legume nodules (Becana et al., 1989). Mn-SODs are present in mitochondria (Sandalio and del Río, 1987; Bowler et al., 1994; del Río et al., 2003), and in different types of peroxisomes (del Río et al., 1983, 2003; Sandalio and del Río, 1988; del Río and Donaldson, 1995; Corpas et al., 1998).
In germinating watermelon (Citrullus lanatus Schrad.) cotyledons, four SOD isozymes have been identified by non-denaturing PAGE: two CuZn-SODs (I and II) and two Mn-SODs (I and II). Isozyme CuZn-SOD I was present in the cytosol whereas CuZn-SOD II was localized in peroxisomes (Sandalio and del Río, 1987, 1988). By determination of SOD latency in intact peroxisomes from watermelon cotyledons and solubilization assays with 0.2 M KCl and 0.1 M sodium carbonate, Mn-SOD I was found to be present in peroxisomal membranes as a peripheral protein possibly at the cytosolic side of the membrane (Sandalio and del Río, 1988; Sandalio et al., 1997). Accordingly, this isozyme will be designated hereafter perMn-SOD. However, Mn-SOD II was localized in mitochondria (Sandalio and del Río, 1987, 1988), and will be named mitMn-SOD. In peroxisomes from castor bean endosperm, a Mn-SOD was also identified in peroxisomal membranes as a peripheral protein (del Río and Donaldson, 1995).
In higher plants, only one Mn-SOD of peroxisomal origin has been purified to homogeneity and partially characterized, the enzyme from pea leaf peroxisomes (Palma et al., 1998), and there is very little information on the molecular properties of the SODs located in these cell organelles compared with the data available for mitochondrial SODs. The presence of an Mn-SOD in peroxisomal membranes suggests a role for this enzyme in cell signalling events, since the product of its reaction, H2O2, is an important transduction signal molecule in different physiological processes and plant stress, leading to the induction of genes encoding different cellular protectants (Desikan et al., 2000; Neill et al., 2002; Apel and Hirt, 2004; del Río et al., 2006).
In order to study the function of Mn-SOD in peroxisomal membranes and carry out comparative biochemical, immunological, and molecular studies with mitMn-SODs, the perMn-SOD from watermelon cotyledons was purified to homogeneity and characterized. The biochemical and immunological properties of this enzyme were compared with those of the mitMn-SOD from watermelon cotyledons.
Materials and methods
Plant material
Seeds of watermelon (C. lanatus Schrad., cv. sugar baby), obtained from Fitó (Barcelona, Spain), were surface-sterilized with 10% (v/v) commercial bleaching solutions for 3 min, and then were washed thoroughly with distilled water. Seeds were germinated in darkness at 30 °C for 3, 5, 7, and 9 d, as previously described (Bueno and del Río, 1992). At day 10, plantlets were transferred to the greenhouse and grown in full-nutrient media for one day more (a total time of 11 d). The greenhouse conditions were: day/night cycle, 25/18 °C, and 80% relative humidity.
Purification of the enzyme
All operations were performed at 4 °C in the presence of reducing agents. Watermelon cotyledons (100 g) were washed with distilled water, and were then homogenized in 300 ml of a medium containing 150 mM TRIS-HCl (pH 8.0), 1 mM dithiothreitol (DTT), 0.8% (w/v) polyvinylpolypyrrolidone (PVPP), 1 mM phenylmethylsulphonyl fluoride (PMSF), and 0.1% (v/v) Triton X-100 in a Sorvall Omni-Mixer. Homogenates were filtered through six layers of nylon cloth and centrifuged at 19 000 g for 30 min. The supernatant was fractionated with ammonium sulphate (30–70%; w/w), and the final pellet, which contained the perMn-SOD, was taken up in 30 ml of 150 mM TRIS-HCl, pH 8.0, 1 mM DTT (buffer T).
The resuspended pellet was dialysed overnight against buffer T, and clarified by centrifugation at 39 000 g for 15 min. The supernatant was applied to a DEAE (diethylaminoethyl)-Sephadex A-25 column [12.5×5 (i.d.) cm], equilibrated with buffer T. PerMn-SOD was eluted after washing the column with 490 ml of buffer T containing 0.1 M KCl. Fractions containing perMn-SOD activity were concentrated up to 25 ml with a PM-10 membrane, and applied to a Mono Q HR 10/10 column connected to an FPLC (fast protein liquid chromatography) system (Pharmacia LKB Biotechnology). Sample volumes of 2 ml (∼70–100 μg of protein) were loaded onto the Mono Q column, previously equilibrated with 50 mM K-phosphate, pH 7.8, 1 mM DTT (buffer P). The fractionation was done at room temperature. The column was washed with 144 ml of buffer P, and then was eluted with 224 ml of a linear salt gradient (0–0.4 M KCl) in buffer P. Fractions of 4.0 ml were collected at a flow rate of 4 ml min−1. The perMn-SOD bound weakly to the column, and was eluted at the beginning of the gradient application. The perMn-SOD activity-containing fractions were pooled, concentrated by ultrafiltration, and then loaded onto a Sephadex G-150 superfine column (2.6×88 cm; Pharmacia LKB Biotechnology), equilibrated with buffer P. Sample volumes of 2 ml were applied to the column, and 2 ml fractions were collected at a flow rate of 10–12 ml h−1. The fractions containing perMn-SOD activity were pooled, concentrated by ultrafiltration with a PM-10 membrane, and stored at –20 °C.
Determination of relative molecular mass and subunit size
The relative molecular mass of perMn-SOD was determined by gel filtration on a Sephadex G-150 superfine column (2.6×88 cm; Pharmacia LKB Biotechnology), equilibrated with 50 mM K-phosphate buffer, pH 7.8.
The subunit size of the enzyme was determined by SDS–PAGE after heating the samples at 100 °C for 5 min in the presence of 0.1% (w/v) SDS and 5 mM DTT. Electrophoresis was performed on 12% acrylamide–SDS slab minigels (Bio-Rad Laboratories, MiniProtean II), as described by Laemmli (1970). Standards used were: phosphorylase b (Mr=97 400 Da), bovine serum albumin (BSA; Mr=66 000 Da), ovalbumin (Mr=45 000 Da), carbonic anhydrase (Mr=31 000 Da), soybean trypsin inhibitor (Mr=21 500 Da), and lysozyme (Mr=14 400 Da) (Bio-Rad Laboratories). Proteins were visualized in gels by silver staining.
Electrophoretic methods
Non-denaturing PAGE was performed on 10% acrylamide cylindrical and slab gels as described by Davis (1964). SOD activity was detected in gels by the photochemical NBT (nitroblue tetrazolium) reduction method (Beauchamp and Fridovich, 1971). SDS–PAGE of crude extracts from watermelon cotyledons was carried out using 12% acrylamide gels. Polypeptides were stained with Coomassie blue as reported earlier (Palma et al., 1997).
Proteins fron native- and SDS–PAGE were also transferred to PVDF (polyvinylidene difluoride) membranes (Immobilon P membranes) in a Mini Trans-Blot Cell, by following the manufacturer's directions (Bio-Rad Laboratories). After transfer, membranes were processed for recognition with a specific antibody against Mn-SOD from pea leaves (see below), using the immunoblot conditions described by Bueno et al. (1995). Antibodies against CuZn-SOD from watermelon cotyledons (Bueno et al., 1995) and catalase from pumpkin cotyledons (Yamaguchi and Nishimura, 1984) were also used.
Isoelectric focusing was carried out in 4.85% acrylamide (T5; C3) slab gels containing 2.3% (w/v) ampholytes (pH range 5–7) and 10% (v/v) glycerol, using a vertical system (Mini Protean II, Bio-Rad Laboratories) (Palma et al., 1997). The following isoelectric point standards (Pharmacia LKB Biotechnology) were used: pepsinogen (pI=2.80), amyloglucosidase (pI=3.50), red-methyl (pI=3.75), glucose oxidase (pI=4.15), soybean trypsin inhibitor (pI=4.55), β-lactoglobulin A (pI=5.20), bovine carbonic anhydrase (pI=5.85), and human carbonic anhydrase (pI=6.55). After isoelectric focusing, gels were processed either for SOD activity (NBT reduction method) or protein staining (silver).
Preparation of an antibody against pea leaf Mn-SOD
Mn-SOD was purified to homogeneity from pea (Pisum sativum L.) leaves, as described by del Río et al. (1983). The antibodies were prepared in New Zealand rabbits by Immune Systems Ltd (Paignton, UK) by injection of 100 μg of purified Mn-SOD as a single dose. The IgG fraction of the serum was isolated using an Econ-Pac Serum IgG purification kit (Bio-Rad) and was tested for monospecificity by western blot using the pre-immune serum as negative control. The IgG fraction was concentrated to approximately 3 mg protein ml−1, and kept frozen until further use. This antibody was used for antigenic cross-reactivity assays with crude extracts of pea leaves and roots, pepper, tomato, and Arabidopsis leaves, rat and trout livers, and crude extracts of shoots and roots from 9 d watermelon seedlings. All extracts were prepared in 50 mM TRIS-HCl buffer, pH 8.0, 0.1% (v/v) Triton X-100, 1 mM DTT (1:4; w/v). Homogenates were filtered through four layers of nylon cloth and centrifuged at 27 000 g for 30 min. The supernatants were used for the assays.
Purification of cell organelles and peroxisomal membranes
Peroxisomes and mitochondria were isolated from watermelon cotyledons by differential and sucrose density-gradient centrifugations (20–57%, w/w), as described by Sandalio and del Río (1987). After centrifugation, gradients were eluted with an Isco gradient fractionator (model 185) equipped with an optical unit and an absorbance detector. Sucrose density-gradients were characterized using catalase as marker enzyme for peroxisomes (Aebi, 1984) and fumarase for mitochondria (Walk and Hock, 1977). Peroxisomes and mitochondria were well separated in the gradients, with practically no cross-contamination and a good intactness degree (Sandalio and del Río, 1987, Sandalio and del Río, 1988). For the preparation of peroxisomal membranes, the peroxisomal peaks from the sucrose density-gradient were pooled and broken by hypotonic shock in 50 mM K-phosphate buffer, pH 7.8, containing 0.02 mM FAD and 0.1 mM DETAPAC (diethylenetriaminepentaacetic acid), and the pellet was separated by centrifugation at 151 000 g for 30 min (Sandalio et al., 1988).
Electron microscopy and immunocytochemistry
Cotyledon segments (1 mm2) were fixed according to Corpas et al. (1994). Labelling experiments were done using ultrathin sections with the antibody against Mn-SOD (dilution 1:500), reported above, as primary antibody, and goat anti-rabbit IgG conjugated to 15 nm gold particles as secondary antibody (Sandalio et al., 1997). Sections were post-stained in 2% (w/v) uranyl acetate for 3 min and examined with a Zeiss EM 10C transmission electron microscope.
Enzyme and protein assays
Hydroxypyruvate reductase and isocitrate lyase were determined by the method described by Schwitzguébel and Siegenthaler (1984) and Archer and Ting (1996), respectively. For the catalase activity, the method of Aebi (1984) was followed. SOD activity was measured by the ferricytochrome c method, using xanthine/xanthine oxidase as the source of superoxide radicals (McCord and Fridovich, 1969). Proteins were determined by the method of Lowry et al. (1951), using BSA as a standard protein.
Results
In order to select the appropriate material for the purification of the perMn-SOD, the activity of SOD isozymes and different marker enzymes was analysed in watermelon cotyledons during seed germination and seedling development. The activity of the photorespiratory enzyme hydroxypyruvate reductase (HRP) and that of the glyoxylate cycle isocitrate lyase (ICL) was analysed (Fig. 1A). Catalase activity (Fig. 1A) displayed a parallel behaviour to that observed for the protein content in western blot assays (Fig. 1C). Total SOD activity gradually increased with time and reached a maximum value at 11 d (Fig. 1A). The analysis of the SOD isoenzymes in native gels showed that the total Mn-SOD (perMn-SOD and mitMn-SOD) and CuZn-SOD I activities were maintained between 5 d and 11 d (Fig. 1B). In contrast, the activity of isozyme CuZn-SOD II increased with time and reached a maximum in 9 d cotyledons. Western blot analysis showed that the level of total Mn-SOD protein was steady until day 9, and then was clearly reduced (Fig. 1C). CuZn-SOD protein content was enhanced with time, with the highest intensity being observed at day 9. On the basis of these results, the growth time selected for obtaining cotyledons for the purification of perMn-SOD was 9 d, because at this time the Mn-SOD activity was comparatively high.
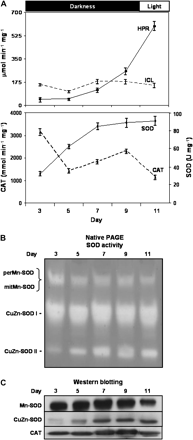
Hydroxypyruvate reductase (HPR), isocitrate lyase (ICL), superoxide dismutase (SOD), and catalase (CAT) activities in watermelon cotyledons during seed germination and development. Seeds were sown and grown in the dark except for those at the last time studied (11 d) that were grown for 1 d under greenhouse conditions. At 3, 5, 7, 9, and 11 d growth, crude extracts of watermelon cotyledons were prepared and analysed. (A) Total enzymatic activities. Activity data are expressed as the mean ±SEM of three independent experiments. (B) Activity of SOD isoenzymes separated by native PAGE. For each sample, 45 μg of protein was loaded per lane. (C) Western blotting of samples (20 μg protein) using antibodies against Mn-SOD from pea leaves, CuZn-SOD from watermelon cotyledons, and catalase from pumpkin cotyledons. All antibodies were used at a 1:1000 dilution.
The purification of perMn-SOD from watermelon cotyledons is summarized in Table 1. The enzyme was purified about 5600-fold with a yield of 260 μg of protein per 100 g of cotyledons. Purified Mn-SOD had a specific activity of 480 U mg−1 protein. FPLC on the anion exchanger Mono Q was a crucial step since all the SOD isozymes present in extracts of watermelon cotyledons (Mn-SOD I and II, and CuZn-SOD I and II) could be separated as four independent activity peaks. PerMn-SOD (Mn-SOD I) eluted in the Mono Q column at a KCl range of 0.03–0.08 M, whereas mitMn-SOD (Mn-SOD II) was eluted at 0.14–0.22 M KCl (Fig. 2A, B), and corresponded to SOD activity peaks 3 and 4, respectively. Therefore, peaks 3 and 4 were pooled independently and used for the following purification steps of both perMn-SOD and mitMn-SOD.
Purification of peroxisomal membrane Mn-SOD from watermelon cotyledons (100 g of cotyledons)
Step | Total activity (U) | Total protein (mg) | Specific activity (U mg−1) | Yield (%) | Purification (fold) |
Crude extract | 278 | 3232 | 0.086 | 100 | 1 |
(NH4)2SO4 precipitate (30–70%; w/w) | 271 | 2212 | 0.123 | 97.4 | 1.43 |
Batch DEAE-Sephadex | 220 | 1238 | 0.178 | 79.1 | 2.07 |
Mono Q FPLCa | 169 | 5.503 | 30.71 | 60.8 | 357 |
Sephadex G-150 | 126 | 0.261 | 483 | 45.3 | 5616 |
Step | Total activity (U) | Total protein (mg) | Specific activity (U mg−1) | Yield (%) | Purification (fold) |
Crude extract | 278 | 3232 | 0.086 | 100 | 1 |
(NH4)2SO4 precipitate (30–70%; w/w) | 271 | 2212 | 0.123 | 97.4 | 1.43 |
Batch DEAE-Sephadex | 220 | 1238 | 0.178 | 79.1 | 2.07 |
Mono Q FPLCa | 169 | 5.503 | 30.71 | 60.8 | 357 |
Sephadex G-150 | 126 | 0.261 | 483 | 45.3 | 5616 |
FPLC, fast protein liquid chromatography.
Purification of peroxisomal membrane Mn-SOD from watermelon cotyledons (100 g of cotyledons)
Step | Total activity (U) | Total protein (mg) | Specific activity (U mg−1) | Yield (%) | Purification (fold) |
Crude extract | 278 | 3232 | 0.086 | 100 | 1 |
(NH4)2SO4 precipitate (30–70%; w/w) | 271 | 2212 | 0.123 | 97.4 | 1.43 |
Batch DEAE-Sephadex | 220 | 1238 | 0.178 | 79.1 | 2.07 |
Mono Q FPLCa | 169 | 5.503 | 30.71 | 60.8 | 357 |
Sephadex G-150 | 126 | 0.261 | 483 | 45.3 | 5616 |
Step | Total activity (U) | Total protein (mg) | Specific activity (U mg−1) | Yield (%) | Purification (fold) |
Crude extract | 278 | 3232 | 0.086 | 100 | 1 |
(NH4)2SO4 precipitate (30–70%; w/w) | 271 | 2212 | 0.123 | 97.4 | 1.43 |
Batch DEAE-Sephadex | 220 | 1238 | 0.178 | 79.1 | 2.07 |
Mono Q FPLCa | 169 | 5.503 | 30.71 | 60.8 | 357 |
Sephadex G-150 | 126 | 0.261 | 483 | 45.3 | 5616 |
FPLC, fast protein liquid chromatography.
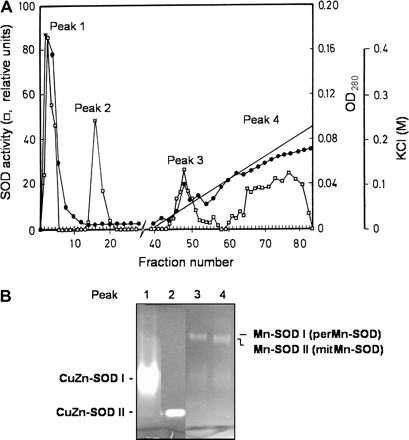
Fractionation of superoxide dismutases from watermelon cotyledons on a Mono Q 10/10 column using an FPLC system. (A) Optical density (OD280) and SOD activity in the eluted fractions. (B) Native PAGE of the SOD activity peaks eluted from the column.
Analysis by SDS–PAGE of the perMn-SOD-containing fractions eluted from the Sephadex G-150 column showed a single protein band after silver staining, thus indicating that the isozyme was homogenous (Fig. 3). The purified enzyme was recognized by a polyclonal antibody against Mn-SOD from pea leaves. By SDS–PAGE on 12% polyacrylamide gels the subunit size of the enzyme was estimated to be 27 kDa (Fig. 3), whereas a native molecular mass of 108 kDa was obtained for perMn-SOD by gel filtration through Sephadex G-150 (Fig. 4). Using the same method, a native molecular mass of 112 kDa was determined for the partially purified mitMn-SOD from watermelon cotyledons (Fig. 4). The visible absorption spectrum of the purified enzyme was characterized by two small absorption maxima at 448 nm and 555 nm (Fig. 5). In the UV region, a shoulder at 275 nm was detected, and at that wavelength perMn-SOD had a molar extinction coefficient (ϵ275) of 4.76×104 M−1 cm−1. The isoelectric point of purified perMn-SOD, determined by isoelectric focusing, was 5.75, whereas for the mitMn-SOD a pI value of 5.66 was obtained (Fig. 6).
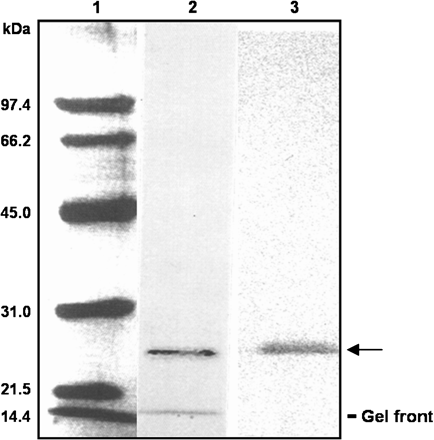
SDS–PAGE and immunoblotting of purified perMn-SOD from watermelon cotyledons. 1, Molecular mass markers; 2, silver-stained pure Mn-SOD (2 μg); 3, western blot (80 ng of protein) assay using an antibody against Mn-SOD from pea leaves. A 1:1000 dilution of the antibody was used. The mobility of the purified perMn-SOD is indicated by an arrow. The faster band observed in lane 2 corresponds to the front.
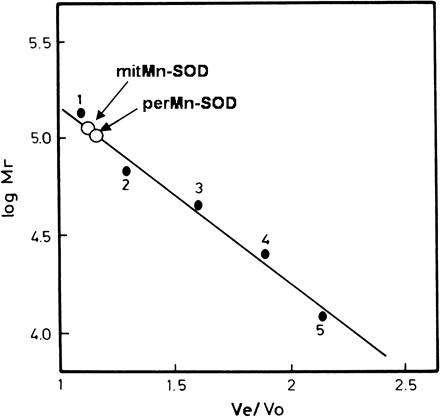
Native molecular mass determination of peroxisomal Mn-SOD by gel filtration on Sephadex G-150. 1, Aldolase (Mr=160 000); 2, BSA (Mr=67 000); 3, ovalbumin (Mr=45 000); 4, chymotrypsinogen (Mr=25 000); 5, cytochrome c (Mr=12 400). In order to determine the molecular size, chromatographies in which either purified perMn-SOD or partially purified mitMn-SOD were loaded separately, were run.

UV-visible absorption spectra of peroxisomal Mn-SOD from watermelon cotyledons. An enzyme concentration of 260 μg ml−1 in 150 mM TRIS-HCl, pH 8.0, 1 mM DTT, was used.
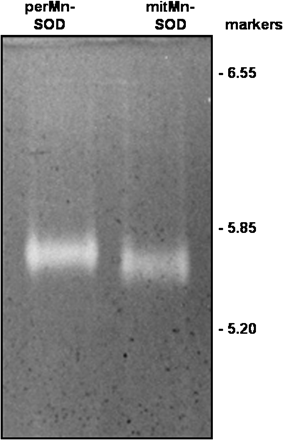
Isoelectric focusing of perMn-SOD and mitMn-SOD from watermelon cotyledons. Runs were performed on 4.85% acrylamide gels with ampholytes in the pH 5–7 range, and loading 1 μg of protein per lane. Markers are indicated on the right.
The effect of high temperature on the stability of the quaternary structure of perMn-SOD was also studied. Pure perMn-SOD was incubated in the presence of 5 mM DTT both at room temperature for 0 min and at 100 °C for 5, 10, and 15 min, and then was subjected to SDS–PAGE on 12% polyacrylamide gels. A single band of 27 kDa was observed in all cases (Fig. 7).
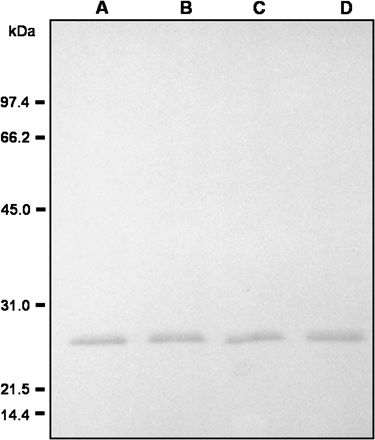
Dissociation of perMn-SOD under denaturing conditions. Pure enzyme (100 ng) was incubated in the presence of 5 mM DTT at room temperature for 0 min and at 100 °C for 5, 10, and 20 min. Then, samples were assayed by western blot using an antibody against Mn-SOD from pea leaves (1/1000 dilution). A, room temperature, 0 min; B, 100 °C, 5 min; C, 100 °C, 10 min. D: 100 °C, 20 min.
The cross-reactivity of the antibody obtained against Mn-SOD from pea leaves was assayed against different crude extracts of plant and animal origin (Fig. 8). The antibody cross-reacted with pea leaf and root crude extracts, and the highest recognition band was observed in roots. In all these cases, an immunoreactive band of 27 kDa was detected. The antibody also cross-reacted with Mn-SODs from pepper and Arabidopsis leaves, trout liver, and, to a lower extent, tomato leaves, but did not recognize rat liver crude extracts. The antibody to Mn-SOD also showed reactivity with several organs of watermelon seedlings, with a maximum intensity in cotyledons, followed by roots and shoots.
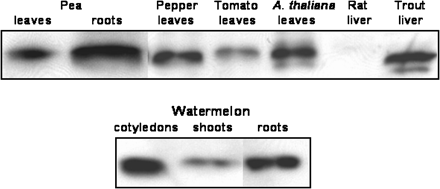
Cross-reactivity of the antibody against pea leaf Mn-SOD with cell-free extracts from plant and animal sources. Proteins were separated by SDS–PAGE in 12% acrylamide gels. The antibody to Mn-SOD from pea leaves was used at a 1:1000 dilution. The samples assayed included: watermelon cotyledons, shoots and roots; pea leaves and roots; pepper leaves; tomato leaves; A. thaliana leaves; rat liver; and trout liver. Crude extracts were prepared as described in the Materials and methods. For each sample, 10 μg protein was loaded.
The electrophoretic behaviour under native conditions of peroxisomal and mitochondrial Mn-SODs from watermelon cotyledons was compared (Fig. 9). Partially purified mitMn-SOD, obtained as reported by Pastori et al. (1996), was used. Purified perMn-SOD- and mitMn-SOD-enriched fractions were loaded onto native polyacrylamide gels, and were then immunoblotted. PerMn-SOD and mitMn-SOD showed distinct electrophoretic mobilities, perMn-SOD being slower than the mitochondrial enzyme. The subcellular localization of peroxisomal and mitochondrial Mn-SODs from watermelon cotyledons is illustrated in Fig. 9. Mitochondria and peroxisomes isolated from watermelon cotyledons, and the purified perMn-SOD and the partially purified mitMn-SOD from the same tissue were separated by native PAGE, and gels were stained for SOD activity. Pure perMn-SOD co-migrated with the SOD activity band detected in the peroxisomal membranes, whereas partially purified mitMn-SOD had a similar electrophoretic mobility to the mitochondrial fractions.
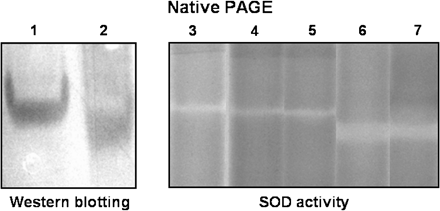
Electrophoretic pattern of purified perMn-SOD and mitMn-SOD from watermelon cotyledons in native gels. 1, Purified perMn-SOD (1 μg); 2, partially purified mitMn-SOD (1 μg); 3, purified perMn-SOD (80 μg); 4, whole peroxisomes from watermelon cotyledons (50 μg); 5, peroxisomal membranes from watermelon cotyledons (25 μg); 6, mitochondria from watermelon cotyledons (50 μg); 7, partially purified mitMn-SOD (10 μg). Subcellular fractions from watermelon cotyledons were purified as indicated in the Materials and methods. A 1:1000 dilution of the antibody against pea leaf Mn-SOD was used.
The localization of Mn-SOD in watermelon cotyledons was investigated by immunogold electron microscopy using the antibody reported above. Within the cell, labelling was exclusively associated with mitochondria and with the peroxisomal membrane (Fig 10A–C). However, gold particles were also detected in the extracellular space (Fig. 8C). Incubation with the pre-immune serum did not show any gold labelling in the tissue (Fig. 10D).
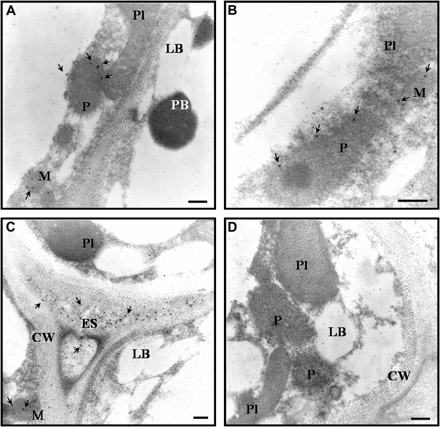
Immunogold electron microscopy localization of Mn-SOD in watermelon cotyledons. Arrows show the localization of Mn-SOD indicated by the detection of the 15 nm gold particles. (A–C) Micrographs showing labelling with the antibody raised against Mn-SOD from pea leaves (dilution 1:500). (D) Micrograph of the tissue incubated with the pre-immune serum. CW, cell wall; ES, extracellular space; LB, lipid body; M, mitochondria; P, peroxisome; Pl, plastid; PB, protein body. Bars represent 1 μm.
Discussion
Characterization of the peroxisomal Mn-SOD from watermelon cotyledons
The plant material used for the purification of perMn-SOD consisted of 9-d-old cotyledons. During germination of oilseeds, a transition of glyoxysomes into leaf peroxisomes takes place during the change from heterotrophic to photoautotrophic growth (Nishimura et al., 1996; Cornah and Smith, 2002). In the present experimental conditions, glyoxysomes still maintained functional the glyoxylate cycle, as shown by the ICL activity, although the photorespiration pathway was being induced, indicating that the metabolic conversion of glyoxysomes into peroxisomes had not been completed yet.
Two buffer systems were used in the purification of perMn-SOD. TRIS-HCl (buffer T) was used as the basic buffer for the first steps of the purification procedure since higher yields were achieved, and K-phosphate buffer (buffer P) was used in the last two chromatographic steps. Moreover, 1 mM DTT had to be used in all buffers throughout the whole purification procedure to improve the enzyme stability and final recovery yields. In the absence of this reducing agent, most of the perMn-SOD activity was lost (results not shown). A similar requirement for DTT to stabilize the enzymatic activity has been reported in the purification of the perMn-SOD from pea leaves (Palma et al., 1998).
After anion-exchange chromatography through a Mono Q column, the four SOD isozymes present in crude extracts of watermelon cotyledons (CuZn-SODs I and II, and Mn-SODs I and II) were completely separated, and no cross-contamination was found in the corresponding activity peaks eluted. However, when buffer T was used in this column chromatography step, isozymes CuZn-SOD I and II co-eluted, and perMn-SOD and mitMn-SOD were not well differentiated (results not shown).
The purification procedure reported here achieved high purification yields compared with those used for the purification of other plant Mn-SODs (Sevilla et al., 1980a; Streller et al., 1994; Kröniger et al., 1995; Palma et al., 1998). However, the specific activity of purified perMn-SOD, obtained in this work, was lower than that reported for other Mn-SODs. This might be due to a lower stability of the protein quaternary structure of this Mn-SOD with possible losses of the enzyme's manganese cofactor. In fact, at room temperature, perMn-SOD of watermelon cotyledons was rapidly dissociated into its monomers and no bands of dimeric, trimeric, or tetrameric forms were detected in PVDF membranes (Fig. 7, lane A). This could explain the low amounts of manganese detected in purified perMn-SOD when the metal–protein stoichiometric analysis was attempted (results not shown). Similar results on the instability of Mn-SOD were also found in Norway spruce needles, where the enzyme dissociated in the absence of 2-mercaptoethanol (Kröniger et al., 1995).
The native perMn-SOD and its monomeric subunit were recognized by an antibody raised against Mn-SOD from pea leaves, as well as the mitMn-SOD from watermelon cotyledons. The native molecular mass and the subunit size of perMn-SOD are in the range reported for other plant Mn-SODs characterized so far (Sevilla et al., 1982; Hassan and Scandalios, 1990; Streller et al., 1994; Kröniger et al., 1995; Palma et al., 1998), with the exception of a Mn-SOD from tea leaves, recently characterized, which apparently has a molecular mass of 169 kDa (Vyas and Kumar, 2005). Results obtained in this work on the native enzyme molecular mass (108 kDa) and monomer size (27 kDa) indicate that perMn-SOD of watermelon cotyledons is a tetramer composed of four equal subunits. The partially purified mitMn-SOD from watermelon cotyledons has a slightly higher native (112 kDa) and subunit (28 kDa) molecular mass than perMn-SOD (Pastori et al., 1996). The native molecular mass of perMn-SOD was also estimated by gel filtration in the presence of 1 mM DTT, and, in these conditions, a molecular mass of 82 kDa was obtained (results not shown). This difference in the molecular mass in the presence and absence of 1 mM DTT was also reported for the Mn-SOD purified from pea leaf peroxisomes (Palma et al., 1998), and could be due to a more compact folding of the enzyme molecule induced by reduction of the protein –SH groups, while in the absence of DTT disulphide bonds can be oxidized and this might produce changes in the enzyme structure, leading to abnormal protein sizes. However, it cannot be ruled out that in the presence of DTT, perMn-SOD might show an odd behaviour on gel filtration and not act as a global protein. The UV-visible spectra of perMn-SOD showed some similarities to those described for other plant Mn-SODs. Thus, the Mn-SOD purified from pea leaves was characterized by absorbance peaks at 275 nm and 290 nm (Sevilla et al., 1980b), whereas peroxisomal Mn-SOD from pea leaves showed maxima at 278, 290, 483, and 542 nm (Palma et al., 1998; see also references therein). In watermelon cotyledons, in the UV region, a shoulder at 275 nm characteristic of phenylalanine was observed. In the visible region, two maxima at 555 nm and 448 nm were detected, the former possibly due to the enzyme's metal prosthetic group.
Binding of peroxisomal Mn-SOD to the organelle membrane
Results reported in this work have confirmed the membrane-bound localization of a Mn-SOD in peroxisomes from watermelon cotyledons, whereas the major Mn-SOD in this tissue (Mn-SOD II) was reported to be present in mitochondria (Sandalio and del Río, 1987). Thus, the immunocytochemistry data shown here clearly demonstrate the dual localization of Mn-SOD in the mitochondrial matrix and in the peroxisomal membrane from this tissue. The localization of Mn-SOD in the peroxisomal membranes was first accomplished in watermelon cotyledons by latency and solubilization assays using catalase, malate synthase, and citrate synthase as markers of peroxisomal soluble enzymes (Sandalio and del Río, 1988). The presence of a Mn-SOD bound to membranes contrasts with most Mn-SODs, including the mitMn-SOD presented in this work, which are soluble in either mitochondria or peroxisomes.
The present experiments also detected the presence of gold labelling in the extracellular space. The existence of extracellular Mn-SOD has been reported in the moss Physcomitrella patens. In this species, the analysis of 77 expressed sequence tag (EST) clones coding for germin-like proteins (GLPs) showed that one of those clones displayed manganese-containing extracellular SOD activity (Nakata et al., 2004), and its sequence was similar to that of a GLP found in cotton (Kim and Triplett, 2004).
Both peroxisomal and mitochondrial Mn-SOD enzymes show similar molecular properties, as is indicated in Table 2. In the peroxisomal Mn-SOD from pea leaves, a 100% identity was found, over a 29 amino acid overlap, between the N-terminus of the peroxisomal enzyme and the mitMn-SOD, and high identity was exhibited with Mn-SODs from other plant species (Palma et al., 1998). Recently, it was reported that mitochondrial and peroxisomal Mn-SODs from pea leaves are regulated differently during leaf senescence (del Río et al., 2003). However, the genetic control of the two Mn-SODs in watermelon cotyledons still remains unknown. The isolation and characterization of cDNAs of watermelon SODs is under way in our laboratory. So far, only one partial clone of Mn-SOD having 436 nucleotides has been obtained in watermelon cotyledons (AY542525). Further research is necessary to clone the cDNA encoding the perMn-SOD. This will supply information on the full amino acid sequence of perMn-SOD and will make it possible to study its physiological function as a peripheral protein in the peroxisomal membrane.
Source | Isozyme | Localization | Molecular mass (kDa) | Subunit size (kDa) | pI | Reference |
Watermelon cotyledons | PerMn-SOD | Peroxisomal membrane | 108 | 27 | 5.75 | This work |
Watermelon cotyledons | MitMn-SOD | Mitochondria | 112 | 27.8 | 5.66 | This work; Pastori et al (1996) |
Pea leaves | PerMn-SOD | Peroxisomal matrix | 92 | 27 | 5.53 | Palma et al. (1998) |
Source | Isozyme | Localization | Molecular mass (kDa) | Subunit size (kDa) | pI | Reference |
Watermelon cotyledons | PerMn-SOD | Peroxisomal membrane | 108 | 27 | 5.75 | This work |
Watermelon cotyledons | MitMn-SOD | Mitochondria | 112 | 27.8 | 5.66 | This work; Pastori et al (1996) |
Pea leaves | PerMn-SOD | Peroxisomal matrix | 92 | 27 | 5.53 | Palma et al. (1998) |
Source | Isozyme | Localization | Molecular mass (kDa) | Subunit size (kDa) | pI | Reference |
Watermelon cotyledons | PerMn-SOD | Peroxisomal membrane | 108 | 27 | 5.75 | This work |
Watermelon cotyledons | MitMn-SOD | Mitochondria | 112 | 27.8 | 5.66 | This work; Pastori et al (1996) |
Pea leaves | PerMn-SOD | Peroxisomal matrix | 92 | 27 | 5.53 | Palma et al. (1998) |
Source | Isozyme | Localization | Molecular mass (kDa) | Subunit size (kDa) | pI | Reference |
Watermelon cotyledons | PerMn-SOD | Peroxisomal membrane | 108 | 27 | 5.75 | This work |
Watermelon cotyledons | MitMn-SOD | Mitochondria | 112 | 27.8 | 5.66 | This work; Pastori et al (1996) |
Pea leaves | PerMn-SOD | Peroxisomal matrix | 92 | 27 | 5.53 | Palma et al. (1998) |
Role of peroxisomal Mn-SOD in the metabolism of watermelon cotyledons
In this work, the purification to homogeneity and characterization of the perMn-SOD from watermelon cotyledons is reported. To our knowledge, this is the second Mn-SOD purified from plant peroxisomes, and the first membrane-bound Mn-SOD characterized from plant peroxisomes. This is also the first report of a Mn-SOD whose binding to the peroxisomal membrane has been unequivocally demonstrated by immunoelectron microscopy analysis. CuZn-SODs and Fe-SODs, which are usually soluble enzymes in different cell organelles, also occur bound to the thylakoid membranes of chloroplasts (Ogawa et al., 1995; Navari-Izzo et al., 1998; Gómez et al., 2004).
During germination of watermelon seeds, there are changes in oxidative metabolism, as deduced from the catalase and SOD activity profiles. The increase of SOD indicates that the O2·– generation rate is stimulated by the important metabolic changes that occur during germination. In peroxisomal membranes, an electron transport chain which regenerates the NAD(P)+ necessary for the organelle metabolism has been reported (Fang et al., 1987; Struglics et al., 1993; López-Huertas et al., 1999), and this chain has been proposed to be involved in the production of O2·– radicals (del Río et al., 2006). In leaf peroxisomes, three integral membrane polypeptides with molecular masses of 18, 29, and 32 kDa have been characterized and demonstrated to be responsible for O2·– generation (López-Huertas et al., 1999). The potential presence of a Mn-SOD in the external side of the peroxisomal membrane suggests a role for this enzyme in the modulation of superoxide radicals which are produced in peroxisomal membranes and released into the cytosol (del Río et al., 2006). However, the peroxisomal membrane Mn-SOD could also indirectly modulate the H2O2 concentration (its reaction product), so that the ROS O2·– and H2O2 could be used effectively by the cell as specific signal molecules. The function of perMn-SOD could be particularly useful under stress conditions, when the release of membrane-generated O2·– radicals into the cytosol can be enhanced (del Río et al., 1996, 2006). In fact, in certain plant species, Mn-SODs have been involved in the tolerance response to oxidative stress induced by abiotic conditions (Van Camp et al., 1997; Alscher et al., 2002).
This work was supported by Projects AGL2002-00988 and BFI2002-04440 from the CICYT-Fondos Europeos de Desarrollo Regional and the Junta de Andalucía (group CVI 0192) Spain. MR-S acknowledges a PhD fellowship from the Ministerio de Ciencia y Tecnología. MCR-P and GMP were holders of fellowships from the Junta de Andalucía and European Commission (Contract CHRX-CT94-0605), respectively. The authors are grateful to Dr Ana M León, Hospital Virgen del Rocío, Sevilla, and Dr Carmen García-Limones, University of Córdoba, for their help in the cross-reactivity assays of the Mn-SOD antibody. The kind donation of an antibody against catalase from pumpkin cotyledons by Dr Mikio Nishimura, National Institute for Basic Biology, Okazaki, Japan, is acknowledged. The electron microscopy studies were carried out at the Centre of Scientific Instrumentation of the University of Granada.
References
Author notes
Present address: Disease and Stress Biology Department, John Innes Centre, Norwich Research Park, Colney Lane, Norwich NR4 7UH, UK.
Present address: Crop Performance and Improvement Division, Rothamsted Research, Harpenden, Hertfordshire AL5 2JQ, UK.
Comments