-
PDF
- Split View
-
Views
-
Cite
Cite
Yu Zhao, Junling Zhang, Xiaodan Han, Saijun Fan, Total body irradiation induced mouse small intestine senescence as a late effect, Journal of Radiation Research, Volume 60, Issue 4, July 2019, Pages 442–450, https://doi.org/10.1093/jrr/rrz026
- Share Icon Share
Abstract
Radiation can induce senescence in many organs and tissues; however, it is still unclear how radiation stimulates senescence in mouse small intestine. In this study, we use the bone marrow transplantation mouse model to explore the late effects of total body irradiation on small intestine. Our results showed that almost all of the body hairs of the irradiated mice were white (which is an indication of aging) 10 months after the exposure to radiation. Furthermore, compared with the age-matched control mice, there were more SA-β-galactosidase (SA-β-gal)–positive cells and an upregulation of p16 and p21 in 8 Gy–irradiated mice intestinal crypts, indicating that radiation induced senescence in the small intestine. Intestinal bacterial flora profile analysis showed that the diversity of the intestinal bacterial flora decreased in irradiated mice; in addition it showed that the principal components of the irradiated and control mice differed: there was increased abundance of Bacteroidia and a decreased abundance of Clostridia in irradiated mice. To explore the underlying mechanism, an RNA-sequence was executed; the results suggested that pancreatic secretion, and the digestion and absorption of proteins, carbohydrates, fats and vitamins were damaged in irradiated mice, which may be responsible for the body weight loss observed in irradiated mice. In summary, our study suggested that total body irradiation may induce senescence in the small intestine and damage the health status of the irradiated mice.
INTRODUCTION
Hematopoietic stem cells transplantation (HSCT) is the most effective therapy for leukemia, lymphoma, and many other hematologic malignancies. [1, 2]. According to the guideline from the International Lymphoma Radiation Oncology Group (ILROG), the most common total body irradiation (TBI) schedules used before HSCT include the following plans: (i) twice-daily 2-Gy fractions given over 3 days (total dose 12 Gy); (ii) twice-daily 1.5-Gy fractions over 4–4.5 days (total dose 12–13.5 Gy); (iii) three-times-daily 1.2-Gy fractions over 4 days (total dose 12–13.2 Gy); and (iv) once-daily 3-Gy fractions for 4 days (total dose 12 Gy) [3]. As a result of TBI, acute injuries such as radiation pneumonitis [4] and oral mucositis [5] often occur 1–6 months after HSCT, but late injuries, including cataract [6] and gonad injury [7] can happen a long time after HSCT. It is well known that TBI can induce radiation enteritis several days after patients have been exposed to TBI [8, 9]; however, the late effects of TBI on small intestinal injury are rarely reported.
Radiation is one of the stimuli for senescence, inducing oxidative stress, DNA damage, and an inflammation response in organs and tissues. Thus, we hypothesized that TBI can also induce small intestine senescence. Senescence is defined as an irreversible growth inhibition, and this can occur when tissues or organs are exposed to potentially oncogenic stress; aging is a progressive loss of physiological integrity as a result of gradually accumulating damage over time, leading to increased vulnerability to death. Senescence has been considered as an important contributor to aging and age-related diseases [10, 11]. The most well-established functions of the small intestine are the digestion of food and the absorption of nutrients; aging can induce small intestine dysfunction and thus impair the health status of the body. The human gut microbiota is a complex system consisting of 100 trillion bacteria of from 500 to 1000 species, representing 1–3% of the body mass and encoding many genes [12, 13]. It has been reported that aging can induce bacterial overgrowth in the small intestine [14, 15] and promote changes in the microbial composition in the colon [16, 17]. In addition, the composition of the gut microbiota differs between the young and the elderly: Bacteroidetes instead of Firmicutes is dominant in the elderly gut microbiota [18, 19]. However, how the microbiota profile varies in the senescent small intestine in mice has remained unclear.
In this study, we used a bone marrow transplantation mouse model to investigate the late effects of TBI on the small intestine in mice. Our results showed that, as a late effect, TBI can induce small intestine senescence: there was decreased body weight, upregulated expression of p16 and p21, a changed intestinal bacterial flora profile (such as increased Bacteroidia and decreased Clostridia) and abnormality in the digestion and absorption functions in the small intestine of irradiated mice. In summary, TBI may induce senescence in the small intestine, thus damaging the health status of mice receiving HSCT. The precise mechanism by which TBI induces senescence, and the relationship between gene expression and bacterial flora in the small intestine, still need to be further explored.
MATERIALS AND METHODS
Mice
C57BL/6J (CD45.2) male mice (aged 8–12 weeks) were purchased from Beijing Huafukang Bioscience Co. Inc (Beijing, China). Male C57BL/6 (CD45.1 and CD45.1/45.2) mice were bred in the Experimental Animal Center of the Institute of Radiation Medicine, Chinese Academy of Medical Sciences & Peking Union Medical College (IRM-PUMC, Tianjin, China). The Animal Care and Ethics Committee of IRM-PUMC approved the use of animals for these experiments, which complied with the Guide for the Care and Use of Laboratory Animals and the National Institutes of Health guide for the Care and Use of Laboratory Animals.
Antibodies and reagents
Anti-mouse CD45.1-FITC (clone A20) and anti-mouse CD45.2-PE (clone 104) antibodies were purchased from Biolegend (San Diego, CA, USA); anti-rabbit p16 (ab211542), anti-rabbit p21 (ab109199), anti- rabbit GADPH (ab181602) and goat anti-rabbit IgG (ab6721) antibodies were purchased from Abcam (Cambridge, MA, USA). FACSTM lysing solution was purchased from BD Biosciences (San Jose, CA, USA). An SA-β-galactosidase (SA-β-gal) staining kit was purchased from Beyotime Biotechnology (Nanjing, China). TRIzol was purchased from Life Technologies (Grand Island, NY, USA). A Revert Aid First Strand cDNA Synthesis Kit was purchased from Thermo Scientific (Waltham, MA, USA). RIPA buffer was purchased from BOSTER Biological Technology co.ltd (Wuhan, China). Phenylmethylsulfonyl fluoride was purchased from Sigma (St Louis, MO, USA). PVDF Immobilon-P membranes were purchased from Millipore (Billerica, MA, USA).
TBI and competitive bone marrow transplantation
Five C57BL/6 (CD45.2) mice were exposed to 8 Gy TBI at a dose rate of 0.99 Gy/min (Gammacell-40 137Cs irradiator, Atomic Energy of Canada Ltd), then 1 × 106 donor bone marrow (BM) cells from C57BL/6 (CD45.1) mice mixed with 1 × 106 competitive bone marrow (BM) cells from C57BL/6 (CD45.1/45.2) mice were transplanted into the irradiated mice through lateral canthus vein injection. The peripheral blood from recipients was harvested 10 months after transplantation. Blood samples were stained with anti-CD45.1 and CD45.2 antibodies, and then red blood cells were removed using Lysing Solution, following the manufacturer’s instruction. Donor- and competitor-derived cells were detected by a BD Accuri C6 flow cytometer (BD Bioscience, San Jose, CA, USA) and analyzed with Flow Jo software.
SA-β-galactosidase staining
SA-β-gal staining was done using an SA-β-gal staining kit (Beyotime Biotechnology, Nanjing, China) according to the manufacturer’s instructions. Senescent cells were identified as blue-stained cells under optical microscopy. The relative signal density of SA-β-gal in the crypts was evaluated using Image Pro-Plus 6.0 software.
Isolation of small intestinal crypts
Small intestinal crypts were isolated as previously described [20, 21]. In brief, the small intestine was opened longitudinally, villi were removed by scraping them off with a glass slide, and the intestine was then cut into 2–3 cm lengths. After digestion by EDTA, the intestinal crypts were harvested.
Quantitative real-time PCR
The total RNA of the small intestinal crypts was extracted with TRIzol. Reverse transcription was performed using a RevertAid First Strand cDNA Synthesis Kit, according to the manufacturer’s protocol. Quantitative real-time PCR was conducted under an ABI 7500 Sequence Detection System (Thermo, Waltham, MA, USA). The sequences of the primers for all genes are listed in Table 1.
Gene . | Forward sequence . | Reverse sequence . |
---|---|---|
Ctrb1 | CAGGATCGTCAACGGAGAGG | ACATCGGTTGTCTTGACCCC |
Ctrl | GCCTAACCCTTAGCCTGGTC | GCCGGTGTTATCCTGGAGAG |
Apoa4 | CCAATGTGGTGTGGGATTACTT | AGTGACATCCGTCTTCTGAAAC |
Cel | CGCCTGGAGGTTCTATTTCTTG | GCCCTTGAAGATGTCAACAGA |
Cela2a | TACCCCACTTATGAGGTGGAG | GTCTGATAGTTGCTGAGGCAAT |
Cela3b | CTCGACTTCTCGCACCTACC | CACAAAGAGGTCTCCAGCGT |
Cpa1 | GAATCTCTGCCACCGACGAA | GTCGAGCAGAGACTGCACAT |
Cap2 | GACCCCGCTGGCTATCATTT | AACCCTGGTTACATAAGGCCAC |
G6pc | CGACTCGCTATCTCCAAGTGA | GTTGAACCAGTCTCCGACCA |
Fabp1 | ATGAACTTCTCCGGCAAGTACC | CTGACACCCCCTTGATGTCC |
Lct | GCATGCGCCAGCCATCTCTGA | CACAATGCCCACGCGTCCCT |
Try4 | GGGCAATGAGCAGTTTGTCA | TTAGTTTGCAGCAATGGTGTTT |
Try5 | AGGACCCTGAACAACGACATC | ATGGGGCATCCAGGCATTGAA |
Amy2a3 | GGTCTTCCTGCTGGCACATA | ATCAGCACAGCTCATGGGAA |
Prss2 | TCTGTGCTCATGACTTTCTGTCA | GCCAGCATTTAGGGACACCT |
Pnlip | CTGGGAGCAGTAGCTGGAAG | AGCGGGTGTTGATCTGTGC |
2210010C04Rik | TGGGAGAACACAACATTGATGC | AGACACAAGACACCTAGTACCAG |
Slc2a2 | TCAGAAGACAAGATCACCGGA | GCTGGTGTGACTGTAAGTGGG |
Slc9a3 | TGAAAAGCAGGACAAGGAAATCT | TTGGCCGCCTTCTTATTCTGG |
Rbp 2 | TCCTTCACAGTCACCGAACG | CTTGCGGGTGGCAAAATCAA |
Gene . | Forward sequence . | Reverse sequence . |
---|---|---|
Ctrb1 | CAGGATCGTCAACGGAGAGG | ACATCGGTTGTCTTGACCCC |
Ctrl | GCCTAACCCTTAGCCTGGTC | GCCGGTGTTATCCTGGAGAG |
Apoa4 | CCAATGTGGTGTGGGATTACTT | AGTGACATCCGTCTTCTGAAAC |
Cel | CGCCTGGAGGTTCTATTTCTTG | GCCCTTGAAGATGTCAACAGA |
Cela2a | TACCCCACTTATGAGGTGGAG | GTCTGATAGTTGCTGAGGCAAT |
Cela3b | CTCGACTTCTCGCACCTACC | CACAAAGAGGTCTCCAGCGT |
Cpa1 | GAATCTCTGCCACCGACGAA | GTCGAGCAGAGACTGCACAT |
Cap2 | GACCCCGCTGGCTATCATTT | AACCCTGGTTACATAAGGCCAC |
G6pc | CGACTCGCTATCTCCAAGTGA | GTTGAACCAGTCTCCGACCA |
Fabp1 | ATGAACTTCTCCGGCAAGTACC | CTGACACCCCCTTGATGTCC |
Lct | GCATGCGCCAGCCATCTCTGA | CACAATGCCCACGCGTCCCT |
Try4 | GGGCAATGAGCAGTTTGTCA | TTAGTTTGCAGCAATGGTGTTT |
Try5 | AGGACCCTGAACAACGACATC | ATGGGGCATCCAGGCATTGAA |
Amy2a3 | GGTCTTCCTGCTGGCACATA | ATCAGCACAGCTCATGGGAA |
Prss2 | TCTGTGCTCATGACTTTCTGTCA | GCCAGCATTTAGGGACACCT |
Pnlip | CTGGGAGCAGTAGCTGGAAG | AGCGGGTGTTGATCTGTGC |
2210010C04Rik | TGGGAGAACACAACATTGATGC | AGACACAAGACACCTAGTACCAG |
Slc2a2 | TCAGAAGACAAGATCACCGGA | GCTGGTGTGACTGTAAGTGGG |
Slc9a3 | TGAAAAGCAGGACAAGGAAATCT | TTGGCCGCCTTCTTATTCTGG |
Rbp 2 | TCCTTCACAGTCACCGAACG | CTTGCGGGTGGCAAAATCAA |
Gene . | Forward sequence . | Reverse sequence . |
---|---|---|
Ctrb1 | CAGGATCGTCAACGGAGAGG | ACATCGGTTGTCTTGACCCC |
Ctrl | GCCTAACCCTTAGCCTGGTC | GCCGGTGTTATCCTGGAGAG |
Apoa4 | CCAATGTGGTGTGGGATTACTT | AGTGACATCCGTCTTCTGAAAC |
Cel | CGCCTGGAGGTTCTATTTCTTG | GCCCTTGAAGATGTCAACAGA |
Cela2a | TACCCCACTTATGAGGTGGAG | GTCTGATAGTTGCTGAGGCAAT |
Cela3b | CTCGACTTCTCGCACCTACC | CACAAAGAGGTCTCCAGCGT |
Cpa1 | GAATCTCTGCCACCGACGAA | GTCGAGCAGAGACTGCACAT |
Cap2 | GACCCCGCTGGCTATCATTT | AACCCTGGTTACATAAGGCCAC |
G6pc | CGACTCGCTATCTCCAAGTGA | GTTGAACCAGTCTCCGACCA |
Fabp1 | ATGAACTTCTCCGGCAAGTACC | CTGACACCCCCTTGATGTCC |
Lct | GCATGCGCCAGCCATCTCTGA | CACAATGCCCACGCGTCCCT |
Try4 | GGGCAATGAGCAGTTTGTCA | TTAGTTTGCAGCAATGGTGTTT |
Try5 | AGGACCCTGAACAACGACATC | ATGGGGCATCCAGGCATTGAA |
Amy2a3 | GGTCTTCCTGCTGGCACATA | ATCAGCACAGCTCATGGGAA |
Prss2 | TCTGTGCTCATGACTTTCTGTCA | GCCAGCATTTAGGGACACCT |
Pnlip | CTGGGAGCAGTAGCTGGAAG | AGCGGGTGTTGATCTGTGC |
2210010C04Rik | TGGGAGAACACAACATTGATGC | AGACACAAGACACCTAGTACCAG |
Slc2a2 | TCAGAAGACAAGATCACCGGA | GCTGGTGTGACTGTAAGTGGG |
Slc9a3 | TGAAAAGCAGGACAAGGAAATCT | TTGGCCGCCTTCTTATTCTGG |
Rbp 2 | TCCTTCACAGTCACCGAACG | CTTGCGGGTGGCAAAATCAA |
Gene . | Forward sequence . | Reverse sequence . |
---|---|---|
Ctrb1 | CAGGATCGTCAACGGAGAGG | ACATCGGTTGTCTTGACCCC |
Ctrl | GCCTAACCCTTAGCCTGGTC | GCCGGTGTTATCCTGGAGAG |
Apoa4 | CCAATGTGGTGTGGGATTACTT | AGTGACATCCGTCTTCTGAAAC |
Cel | CGCCTGGAGGTTCTATTTCTTG | GCCCTTGAAGATGTCAACAGA |
Cela2a | TACCCCACTTATGAGGTGGAG | GTCTGATAGTTGCTGAGGCAAT |
Cela3b | CTCGACTTCTCGCACCTACC | CACAAAGAGGTCTCCAGCGT |
Cpa1 | GAATCTCTGCCACCGACGAA | GTCGAGCAGAGACTGCACAT |
Cap2 | GACCCCGCTGGCTATCATTT | AACCCTGGTTACATAAGGCCAC |
G6pc | CGACTCGCTATCTCCAAGTGA | GTTGAACCAGTCTCCGACCA |
Fabp1 | ATGAACTTCTCCGGCAAGTACC | CTGACACCCCCTTGATGTCC |
Lct | GCATGCGCCAGCCATCTCTGA | CACAATGCCCACGCGTCCCT |
Try4 | GGGCAATGAGCAGTTTGTCA | TTAGTTTGCAGCAATGGTGTTT |
Try5 | AGGACCCTGAACAACGACATC | ATGGGGCATCCAGGCATTGAA |
Amy2a3 | GGTCTTCCTGCTGGCACATA | ATCAGCACAGCTCATGGGAA |
Prss2 | TCTGTGCTCATGACTTTCTGTCA | GCCAGCATTTAGGGACACCT |
Pnlip | CTGGGAGCAGTAGCTGGAAG | AGCGGGTGTTGATCTGTGC |
2210010C04Rik | TGGGAGAACACAACATTGATGC | AGACACAAGACACCTAGTACCAG |
Slc2a2 | TCAGAAGACAAGATCACCGGA | GCTGGTGTGACTGTAAGTGGG |
Slc9a3 | TGAAAAGCAGGACAAGGAAATCT | TTGGCCGCCTTCTTATTCTGG |
Rbp 2 | TCCTTCACAGTCACCGAACG | CTTGCGGGTGGCAAAATCAA |
Western blot analysis
Small intestinal crypts were lysed in a mixture of RIPA buffer and phenylmethylsulfonyl fluoride on ice. The protein samples were subjected to 10% SDS-PAGE and transferred to PVDF Immobilon-P membranes. Antibodies against GAPDH (1:5000), p16 (1:1000) and p21 (1:1000) were diluted in Tris-buffered saline with Tween 20 (TBST) containing 5% non-fat dry milk. Primary antibody incubation was performed at 4°C overnight, and secondary antibody incubation was performed at room temperature for 2 h. Immunoreactive proteins were visualized with enhanced chemilluminescence reagents, according to the manufacturer’s recommendations.
RNA-Seq and data analysis
The total RNA was extracted from small intestine crypts; the RNA integrity was evaluated, then the libraries were constructed. These libraries were sequenced on the Illumina sequencing platform (Illumina HiSeq X Ten), and 125 bp/150 bp paired-end reads were generated. Raw reads were processed and analyzed with the help of Novegene (Beijing, China).
Analysis of intestinal bacterial flora
Mice feces were collected from the rectum and then stored at –80°C before use. Total-genome DNA was extracted from samples. 16S rRNA was amplified with the specific primer (515F, GTGCCAGCMGCCGCGGTAA, and 806R, GGACTACHVGGGTWTCTAAT) using Illumina Hiseq (Novogene Bioinformatics Technology Co., Ltd, Beijing, China). Raw reads were processed and analyzed with the help of Novegene.
Statistical analysis
Significant differences between groups were evaluated by Manny–White test analysis. All analyses were performed using GraphPad Prism 5 software, and differences were considered significant at P < 0.05.
RESULTS
TBI-induced body weight loss and hair whitening in mice
HSCT is an effective therapy for leukemia and other hematologic malignancies; however, very few researchers have reported the late effects of TBI on the small intestine after the hematopoietic system has been reconstituted. As shown in Fig. 1A, donor- and competitor-derived cells were the main types of cells detected in the peripheral blood 10 months after the transplantation of the bone marrow cells (BMCs), indicating a successful hematopoietic system reconstitution in the irradiated mice. Almost all of the body hair of the irradiated mice became white, as shown in Fig. 1B, and there was body weight loss in irradiated mice, compared with the age-matched control mice (Fig. 1C).
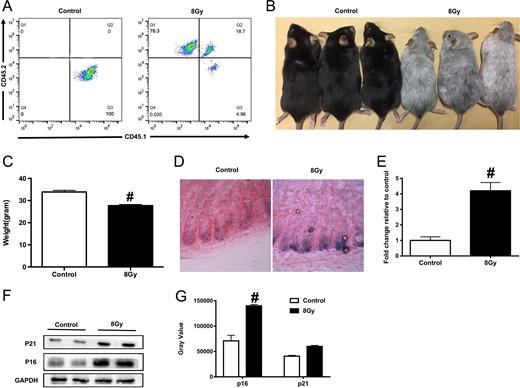
Total body irradiation (TBI) induced small intestine senescence in mice after transplantation of bone marrow cells (BMCs). Five mice were exposed to 8 Gy TBI, then BMCs were injected into mice through the lateral canthus vein. Mice were euthanized 10 months after TBI, then peripheral blood and small intestine were sampled as described in the Materials and Methods. Mice in the control group were of the same age and number as those in the TBI group. (A) Representative FACS plots showing the donor chimerism 10 months after transplantation of BMCs; (B) representative image of mice from the control and TBI groups; (C) bar graph showing the body weight of mice in the two groups (n = 5 in each group); (D) representative images of SA-β-gal staining in the small intestinal crypts; (E) bar graph showing the relative SA-β-gal intensity in the small intestinal crypts; (F) western blot images showing the expression of p21 and p16 proteins; (G) the relative quantitative analyses of p21 and p16 proteins.
TBI-induced small intestine senescence in mice
To evaluate TBI-induced senescence in the small intestine of mice, we carried out the SA-β-gal staining assay. As shown in Fig. 1D, TBI induced an increase in SA-β-gal–positive cells in the intestinal crypts, suggesting there was radiation-induced senescence in the small intestinal crypts in the mice. We further detected the expression of typical senescence-related proteins p16 and p21 [22, 23] in small intestine crypts, as shown in Fig. 1E: compared with the age-matched control group, the expression of p16 and p21 was significantly upregulated in mice exposed to 8 Gy TBI. The above data suggested that TBI induced a late effect in the small intestine of the mice, which was associated with senescence.
TBI induced alteration in the bacterial flora diversity in the mouse intestine
It has been reported that TBI induces significant changes in intestinal bacterial flora [24, 25] several days after radiation; however, how the bacterial flora changes long term after TBI has till now been unknown. As shown in Fig. 2A–E, although there was no alteration of the richness and composition of bacteria (reflected in the Chao1 and weight_unifrac index, respectively) 10 months after mice were exposed to TBI, the diversity of the intestinal bacterial flora decreased significantly, and the principal components in the two groups differed. Our data suggested that even a long time after TBI, the bacterial profile in the small intestine in the mice remained damaged.
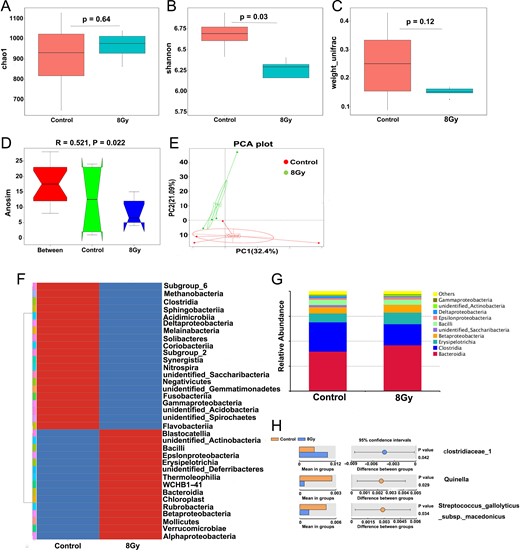
Total body irradiation (TBI)-induced variation in intestinal bacterial flora in mice. Five mice were exposed to 8 Gy TBI, then BMCs were injected into mice through the lateral canthus vein. Mice were euthanized 10 months after TBI, then fresh mice feces were obtained from the rectum, as described in the Materials and Methods. Mice in the control group were of the same age and number as those in the TBI group. (A) Chao1 diversity index, (B) Shannon diversity index; (C) β-diversity, (D) Anosim similarity analysis and (E) principal component analysis of the intestinal bacteria of control mice and TBI mice as detected by 16s high-throughput sequencing; (F) variation in intestinal bacterial flora at the class; (G) relative abundance of the top 10 intestinal bacteria at the class level 10 months after TBI; (F) differential intestinal flora at family, genus and species level 10 months after TBI.
TBI-induced increase in Bacteroidia abundance and decrease in Clostridia abundance
As shown in Fig. 2F–H, at the class level, the abundance of Bacteroidia (including Bacteroidetes, Bacilli and Betaproteobacteria) was increased, and the abundance of Bacteroidia, Bacilli and Betaproteobacteria) was decreased 10 months after mice were exposed to TBI. Among them, clostridiaceae_1 (at family level) was increased, whereas Quinella (at genus level) and Streptococcus_gallolyticus (at species level) were decreased significantly. The above data illustrate a specific changed bacterial profile 10 months after mice were exposed to TBI.
TBI-regulated RNA expression in the small intestinal crypt in mice
To illustrate the underlying mechanisms for TBI-induced injury in the small intestine in mice, we executed an RNA-sequence in the intestinal crypt. As shown in Fig. 3A, most genes in the age-matched control mice and the 8 Gy-irradiated TBI group overlapped, but there were still hundreds of differentially expressed genes specific to each group. These genes were mainly involved in pancreatic secretion, and in protein, carbohydrate, fat and vitamin digestion and absorption, as determined by a KEGG pathway enrichment analysis (Fig. 3B). As shown in Fig. 3C–D, after mice were exposed to 8 Gy TBI, Slc2a2, Slc9a3, G6pc, Apoa4, Fabp1, Rbp2 and Lct were upregulated significantly; Ctrb1, Ctrl, Cel, Cela3b, Cela2a, Cpa2, Try5, 2210010C04Rik, Amy2a3, Prss2 and Pnlip were downregulated significantly. The above data suggest that TBI induced digestion and absorption dysfunction in the mice intestine.
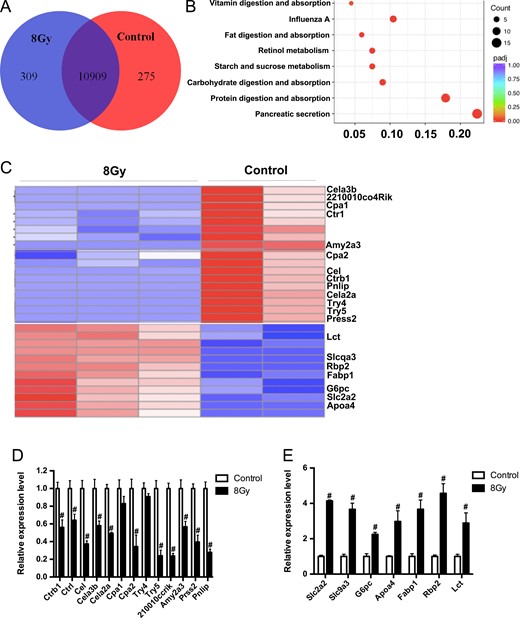
Total body irradiation (TBI)-induced gene expression changes in mice small intestine crypts. Five mice were exposed to 8 Gy TBI, then BMCs were injected into mice through the lateral canthus vein. Mice were euthanized 10 months after TBI, then small intestinal crypts were obtained from the rectum, as described in the Materials and Methods. Mice in the control group were of the same age and number as those in the TBI group. (A) Venn diagram showing the overlap and significantly differentially expressed genes between the control mice and TBI mice small intestinal crypts; (B) Kyoto Encyclopedia of Genes and Genomes (KEGG) pathway enrichment analysis 10 months after mice were exposed to TBI; (C) heat map of significantly differentially expressed genes enriched in the KEGG pathway; (D) relative expression of downregulated (D) and upregulated (E) significantly differentially expressed genes, verified by RT-PCR (n = 3 in each group).
DISCUSSION
It is well known that TBI may induce hematopoietic stem cell senescence, impaired self-renewal and a reduced long-term repopulating capacity [26]. However, has remained unclear whether there is TBI-induced senescence in the small intestine in mice. In our experiment, mice were exposed to 8 Gy TBI and then transplantation of BMCs was executed to ensure the mice survived without hematopoietic system failure. The mice were euthanized 10 months after the TBI, and donor- and competitor-derived cells were the main blood cells detected, which confirmed the success of the hematopoietic system reconstitution. To detect injury in the small intestine of the mice, we harvested the duodenum and tested for the presence of senescence markers. Compared with the age-matched control group, there were more SA-β-gal–positive cells in the crypts of the 8 Gy–irradiated mice besides, and the expression of p16 and p21 proteins were also upregulated. The small intestine is less sensitive to radiation than the hematopoietic system, as a result of its rapid response to and repair of damage [27], so the TBI dose for HSCT didn’t cause obvious gastrointestinal syndrome in mice; however, as a late effect, small intestine senescence persisted and became a threat to the animals’ health. In fact, Kumar and colleagues reported that even 2 months after mice were exposed to 0.5 Gy of γ-ray irradiation, senescence could be detected in the small intestinal crypts [28]. Senescence is a special form of durable cell-cycle arrest, preventing a damaged cell from entering cell cycling and proliferation, thus suppressing cancer in mammals [29, 30]. However, due to the senescence-associated secretory phenotype (SASP), radiation may eventually promote the initiation and progression of colorectal cancer [31]. So, it is important to accurately identify the time point at which senescent cells arise after mice have been exposed to 8 Gy, so that protective treatment can be administered in time.
As an acute effect, TBI destroys the ecological balance of the intestinal flora, and changes its richness, diversity and principal components; fecal microbiota transplantation can protect against radiation-induced injury [24], which suggests that the intestinal flora plays an important role in TBI-induced small intestinal injury. Our results showed that, even 10 months after mice were exposed to TBI, the profile of the intestinal flora was still abnormal compared with that of the age-matched control mice. Although there was no difference in the richness and composition of the bacteria, the subgroups of the intestinal bacterial flora decreased significantly, and the principal components in the two groups were significant different. The abundance of Bacteroidia had increased and that of Clostridia had decreased 10 months after the mice had been exposed to TBI. Bacteroidia has a mucolytic capacity [32, 33], which can cause colitis [34, 35] in mouse and human populations. In addition, Bacteroidia is associated with other diseases, such as celiac disease [36] and colorectal cancer [37]. Clostridia are dominant in the mouse intestine, as in other gut ecosystems [38], and are evidently important for providing colonization resistance to pathogenic Enterobacteriaceae [39]. Atarashi and colleagues reported that oral administration of a combination of 17 Clostridia strains to adult mice attenuated disease in models of colitis and allergic diarrhea [40], and Kim and colleagues reported that Clostridia protected neonatal mice from pathogen infection and abrogated intestinal pathology upon pathogen challenge [39]. We suggest that the increased Bacteroidia and decreased Clostridia is likely to cause aging-related gut diseases such as colitis in irradiated mice.
Intestinal stem cells reside at the base of the intestinal crypts; new cells are continuously generated by a population of multipotent stem cells to sustain the fast cell turnover of the small intestine [27]. Most of the senescent cells in the small intestine of irradiated mice were located in the crypts, so we analysed the gene expression in the crypts. Lots of genes were regulated and these genes were mainly involved in the digestion and absorption pathways, including the pathways for the digestion and absorption of proteins, carbohydrates, fats and vitamins. We concluded that dysfunctionality of gut digestion and absorption may have been responsible for the body weight loss of the irradiated mice. Zheng and colleagues analyzed the small intestine gene expression profile of non-human primates at 4, 7 and 12 days after they were exposed to 6.7 Gy or 7.4 Gy; their results showed that tumor necrosis factor α (TNFα) cascade, tight junction, apoptosis, cell cycle control/DNA damage repair and coagulation system signaling were significantly affected [41]. As an acute effect, radiation may activate the stress response and damage repair pathways, and as a late effect, radiation may affect the digestion and absorption pathways.
In summary, our results indicated that even if radiation-induced gastrointestinal syndrome is successfully overcome in HSCT patients, long-term intestinal abnormalities in digestion and absorption caused by irradiation can still be a concern, and nutritional support therapy may be useful. The relationship between late effects such as crypt senescence, ecological imbalance in intestinal flora, and dysfunctional gut digestion and absorption in mice that have been exposed to TBI remains unknown and needs to be further explored.
CONFLICT OF INTEREST
The authors declare that there are no conflicts of interest.
FUNDING
This work was supported by the National Natural Science Foundation of China [81730086, 81572969], the CAMS Innovation Fund for Medical Sciences [CIFMS, No. 2016-I2M-1-017], the Technology and Development and Research Projects for Research Institutes, Ministry of Science and Technology [2014EG150134], the Natural Science Foundation of Tianjin [16JCQNJC13600] and the Youth Backbone Fund of IRM-CAMS [2017032].