-
PDF
- Split View
-
Views
-
Cite
Cite
Tsz K Suen, Simone J C F M Moorlag, Wenchao Li, L Charlotte J de Bree, Valerie A C M Koeken, Vera P Mourits, Helga Dijkstra, Heidi Lemmers, Jaydeep Bhat, Cheng-Jian Xu, Leo A B Joosten, Joachim L Schultze, Yang Li, Katarzyna Placek, Mihai G Netea, BCG vaccination induces innate immune memory in γδ T cells in humans, Journal of Leukocyte Biology, Volume 115, Issue 1, January 2024, Pages 149–163, https://doi.org/10.1093/jleuko/qiad103
- Share Icon Share
Abstract
Bacillus Calmette–Guérin vaccine is well known for inducing trained immunity in myeloid and natural killer cells, which can explain its cross-protective effect against heterologous infections. Although displaying functional characteristics of both adaptive and innate immunity, γδ T-cell memory has been only addressed in a pathogen-specific context. In this study, we aimed to determine whether human γδ T cells can mount trained immunity and therefore contribute to the cross-protective effect of the Bacillus Calmette–Guérin vaccine. We investigated in vivo induction of innate memory in γδ T cells by Bacillus Calmette–Guérin vaccination in healthy human volunteers by combining single-cell RNA sequencing technology with immune functional assays. The total number of γδ T cells and membrane markers of activation was not influenced by Bacillus Calmette–Guérin vaccination. In contrast, Bacillus Calmette–Guérin changed γδ T cells’ transcriptional programs and increased their responsiveness to heterologous bacterial and fungal stimuli, including lipopolysaccharide and Candida albicans, as simultaneously characterized by higher tumor necrosis factor and interferon γ production, weeks after vaccination. Human γδ T cells in adults display the potential to develop a trained immunity phenotype after Bacillus Calmette–Guérin vaccination.
1 Introduction
Immunologic memory is a feature of immune cells that allows them to mount a more robust and efficient immune response upon reencountering a pathogen. Until recently, immune memory was attributed solely to adaptive immune cells such as T and B lymphocytes. However, the striking observation in many epidemiologic studies that Bacillus Calmette–Guérin (BCG) vaccine, a live attenuated vaccine developed to protect against tuberculosis caused by Mycobacterium tuberculosis infections, results in an overall increased survival rate of vaccinated children,1–7 suggested that BCG may induce heterologous protective effects (cross-protection) against other types of infections through innate immune mechanisms. This, and similar observations in experimental models, led to breakthrough findings showing that innate immune cells such as monocytes, macrophages, and natural killer (NK) cells also “remember” a primary activation during infection and respond more effectively during reinfection with different antigens.8–12 This property has been termed the innate immune cell memory or trained immunity. Innate and adaptive immune cell memory differ, however, in their ability to respond to a secondary infection: while adaptive immune memory is antigen specific, innate immune memory is antigen independent.8,13
Induction of trained immunity primarily involves metabolic and epigenetic reprogramming, leading to stronger immune response to secondary stimuli in the myeloid cell compartment, their progenitors, and NK cells.8,14–19 While the effect of BCG-induced trained immunity persists in the human monocyte/macrophage compartment for at least 3 mo,8 long-term in vivo studies showed that BCG-induced cross-protection 1 yr after vaccination is most likely driven by heterologous interferon gamma (IFN-γ) and interleukin (IL) 17 production.9,20 BCG vaccination also generates long-lived NK memory cells capable of producing elevated levels of IFN-γ upon rechallenge,21 yet the role of NK cells in BCG-induced cross-protection is still unclear.9
Somatic rearrangement of T-cell receptors (TCRs) during T-cell development in the thymus leads to the generation of major histocompatibility complex (MHC)–restricted and highly diverse αβ T cells, as well as MHC-unrestricted, less diverse unconventional γδ T cells. γδ T cells recognize nonpeptide antigens via butyrophilin molecules22,23 and respond to stimulation in a faster manner than conventional T cells. Interestingly, γδ T cells also possess features of innate immune cells such as expression of innate-like receptors (e.g. natural killer group 2 member D and Toll-like receptors [TLRs]).24,25 Responsiveness to nonspecific pathogen- or stress-related signals allows a rapid activation of γδ T cells, an additional characteristic reminiscent of innate immune cells. Despite these unique characteristics, so far, the capacity of γδ T cells to mount immune memory has been mainly addressed in a pathogen-specific context,26–28 with no reports addressing the induction of innate immune memory in these cells.
Human γδ T cells are classified based on the Vδ chain variant of the TCR in Vδ1, Vδ2, and Vδ3 T cells. In adult human peripheral blood, γδ T cells represent 1% to 10% of leukocytes. The Vδ2 T-cell subset predominates and represents 50% to 95% of the whole γδ T-cell pool in human peripheral blood.29 Vδ2 chain mainly pairs with Vγ9 and such composed TCR recognizes microbial-derived phosphoantigens such as (E)-4-hydroxy-3-methyl-but-2-enyl pyrophosphate (HMBPP) and isopentyl pyrophosphate,30 an intermediate of cholesterol synthesis in the eukaryotes. HMBPP is a product of isoprenoid biosynthesis in the nonmevalonate pathway used by most eubacteria (including all mycobacteria) and some protozoa (e.g. Plasmodium falciparum) but not by mammalian cells. It is also a well-known antigen in M. tuberculosis infection,31 and many microorganisms that produce HMBPP can activate Vδ2 T cells.30 Considering the fact that the TCR of Vδ2 cells recognizes pathogen-associated molecular patterns, rather than specific antigens, the TCRs of these cells resemble innate immune receptors. Consistent with that, the early study by Munk et al. reported that human peripheral γδ T cells preexpanded with M. tuberculosis in vitro were able to proliferate in response to rechallenge with unrelated pathogens such as Listeria monocytogenes, group A streptococci, or Staphylococcus aureus,32 indicating the pathogen-unspecific γδ T-cell proliferating responses.
BCG vaccination induces a rapid proliferation of Vδ2 T cells with a well-established role in protection against M. tuberculosis. In nonhuman primates, the secondary administration of BCG results in enhanced proliferation of Vδ2 T cells.26M. tuberculosis stimulation of peripheral blood mononuclear cells (PBMCs) from BCG-vaccinated individuals resulted in greater expansion of Vδ2 T cells compared to nonvaccinated controls.33 Moreover, γδ T cells (most likely Vδ2 T cells) from BCG-vaccinated individuals showed increased production of IFN-γ upon in vitro stimulation with BCG.21 Taken together, these reports imply the generation of immune memory by γδ T cells during mycobacterial infection. Not only γδ T cells develop immune memory characteristics, but they are also suggested, together with NK cells, to be the main producers of IFN-γ during memory responses to secondary challenge in vaccinated infants.34 However, whether memory γδ T cells exhibit an enhanced response to heterologous stimuli needs to be investigated. In this study, we assessed whether BCG vaccination can induce heterologous immunologic memory and increased responsiveness to nonmycobacterial stimuli in Vδ2 T cells.
2 Methods
2.1 Sample collection from healthy volunteers and participants of clinical trial
BCG-vaccinated volunteers were a part of the 300BCG clinical trial approved by the Arnhem-Nijmegen Ethical Committee (approval number NL58553.091.16), performed at Radboud University Medical Center, Nijmegen, the Netherlands, according to the Declaration of Helsinki and Good Clinical Practice. Prior to inclusion, study volunteers were medically screened and provided written informed consent (Supplementary Table 1). Volunteers were not previously vaccinated with BCG before the study and did not receive any other vaccination 3 mo prior to the start of the study. All participants were vaccinated with 1 standard dose of BCG vaccination (BCG Bulgaria strain, Intervax; 0.1 mL intradermally), and blood was collected before vaccination and postvaccination (i.e. after 2 and 12 wk).
2.2 PBMC isolation and stimulation
Human PBMCs were isolated from peripheral blood of healthy volunteers by Ficoll (GE Healthcare) density gradient centrifuged. PBMC cultures were stimulated with heat-inactivated infectious agents: M. tuberculosis (2 μg/mL), Escherichia coli (106 colony-forming units [CFU]/mL), S. aureus (106 CFU/mL), Candida albicans (106 CFU/mL), and BCG (106 CFU/mL) reconstituted from lyophilized vials of commercial BCG vaccine provided by InterVax Ltd. For single-cell RNA sequencing (scRNA-seq) analysis, isolated PBMCs were stimulated for 4 h with either RPMI (control) or lipopolysaccharide (LPS) 10 ng/mL (serotype 055: B5; Sigma).
2.3 Flow cytometry
Cell surface marker staining was performed with the following antibodies: anti-human CD45 (HI30; BioLegend) Brilliant Violet 605, anti-human CD3 (UCHT1; BioLegend) Pacific Blue, anti-human TCRγδ (11F2; BD Bioscience) PE-Cy7 or PE, anti-human CD69 (FN50; BioLegend) APC-Cy7 or PE-Cy7, anti-human CD25 (M-A251; BioLegend) APC, and anti-human TCRαβ (IP26; BioLegend) APC-Cy7 at 4 °C in the dark for 30 min. The cells were then washed with phosphate-buffered saline (PBS) followed by fixation (CellFIX; BD Bioscience). Intracellular cytokine staining was performed on the cells, which were stimulated for 4 h with anti-human CD3 (1 μg/mL; BD Bioscience) and anti-human CD28 (1 μg/mL; BD Bioscience) in the presence of GolgiPlug (BD Bioscience; 1:1,000) prior to staining. PBMCs were then incubated for 30 min in cold PBS in the dark with the following antibodies against cell surface markers. Permeabilization and fixation were performed with the commercially available kit according to the manufacturer's instructions (eBioscience). After fixation and permeabilization, intracellular staining was performed with the following antibodies: anti-human perforin (dG9; BioLegend), anti-human-IL17A (BL168; BioLegend), anti-human TNF (MAb11; BioLegend) APC, and anti-human IFN-γ (B27; BD Pharmingen) PerCP-Cy5.5. After a 30-min incubation, the cells were washed with PBS and stored in CellFIX reagent (BD Bioscience) until analysis. Cells were acquired on an LSR II flow cytometer (BD Biosciences) and analyzed using FlowJo software version 10.8.0.
2.4 Single-cell library preparation and RNA sequencing of 300BCG cohort
Blood samples collected on day 0 and 3 mo post-BCG vaccination were used for the scRNA-seq analysis. In total, 156 samples were mixed into 32 pooled libraries. In each pool, an equal number of cells from 3 or 5 different donors were pooled together. Single-cell gene expression libraries were generated on the 10× Genomics Chromium platform using the Chromium Next GEM Single Cell 3′ Library & Gel Bead Kit v3.1 and Chromium Next GEM Chip G Single Cell Kit (10× Genomics) according to the manufacturer's protocol. Libraries were sequenced on a NovaSeq 6000 S4 flow cell using v1 chemistry (Illumina) with 28-bp R1 and 90-bp R2 run settings. These pools were sequenced in 3 batches in which conditions and time points were mixed to minimize potential batch effects. Sequencing data are deposited in the European Genome-Phenome Archive under the accession number EGAS00001006990.
2.5 Single-cell data preprocessing and demultiplexing
In each library, the bcf2fastq Conversion Software (Illumina) was used to convert BCL files to FASTQ files, along with a sample sheet including 10× barcodes. The proprietary 10× Genomics STAR in CellRanger pipeline (v3.1.0) was used to align read data to GRCh38/b38 (downloaded from 10× Genomics). We set the expected cell parameter to 2,000. This generated a gene expression matrix that recorded Unique Molecular Identifier (UMI) count of each gene in each cell.
To remove duplicates, cells were assigned to their origin using the souporcell (v2.0) tool using premapped bamfiles.35 In total, 91.98% cells were retrieved for downstream analysis. Since the T0 data (before vaccination) of 1 individual contained few cells at T3m (3 mo postvaccination), we discarded them from downstream analysis. Subsequently, the souporcell tool was employed to cluster cells based on allele counts using a hierarchical clustering strategy and assign individuals to clusters. Further, the consistency between the assigned individuals via souporcell and individual phenotypes’ ID was cross-checked using the genotype data set of each individual.
2.6 Single-cell data quality control, integration, and clustering
Cells were removed if they had (i) a percentage of mitochondrial genes more than 25% and (ii) a number of detected genes fewer than 100 or more than 5,000. Only genes that were expressed in at least 5 cells were considered for downstream analysis, resulting in approximately 200,000 cells and 21,975 genes.
In total, cells were collected from 3 batches. There are 10 pools in batch 1, 10 pools in batch 2, and 12 pools in batch 3. In each pool, 5 samples from different individuals at different time points with different stimulations were pooled randomly. Seurat (v4.0.0)36 package of R (v4.0.2) was used to integrate and analyze all data together. In brief, for each independent data set from each pool, UMI counts were normalized and the top 2,000 variable features were selected using the NormalizeData and FindVariableFeatures function with default parameters, respectively. Later, repeated features were then identified across all independent data sets and utilized for scaling and principal component analysis (PCA) analysis on each data set. Instead of canonical correlation analysis, reciprocal PCA was used via the SelectIntegrationFeatures function to detect integration anchors in order to speed up calculation for integration and avoid overcorrection.37 The integrated data set was then scaled and clustered using default parameters. Cell clusters were further annotated by combining the results from the SingleR38 package of R (HumanPrimaryCellAtlasData, BlueprintEncodeData, MonacoImmuneData, DatabaseImmuneCellExpressionData, and NovershternHematopoieticData were selected as reference) and the expression level of known cell markers (CD4 T cells: IL7R, CD3D; CD8 T cells: CD8A, CD8B, CD3D; NK cells: GNLY, NKG7).
2.7 Identification and clustering of γδ T cells from the 300BCG data set
After cell-type annotation, we first selected T cells and NK cells from the scRNA-seq data set and extracted γδ T cells from these populations due to their overlapping gene expression profiles (Reference to PNAS paper).39 The γδ T cells were annotated based on known their markers, including TRDC, TRGC1, TRGC2, CD3D, CD3E, GZMB, and GZMK.
The FindVariableFeatures function of the Seurat was employed to detect the top variable genes in γδ T cells and perform unsupervised clustering. In order to test the heterogeneity of γδ T cells leading by trained immunity, we calculated the changes of each gene between after being trained (3 mo after BCG vaccination, with LPS restimulation, T3m_LPS) and before begin trained (before BCG vaccination, with LPS stimulation, T0_LPS). Initially, we calculated the average expression level of each gene at T0_LPS for each individual. Then the expression level of each gene in each γδ T cell of each individual at T3m_LPS was subtracted from the corresponding average expression of that gene in the γδ T cell at T0_LPS, and this difference was defined as “TI changes.” Next, based on TI changes, unsupervised clustering employing FindNeighbors and FindClusters in the Seurat package was performed to estimate the similarity between cells. The γδ T cells were clustered into 5 clusters using the K-nearest neighbor graph and Louvain algorithm.
2.8 Identification of trained immunity response genes and enrichment analysis
Differential gene expression analysis was performed using the FindMarkers/FindAllMarkers function with Wilcoxon rank-sum test in the Seurat package. Genes that were expressed in at least 10% cells and having a P value less than 0.05 (after Bonferroni correction) were considered significant. Trained immunity response genes (TIGs) were identified by the gene expression levels at T3m_LPS and T0_LPS.
For the enrichment test, sets of significant genes were analyzed using enrichGO and enrichKEGG function (with the pAdjustMethod set to “B” and a q value cutoff of 0.05) of the ClusterProfiler (v3.18.1)40 package in R.
2.9 Cell–cell interaction analysis
NicheNet (v1.0.0)41 was used to infer cell–cell interaction in the T3m_LPS based on aggregated prior information of ligand–receptor, signaling, and gene regulatory data. In brief, communications analysis was performed between monocytes and trained γδ T subpopulations, and one-time monocytes and the γδ T subpopulation were set as “receiver” and “sender,” respectively, and other time vice versa. In each analysis, TIGs were regarded as sets of interest (targets) and ligands were identified from senders. Top 20 ligands were selected based on the Pearson correlation coefficients with TIGs. The genes expressed in at least 10% of the cells from the senders were used as the background genes.
2.10 Statistical analysis
Statistical analyses were performed with GraphPad Prism 7 (GraphPad Software). Statistical significance of differences observed was calculated by the Friedman or Mann–Whitney test as indicated in the figure description. Differences were considered significant when P value was < 0.05 (*), < 0.01 (**), < 0.001 (***), or < 0.0001 (****).
3 Results
3.1 S. aureus and C. albicans are the most potent activators of human peripheral blood γδ T cells
In order to better understand the functional responses of human peripheral blood γδ T cells, we first assessed CD25 and CD69 activation markers on γδ T cells in response to in vitro stimulation with heat-killed pathogens using flow cytometry. In our experimental setup, PBMCs from nonvaccinated healthy individuals were cultured with M. tuberculosis, E. coli, S. aureus, and C. albicans up to 96 h. By gating on the TCR γδ T-cell population within PBMCs, we found that stimulation with all 4 inactivated pathogens caused upregulation of CD69 (Fig. 1A and C) and CD25 (Fig. 1B and D) activation markers on γδ T cells as early as 24 h after stimulation. As expected, M. tuberculosis induced significant CD25 expression on γδ T cells after 24 and 96 h, but a significant expression of CD69 was found on γδ T cells only after 24 h. S. aureus induced a significant expression of the early activation marker (CD69) on γδ T cells at 24 h (but not after 96 h), while the late activation marker (CD25) was significantly expressed on γδ T cells at both time points. Notably, C. albicans induced a statistically significant increase in both early and late activation markers on γδ T cells.
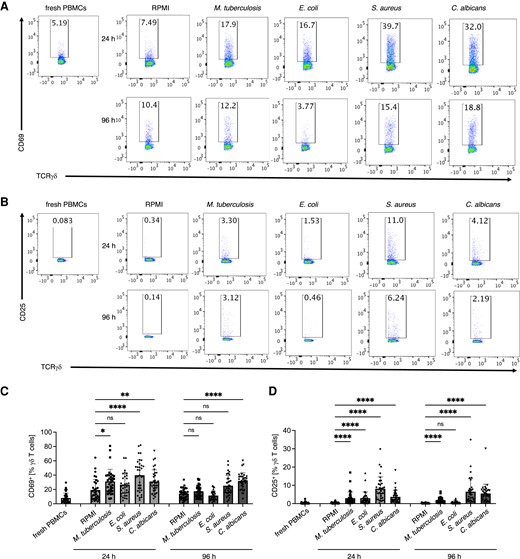
Human peripheral blood γδ T cells upregulate activation markers: CD25 and CD69 in response to M. tuberculosis, E. coli, S. aureus, and C. albicans as early as 24 h after in vitro cultures. (A) Flow cytometry analysis of CD69 expression on γδ T cells in PBMC cultures stimulated in vitro with RPMI alone or with indicated heat-killed pathogens assessed before (0 h), 24 h, and 96 h after stimulation. PBMCs were gated for CD45+CD3+TCRγδ+ cells and representative dot plots are shown. (B) Cumulative data of percentage of CD69+ among γδ T cells assessed as in A. (C) Flow cytometry analysis of CD25 expression on γδ T cells assessed as in A. (D) Cumulative data of percentage of CD25+ among γδ T cells assessed as in C. Friedman test, P value <0.05 (*), <0.01 (**), <0.001 (***), or <0.0001 (****); n = 34.
Additionally, we analyzed IFN-γ and TNF expression, which are effector molecules of γδ T cells. While we did not observe a significant increase in tumor necrosis factor (TNF) single-producing cells upon stimulation with selected pathogens (Fig. 2A and B), M. tuberculosis and C. albicans induced a significant increase in numbers of IFN-γ single-producing γδ T cells after 4 d of activation (Fig. 2A and C). IFN-γ and TNF double-producing T cells were highly induced by S. aureus and C. albicans (Fig. 2A and D). Thus, we found a distinct functional response of human γδ T cells toward the heat-killed pathogens.
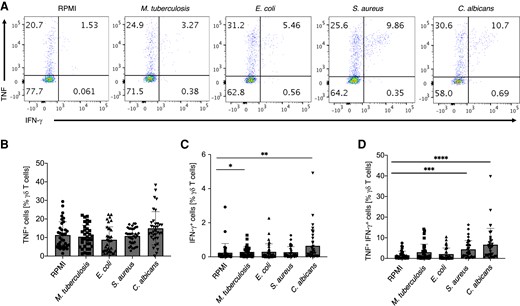
S. aureus and C. albicans are the most potent inducers of cytokine production by peripheral blood γδ T cells. Human PBMCs were stimulated as in Fig. 1. TNF and IFN-γ cytokine production by γδ T cells was assessed by intracellular staining at day 4 of culture upon restimulation with anti-CD3/CD28 for 4 h. PBMCs were gated for CD45+CD3+TCRγδ+ cells and representative dot plots are shown. (A) Representative dot plots of TNF and IFN-γ expression by γδ T cells in response to indicated stimuli. (B) Cumulative data of percentage of TNF+ IFN-γ− γδ T cells assessed as in A. (C) Cumulative data of percentage of TNF− IFN-γ+ γδ T cells assessed as in A. (D) Cumulative data of percentage of TNF+ IFN-γ+ γδ T cells assessed as in A. Friedman test, P value <0.05 (*), <0.01 (**), <0.001 (***), or <0.0001 (****); n = 34.
3.2 BCG vaccination in adults enhances perforin production by γδ T cells
To determine the functional characteristics of γδ T cells, we investigated γδ T-cell responses to various stimuli in healthy adult volunteers who had been vaccinated with BCG. As represented in a schematic diagram, we assessed γδ T-cell subset distribution and activation status before and after BCG vaccination (Fig. 3A). BCG vaccination did not affect γδ T-cell percentages in the peripheral blood of vaccinated volunteers (Fig. 3B and C). Similarly, BCG did not influence the activation status of γδ T cells 2 and 12 wk after vaccination, as characterized by the expression of CD69 (Supplementary Fig. 1A and B) and CD25 (Supplementary Fig. 1C and D). The assessment of the perforin production in PBMC cultures treated 24 h with RPMI alone revealed that perforin amounts were significantly increased in γδ T cells 3 mo after BCG vaccination (Fig. 3D and E). Therefore, BCG vaccination has no effect on activation status of human γδ T cells in a steady state but likely can boost their effector function by enhancing cytotoxic molecule production.
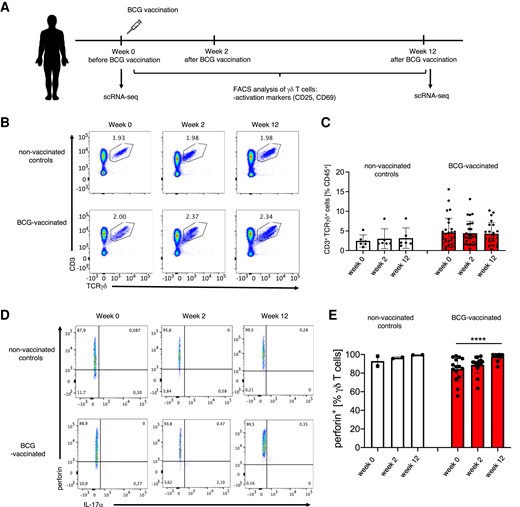
BCG vaccination in adults enhances perforin production by γδ T cells. (A) Schematic representation of the experimental setup for γδ T-cell characterization in BCG-vaccinated volunteers. (B) Flow cytometry analysis of CD3+TCRγδ+ T cells among CD45+ cells in peripheral blood of unvaccinated controls and BCG-vaccinated volunteers before (week 0) vaccination and 2 wk (week 2) and 3 mo (week 12) after vaccination; gated CD45+ cells. (C) Cumulative percentages of CD3+TCRγδ+ T cells assessed as in B. (D) Representative flow cytometry dot plots. (E) cumulative percentages of perforin-producing γδ T cells (nonvaccinated controls, n = 2; BCG vaccinated, n = 15).
3.3 BCG vaccination induces transcriptional changes in γδ T cells
To further determine transcriptional changes induced in γδ T cells by the BCG vaccine, sRNA-seq was performed on PBMCs collected from 39 healthy volunteers. These cells were collected before and 3 mo after receiving the BCG vaccination, both with and without in vitro LPS stimulation. In total, we profiled ∼200,000 PBMCs after filtering out doublets, followed by quality control. To identify γδ T cells from the scRNA-seq data set, we first selected CD4 T cells, CD8 T cells, and NK cells and visualized those cell types in UMAP (Fig. 4a). Based on manually curated markers, cluster 7 was annotated as γδ T cells with a high expression level of TRDC, TRGC1, TRGC2, GZMB, and GZMK (Fig. 4B and 4C). In total, 6,338 γδ T cells were obtained with 1,664, 1,754, 1,525, and 1,395 cells from four conditions: before BCG vaccination without/with LPS (T0_RPMI, T0_LPS) and 3 mo after BCG vaccination without/with LPS stimulation (T3m_RPMI, T3m_LPS), respectively.
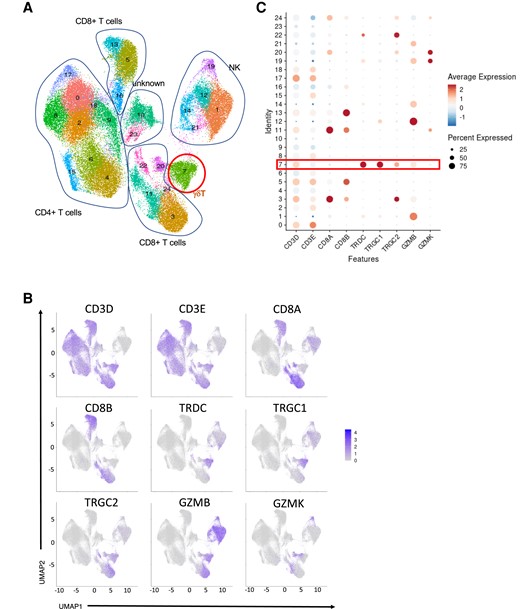
Overview of γδ T cells in the 300BCG data set at day 0 before BCG vaccination and 3 mo postvaccination. (A) UMAP of CD4T, CD8T, and NK cells from 300BCG. (B) FeaturePlot of γδ T marker (CD3D, CD3E, TRDC, TRGC1, TRGC2, GZMB, GZMK) expression distribution. (C) γδ T marker expression level in each cluster.
We next used an unsupervised clustering method and identified 5 distinct subpopulations of trained γδ T cells (Fig. 5A). Samples collected before BCG and 3 mo after BCG vaccination with LPS stimulation were used for downstream analysis. Interestingly, IFNG was highly expressed in cluster 2 as one of the main markers (Fig. 5B). In order to explore the trained immunity features of identified γδ T-cell subpopulations, we calculated TIGs by comparing gene expression levels between T3m_LPS and T0_LPS (Fig. 5c). Cluster 1 showed the greatest number of TIGs, with 80% of them being downregulated. Although cluster 0 had the highest number of cells, it had the least number of identified TIGs compared to the other clusters, which may indicate that the majority (67%) of γδ T cells were not strongly trained (Fig. 5A and C).
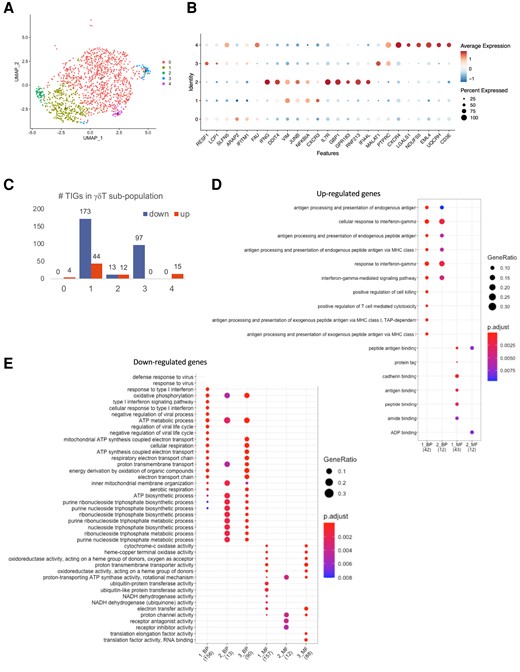
Trained immunity characteristics of γδ T cells. (A) UMAP γδ T-cell subpopulation after being trained by BCG assessed 3 mo postvaccination. (B) Markers of identified subpopulations. (C) The number of TIGs in each subpopulation. (D) GO enrichment of upregulated TIGs. (E) GO enrichment of downregulated TIGs.
To further investigate the functions of the identified TIGs, Gene Ontology (GO) and Kyoto Encyclopedia of Genes and Genomes (KEGG) enrichment analyses were performed based on the up- and downregulated TIGs in each cluster (Fig. 5D and E and Supplementary Fig. 2). GO analyses of the upregulated genes in cluster 1 and cluster 2 identified GO terms related to “response to IFN-γ” and “IFN-γ mediated signaling” (Fig. 5D). The KEGG pathway analyses showed that upregulated TIGs in cluster 1 and cluster 2 were enriched in the “antigen processing and presentation” pathway (Supplementary Fig. 2A). In contrast, the downregulated TIGs in γδ T-cell cluster 1 were enriched in the “NOD-like receptor signaling” pathway (Supplementary Fig. 2B).
Next, we tested if γδ T cells interact with monocytes during the training process through cell–cell communication analyses. Monocytes are well known to undergo metabolic and epigenetic reprogramming by BCG, resulting in a trained immunity phenotype8,17,42 as well as being potent activators of γδ T cells,43–45 raising the possibility that the interaction between the 2 cell types is important for the formation of trained immunity. Therefore, we explored the interaction pairs where monocytes express the ligand and γδ T-cell subpopulations express the receptor (Fig. 6A–D). This analysis predicted that the CXCL family from monocytes were highly ranked ligands, which likely affected the TIGs in γδ T cluster 1 (Fig. 6A). Meanwhile, IL family from monocytes were potential ligands to regulate receptors (TIGs) in γδ T cluster 2 (Fig. 6b). IFNG, as one of the receivers, was involved in the crosstalk between monocytes and γδ T-cell cluster 2. Combined with the GO analyses (Fig. 5D), the upregulated TIGs were enriched in IFN-γ–related biological processes, suggesting that IFNG played an important role in γδ T-cell impact on monocytes during induction of trained immunity. Additionally, we focused on the interaction in which γδ T-cell subpopulations express the ligand, while monocytes express the receptor (Fig. 6C and D). We observed that IFNG was highly expressed in cluster 2 and interacted with all the identified TIGs in monocytes. These analyses strongly suggest that after BCG vaccination, a subset of γδ T cells (but not all) displays an increase in responses to heterologous (nonmycobacterial) stimuli suggestive of induction of trained immunity, and 2 of the γδ T-cell subpopulations that possess features of trained immunity were related to IFN-γ–related pathways.
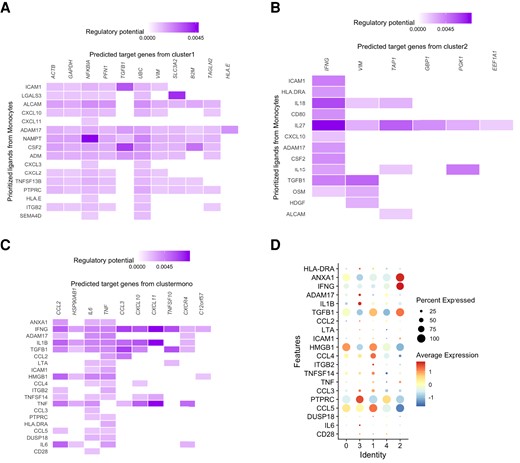
Putative γδ T-cell interactions with monocytes assessed by scRNA-seq in PBMC cultures 3 mo after BCG vaccination. (A) Cell–cell interaction between monocytes (senders) and γδ T cluster 1 (receiver). (B) Cell–cell interaction between monocytes (senders) and γδ T cluster 2 (receiver). (C) Cell–cell interaction between γδ T subpopulation (senders) and monocytes. (D) The expression level of potential ligands in each γδ T-cell subpopulation.
3.4 BCG vaccine enhances human γδ T-cell responses to heterologous stimuli
We next compared the γδ T-cell responses to in vitro stimulation in subjects before and after BCG vaccination (Fig. 7). We assessed the expression of activation markers CD25 and CD69 (data not shown) on γδ T cells, as well as cytokine production in response to the in vitro stimuli of PBMCs isolated from adults before (week 0) and 2 wk (week 2) and 12 wk (week 12) after BCG vaccination and compared to nonvaccinated controls (Fig. 7A). The fold change of CD69+ and CD25+ γδ T cells in response to all stimuli before and after vaccination was not different in BCG-vaccinated vs nonvaccinated controls (Supplementary Fig. 3). In contrast, the fold change of IFN-γ–producing γδ T cells in response to M. tuberculosis in BCG-vaccinated volunteers 2 wk after vaccination was significantly increased compared to nonvaccinated controls, indicating the generation of specific immune memory by γδ T cells (Fig. 7B).
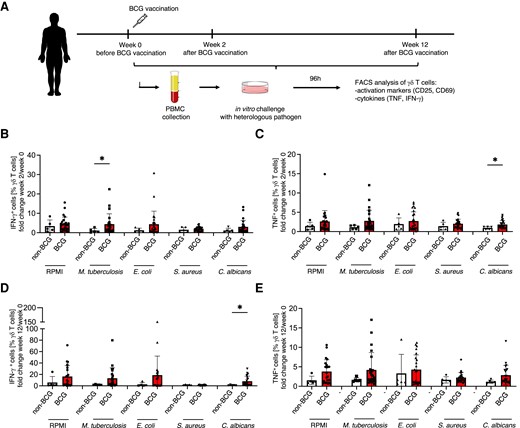
BCG vaccination displays a tendency to induce innate immune memory in peripheral blood γδ T cells. (A) Experimental layout of the BCG vaccination trial. (B) Fold change of TNF-producing γδ T cells 2 wk after BCG vaccination over week 0 before vaccination. (C) Fold change of IFN-γ–producing γδ T cells 2 wk after BCG vaccination over week 0 before vaccination. TNF+ and IFN-γ+ γδ T cells were assessed by flow cytometry 96 h after in vitro culture with indicated pathogens or RPMI medium alone. (D) Fold change of IFN-γ–producing γδ T cells 12 wk after BCG vaccination over week 0 before vaccination. (E) Fold change of TNF-producing γδ T cells 12 wk after BCG vaccination over week 0 before vaccination. IFN-γ+ and TNF+ γδ T cells were assessed by flow cytometry 96 h after in vitro culture with indicated pathogens or RPMI medium alone. Mann–Whitney test, *P < 0.05. Nonvaccinated volunteers, n = 6; BCG-vaccinated volunteers, n = 26.
The heterologous responses of γδ T cells to nonmycobacterial stimuli followed a pattern previously described in BCG vaccination studies, in which only approximately half of the volunteers respond with a potent trained immunity phenotype.46,47 The TNF and IFN-γ production capacity of γδ T cells after stimulation with E. coli and S. aureus was increased in 30% to 50% of the BCG-vaccinated individuals compared to controls (Fig. 7B and E). BCG vaccination significantly increased the TNF and IFN-γ production stimulated by C. albicans (Fig. 7C and D).
4 Discussion
Understanding the biological mechanisms and immune cell subsets involved in the induction of immunologic memory is essential for the design and development of effective vaccines. For decades, immunologic memory was solely attributed to adaptive immune cells such as conventional T and B cells. Subsequently, these cells became the main target for vaccination strategies. The recent discovery that other cells of the immune system such as myeloid cells (monocytes, macrophages, neutrophils), NK cells, and innate-lymphoid cells can mount an enhanced response upon secondary stimulation48 has opened a new avenue for the design of preventive measures against infections. Human γδ T cells have been long known to develop memory characteristics.21,26,33 Despite the fact that they possess innate immune features (rapid and MHC-unrestricted activation, recognition of nonpeptide antigens, and expression of innate-like receptors), γδ T-cell memory has been mainly described in the pathogen-specific context,26 with a very limited understanding about the nonspecific character of memory γδ T cells.49 In this study, we address for the first time whether human peripheral blood γδ T cells can also display memory responses to heterologous stimuli (trained immunity characteristics) after BCG vaccination.
BCG vaccination did not induce changes in γδ T-cell proportion 2 and 12 wk after vaccination. While various reports showed that γδ T cells expand in peripheral blood upon various types of infections50 such as tularemia,51,52 listeriosis,53 ehrlichiosis,54 toxoplasmosis,55 malaria,56,57 leishmaniasis,58 and Salmonella infections,59 there is no consistency between studies on M. tuberculosis infections. Some reports suggest an increased number of peripheral blood γδ T cells during the disease,60,61 while other studies were not able to reproduce these findings.62–65 Studies in macaques show that Vδ2 T cells indeed expand in peripheral blood upon BCG inoculation, with a sharp peak of expansion around days 14 to 30 and a decrease to steady-state cell numbers by day 40.26,66 Intradermal inoculation of BCG in nonhuman primates slightly expands γδ T cells in peripheral blood only at high doses (100 times higher than used in our study).66 It is therefore not unexpected that regular intradermal BCG vaccination as performed in our study did not induce human γδ T-cell proliferation. This is similar to tularemia in which Francisella tularensis drives γδ T-cell expansion, while the tularemia vaccination does not.52
In contrast to the lack of an effect on the total number of γδ T cells, BCG vaccination resulted in increased production of the cytotoxic molecule perforin by γδ T cells, suggesting a functional reprogramming of these cells. This is in line with earlier studies that have shown that BCG can increase perforin production in in vitro PBMC cultures, bladder tissue in patients with cancer undergoing BCG therapy,67 and peripheral immune cells of vaccinated infants.68 However, to our knowledge, this is the first report showing the effect of BCG vaccination on perforin expression in human γδ T cells. Moreover, scRNA-seq of circulating immune cells stimulated before and 12 wk after BCG vaccination clearly shows strong changes in the transcriptional programs of γδ T cells. Interestingly, we identified 5 distinct γδ T-cell subpopulations when assessed based on the trained immunity responses. While cluster 0 contained the highest number of γδ T cells, this was also the cluster that showed the least changes after vaccination: this indicates that most (approximately two-thirds) of the γδ T cells are not trained. This is different from other innate immune cell types that are more potently trained after BCG vaccination (e.g. approximately three-quarters of monocytes undergo different trained immunity programs).69 In contrast, trained immunity is induced in a smaller proportion of γδ T cells. While cluster 1 showed the greatest number of TIGs, 80% of them were downregulated after BCG vaccination. A very interesting pattern has been subsequently seen in cluster 2 of γδ T cells, in which IFNG was highly expressed after BCG vaccination, as one of the main markers. In line with this, GO analyses of the upregulated genes in cluster 1 and cluster 2 identified GO terms related to “response to IFN-γ” and “IFN-γ mediated signaling.” Consistently, a recent scRNA-seq analysis of in vitro BCG-treated PBMC cultures revealed an upregulated IFN-γ signature within γδ T cells.70 KEGG pathway analyses showed that upregulated TIGs in cluster 1 and cluster 2 were enriched in the “antigen processing and presentation” pathway. These clusters might represent previously described γδ T cells with antigen-presenting properties.66,67,71,72 The Vδ2 T cells pulsed with M. tuberculosis purified protein derivative acquired antigen-processing molecules and were able to induce proliferation of conventional αβ T cells in vitro.66,67 Monocyte–γδ T-cell interaction analysis also predicted that IFNG played an important role in γδ T-cell impact on monocytes during induction of trained immunity. Previous studies have indicated that mycobacteria-infected human monocytes are potent in activating γδ T cells by an efficient antigen presentation.65,66 Upon M. tuberculosis stimulation, antigen presentation and costimulation exerted by monocytes are also essential for triggering IFN-γ production by γδ T cells.73 Here, our data confirmed the importance of this interaction not only for γδ T-cell activation but also for the induction of γδ T-cell trained immunity. In addition, we report for the first time a list of specific ligands that may induce such interaction. Because of the presence of various immune cells in the PBMC cultures, we do not exclude the possibility that interactions with other cells are important for the induction of trained immunity in γδ T cells. The identification of the molecular mechanism of trained immunity induction by these interactions requires further investigation. Altogether, the scRNA-seq analysis strongly suggested induction of a trained immunity program in γδ T cells, with the IFN-γ pathway likely to play an important role in their enhanced function.
The functional assessment of γδ T-cell responses supports this observation. Stimulation of PBMC cultures with heterologous heat-killed pathogens showed significantly increased numbers of IFN-γ–producing γδ T cells 2 wk after BCG vaccination, supporting the induction of a trained immunity phenotype of γδ T cells. BCG-expanded γδ T cells may be also effective in lysing cancer cells, as suggested by the recent report on in vitro BCG-treated PBMC cultures, indicating a secondary responses to heterologous stimuli.70 It has to be noted, however, that an effective induction of trained immunity after bacterial stimulation was observed only in 30% to 50% of the individuals, similar to earlier observations during BCG vaccination in experimental models of malaria74 or yellow fever vaccination.47 In contrast, significant increases in TNF- and IFN-γ–producing γδ T cells have been observed in response to C. albicans stimulation. The smaller effect of BCG vaccination on γδ T cells in this study might be due to the adult age of the trial participants, as it is debated whether the BCG vaccine has a weaker effect in adults than in children.75,76 Consistent with that hypothesis, in vitro BCG stimulation of PBMCs from BCG-vaccinated children leads to a robust expansion of γδ T cells.77 Furthermore, comparative studies show that γδ T-cell memory responses are more significant in individuals vaccinated at a neonatal age than in adulthood.34 It is therefore of great importance to address the memory formation potential of γδ T cells in newborns in order to understand the heterogeneity of the efficacy of the BCG vaccine.
One additional important aspect to be considered is also the use of different strains of the BCG vaccine, with considerable differences reported between the immunomodulatory effects of various BCG strains.78 The BCG-Bulgaria strain used in our study, which is similar to BCG-Russia used in 70% of the countries worldwide, showed a weaker effect on cytokine production in whole blood cell cultures than BCG-Denmark,79 and this should be considered in the interpretation of our results. Indeed, scRNA-seq analysis of PBMC cultures treated in vitro with 2 different strains of BCG—namely, iBCG (BCG-Moreau) and BCG-Tice (OncoTICE)—revealed distinct effects on NK and γδ T-cell populations.70 While iBCG was potent in activating and expending γδ T cells, BCG had a boosting effect on NK cells. Future studies are warranted to assess the impact of other BCG strains on γδ T cells.
It needs to be noted that we addressed γδ T-cell responses in terms of cytokine production, while most of the reports address proliferative responses. As both are an indication of effector function, our data suggest that the 2 processes are uncoupled. Because of sample limitation, we focused our analysis on cytokine production as we believe it is of bigger relevance for the effector function. An additional limitation of the study is that our experimental setup did not include the discrimination between Vδ1 and Vδ2 γδT cells. However, because most γδ T cells in human peripheral blood are Vδ2 T cells,29 which are also the subpopulation responding to BCG, we believe that the observed effect of vaccination on the γδ T-cell population is mainly attributed to Vδ2 T cells.
Interestingly, we found that S. aureus and C. albicans are potent activators of human peripheral blood γδ T cells based on CD69 and CD25 upregulation, as well as TNF and IFN-γ expression. While mouse γδ T cells are known to be involved in the defense against S. aureus80–87 and C. albicans infections,83,84,88,89 there are few studies addressing human γδ T-cell activation by these pathogens.32,85–87 These studies reveal a proliferative response of human γδ T cells to S. aureus stimulation32 and, in line with our findings, IFN-γ production, mainly by Vδ2 T cells.90 However, this study reveals a strong proliferative response and a strong upregulation of IFN-γ and IL-17 of Vδ1 but not Vδ2 T cells to C. albicans in vitro.91,92 Whether the cytokine-producing γδ T cells are Vδ1 or Vδ2 in our cultures has not been addressed and remains to be determined. Future studies should also investigate the molecules (HMBPP, β-glucans, TLR ligands, etc.), receptors, and signaling pathways involved in γδ T-cell stimulation by these microorganisms. As increasing evidence is pointing to the role of γδ T cells in the immune response against S. aureus and C. albicans infection in humans, others’ and our observations pave the way for investigation of the function of these cells in candidiasis and Staphylococcus infections and the development of new therapeutic approaches.
In conclusion, we report induction of a trained immunity phenotype in γδ T cells in vivo in adults, although large interindividual variations are present. Because newborns contain truly naive γδ T cells, conducting a similar assessment in cord blood or blood from young children will provide the answer to whether human γδ T cells can develop stronger innate immune memory in childhood. Furthermore, our human study is limited in the capacity to provide causality proof due to inherent ethical limitations and can only characterize γδ T-cell trained immunity responses. However, based on the molecular mechanisms known to drive trained immunity phenotype in myeloid cells,42 one can speculate that metabolites such as HMBPP produced by BCG and known to activate human peripheral γδ T cells93,94 or other BCG components induce metabolic and epigenetic reprograming of these cells. As a result of metabolic and epigenetic changes induced by a primary trigger, the cells are in a more poised state to respond. Therefore, upon the secondary stimulation by unrelated pathogens/their components, γδ T cells produce elevated levels of effector molecules. These could be driven by the γδ TCR signaling but also by innate-like receptors such as TLRs present on the surface of γδ T cells.95 Importantly, we did find that S. aureus and C. albicans are very potent stimulators of human peripheral blood γδ T cells. This observation might be of clinical relevance as candidemia is a major cause of morbidity and mortality in health care settings,96 while staphylococcal infections are an increasing threat due to the emergence of multidrug-resistant strains.97 Whether γδ T cells can be a new therapeutic or diagnostic tool to fight these infectious needs further investigation.
Authorship
Conceptualization: M.G.N., J.L.S., L.A.B.J., and K.P.; methodology: T.K.S., J.B., and K.P.; investigation: T.K.S., K.P., L.C.J.deB., S.J.C.F.M.M., V.A.C.M.K., V.P.M., H.D., and H.L.; data curation: L.C.J.deB., S.J.C.F.M.M., V.A.C.M.K., and V.P.M.; formal analysis: T.K.S., K.P., and W.L.; resources: M.G.N., L.A.B.J., and Y.L.; writing—original draft preparation: K.T.S. and K.P.; writing—review and editing: M.G.N., J.L.S., J.B., L.C.J.deB., S.J.C.F.M.M., V.A.C.M.K., V.P.M., W.L., C.J.X., and L.A.B.J.; supervision: M.G.N. and K.P.; project administration: K.P.; funding acquisition: M.G.N., J.L.S., Y.L., and K.P. All authors have read and agreed to the published version of the manuscript.
Supplementary material
Supplementary materials are available at Journal of Leukocyte Biology online.
Funding
M.G.N. was supported by the European Union (ERC Advanced Grant, #833247) and a Spinoza Grant of the Netherlands Organization for Scientific Research by the Dutch Research Council (NWO). K.P. has received funding from the European Union’s Horizon 2020 research and innovation program under the Marie Skłodowska-Curie grant agreement No. 798582. This work was also supported by the German Research Foundation (DFG) to J.L.S. and M.G.N. (EXC2151/1 ImmunoSensation2—the immune sensory system, project number 390873048). Y.L. was supported by the European Union (ERC Starting Grant, #948207), NWO ASPASIA Grant, and Radboud University Medical Centre Hypatia Grant.
References
Abbreviations
- BCG
Bacillus Calmette–Guérin
- CFU
colony-forming unit
- HMBPP
(E)-4-hydroxy-3-methyl-but-2-enyl pyrophosphate
- IFN
interferon
- IL
interleukin
- LPS
lipopolysaccharide
- MHC
major histocompatibility complex
- NK
natural killer
- PBMC
peripheral blood mononuclear cell
- TCR
T-cell receptor
- TLR
Toll-like receptor
- TNF
tumor necrosis factor
Author notes
Katarzyna Placek and Mihai G. Netea contributed equally.
Conflict of interest statement. L.A.B.J. and M.G.N. are scientific founders of TTxD and Lemba. All authors claim no conflict of interest.