-
PDF
- Split View
-
Views
-
Cite
Cite
Roselle A Herring, Iain Parsons, Fariba Shojaee-Moradie, Mary Stevenage, Nicola Jackson, Ralph Manders, A Margot Umpleby, Barbara A Fielding, Melanie Davies, David L Russell-Jones, Effect of Dapagliflozin on Cardiac Function and Metabolic and Hormonal Responses to Exercise, The Journal of Clinical Endocrinology & Metabolism, Volume 108, Issue 4, 1 April 2023, Pages 888–896, https://doi.org/10.1210/clinem/dgac617
- Share Icon Share
Abstract
This work aimed to investigate the effect of the SGLT2 inhibitor, dapagliflozin (DAPA), on cardiac function and the metabolic and hormonal response to moderate exercise in people with type 2 diabetes.
This was a double-blind, placebo-controlled crossover study with a 4-week washout period. Nine participants were randomly assigned to receive either 4 weeks of DAPA or 4 weeks of placebo. After each treatment, they underwent an exercise protocol with 2 consecutive 10-minute stages at a constant load corresponding to 40% and 70% maximal oxygen consumption (VO2max), coupled with hormonal and metabolic analysis. A blinded transthoracic echocardiogram was performed 3 days later.
During the exercise protocol, glucose and lactate were lower (P < .0001 and P < .05, respectively) and β-hydroxybutyrate (BOBH) and growth hormone (GH) were higher (P < .0005 and P = .01) following DAPA treatment compared to placebo. There was a trend for lower insulin with DAPA. Adrenalin, noradrenalin, and glucagon were not different. Following DAPA participants demonstrated an increased mean peak diastolic mitral annular velocity (e’) in comparison to placebo (P = .03). The indexed left atrial volume and right ventricular e” were reduced following DAPA compared with placebo (P = .045 and P = .042, respectively). Arterial stiffness was not different between treatments (DAPA 9.35 ± 0.60 m/s; placebo 9.07 ± 0.72 m/s).
During exercise, GH may be more important than catecholamines in driving the shift from glucose to fatty acid metabolism by SGLT2 inhibitors. The 4-week crossover design showed changes in cardiac function were rapid in onset and reversible.
Diabetic cardiomyopathy is commonly due to left ventricular (LV) diastolic dysfunction, which, like cardiovascular disease and other comorbid factors such as aging, hypertension, and obesity, is a contributor to the development of heart failure (1). This is apparent for patients with a reduced ejection fraction and those with a preserved ejection fraction. SGLT2 inhibitors are a class of drug that are insulin independent and promote urinary glucose excretion to lower blood glucose levels, sustain weight loss, and improve glycemic control when used as a monotherapy (2) or in conjunction with other glucose-lowering medications (3-5) including insulin (6). Multiple clinical trials with SGLT2 inhibitors in type 2 diabetes have shown positive cardiovascular outcomes (7-10), and further trials have demonstrated that these beneficial outcomes also translate to patients without type 2 diabetes (11).
The underlying mechanism for the improved cardiovascular outcomes remains unclear, but the beneficial effects in people without diabetes suggest it is independent of improved blood glucose control. Experimental and clinical data have suggested that the glucosuria and natriuresis simulate an osmotic diuretic effect resulting in reduced plasma volume (12). Other possible mechanisms include a reduction in sympathetic activation, modulation of intracytoplasmic Na + in the cardiomyocyte, SGLT2 inhibitor–induced erythropoiesis (13, 14), and improved myocardial energetics due to increased availability of β-hydroxybutyrate (BOHB), a fuel source in heart failure (15).
The aim of this study was to further investigate SGLT2 inhibitors’ potential mechanisms of action by comparing metabolic, hormonal, and cardiovascular responses to submaximal exercise as well as assessing resting cardiovascular function.
Materials and Methods
The study was a randomized, double-blind, placebo-controlled crossover trial of people with type 2 diabetes at the Cedar Centre, Royal Surrey NHS Foundation Trust, UK. Ethics approval was granted from the HRES committee: Southern Central Berkshire B. The clinical trial was registered with the European Clinical Trials Database (EudraCT) under number 2016-004878-17 and ClinicalTrials.gov NCT 04219124. The study was funded by Astra Zeneca Ltd.
Inclusion criteria were diagnosis of type 2 diabetes for more than 12 months, single-, dual-, or triple-therapy glucose-lowering agents comprising sulfonylureas, biguanides, and DDP-IV but no previous exposure to SGLT2 inhibitors, aged 18 to 75 years, body mass index less than 40, and glycated hemoglobin A1c greater than or equal to 6.5% (48 mmol/mol) and less than 9% (75 mmol/mol) within 1 month of screening. Exclusion criteria included proliferative retinopathy requiring acute treatment within the previous 3 months, moderate to severe renal impairment (creatinine clearance < 60 mL/min or estimated glomerular filtration rate < 60 mL/min/1.73 m2), severe hepatic impairment, New York Heart Association class III to IV cardiac failure, uncontrolled cardiac arrhythmia, uncontrolled hypertension, mental incapacity, pregnancy or breastfeeding, those of childbearing potential not taking adequate contraception precautions, and suspected allergy to trial products.
Design
Participants gave written informed consent. Following screening and randomization, participants received either 10 mg daily dapagliflozin (DAPA) or placebo (PLACEBO) for 28 days before a submaximal exercise test and a transthoracic echocardiogram and measure of pulse wave velocity. After a 28-day washout period, they crossed over to the other treatment and the testing was repeated (Fig. 1). They were made aware of potential changes in glycemic control and were asked to record trial medication administration, administration of any concomitant medication, hypoglycemia frequency (defined as capillary glucose level <4 mmol/L), and any adverse events.
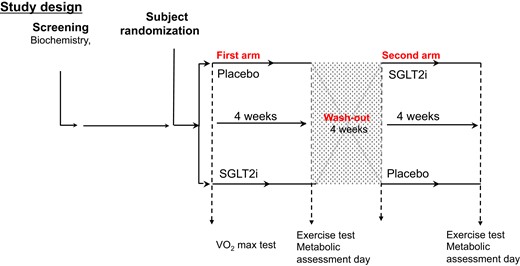
Exercise Protocol
Before the first intervention period, V̇O2peak (L.min−1) was determined from an incremental test to volitional exhaustion on a cycle ergometer (Lode; Excaliber Sport) using a breath analyzer UltimaCardiO2 (Medical Graphics, MGC diagnostics UK Ltd) in temperate laboratory conditions. This provided a baseline of finest level and variability.
Participants had a standardized meal the evening before the exercise study and then fasted overnight before arrival at the research unit. On arrival, an intravenous cannula was inserted into an antecubital vein and baseline blood samples (discussed next) were taken (time 0). Participants performed a 5-minute warm-up at 50 W. The submaximal exercise protocol consisted of 2 consecutive 10-minute stages at a constant load corresponding to 40% and 70% maximal oxygen consumption (VO2max) followed by 5 minutes’ warm down. Plasma nonesterified fatty acid concentrations (NEFA), growth hormone (GH), glucose, β-hydroxybutyrate (BOHB), noradrenaline, and adrenaline were taken at baseline (discussed earlier) 5-minute (end of warm-up), 10-minute (40% VO2max), 25-minute (end 70% VO2max), 30-minute (end of warm down), and 60-minute (postexercise) time points.
Heart rate (HR) and electrocardiography was measured continuously as was VO2 and CO2 with a Cardio2 gas exchange system (Medical Graphics). VCO2 and expired VCO2 were recorded at 5-, 10-, 25-, 30-, and 60-minute intervals along with HR, systolic blood pressure, respiratory rate, rate pressure product, VO2, expired VCO2, and respiratory exchange ratio (RER). Fat and carbohydrate oxidation were calculated from the measurements of oxygen consumption and carbon dioxide production, as described by Ferrannini (16).
Echocardiography and Pulse Wave Velocity
A transthoracic echocardiogram (TTE) (CX50, Phillips, USA) was undertaken 2 to 3 days after the exercise testing. TTEs were performed by a blinded British Society of Echocardiography–accredited practitioner (IP). Echocardiography measures were performed in the resting partial left decubitus position with the couch head at 30° elevation (flat for subcostal views), satisfying the requirements of the British Society of Echocardiography minimum data set (17). Resting HR was averaged over the parasternal long axis windows by 3-lead electrocardiogram. The left atrial (LA) and right atrial (RA) volumes were calculated by biplane area length (18, 19). LV mass was calculated using the Deverux method (20).
Arterial stiffness was assessed by measuring pulse wave velocity (PWV) between the carotid and femoral artery, using the Vicorder apparatus (Vicorder, SMT Medical GmbH & Co) at a separate visit.
At the end of the study, participants were prescribed the investigational medicinal product or placebo required for the second arm of the study depending on the randomization code, to be taken after the washout period. The protocol (exercise study, PWV, and echocardiogram) were repeated after 28 days’ treatment.
Plasma Measurements
Plasma glucose concentrations were measured with a Horiba Medical Pentra C400 analyzer using the ABX Pentra glucose kit (Horiba ABX) and BOHB concentrations using Randox kits (Glycerol and RANBUT; Randox Laboratories Co). Plasma NEFA concentrations were measured using an enzymatic kit from (WAKO Chemicals GmbH).
Glucagon was measured by radioimmunoassay with a kit from Merck Millipore (Merck Chemicals). Insulin was measured by an enzyme-linked immunosorbent assay (ELISA) from DRG Instruments GmbH, GH with an radioimmunoassay kit from DRG Diagnostics, catecholamines with ELISA kits from IBL International, GmbH, all supplied by IDS PLC.
Statistics
This study was part of a larger metabolic study of which the primary end point was the plasma BOHB concentration at the end of a meal study (21). The trial was terminated 9 participants enrolled due to the COVID-19 pandemic. Due to the exploratory nature of the exercise study, no power calculations were performed.
All measures were assessed for normality using the Shapiro-Wilk test before data analysis. Exercise measurements: Resting and exertional blood measures and cardiovascular measures were analyzed using a 2-way analysis of variance (mixed-effects model to account for any partially complete data). Baseline measurements (prior to exercise) were analyzed with a paired t test. Values were expressed as mean and SEM. For TTE data, a Cohen D effect size (ES), with 95% CIs, was calculated for each echocardiographic variable. The ES was estimated using a threshold scale where an ES of less than 0.2 was considered trivial; 0.2 to 0.49, a small effect; 0.5 to 0.79, a moderate effect; 0.8 to 1.39, a large effect; and 1.4 or greater, a very large effect. A paired t test of TTE parameters was performed for parametric, Mann-Whitney U for nonparametric, to confirm statistical significance, where the ES 95% CI did not cross zero or the ES was greater than 0.5. The α level was set to .05. All statistical analyses were performed using GraphPad Prism 8.0 (GraphPad Software).
Results
Nine participants completed the study (age: 62.0 ± 3.7 years, body mass index: 28.9 ± 1.0, glycated hemoglobin A1c: 7.7 ± 0.2% [60.2 ± 2.5 mmol/mol], 3 women/6 men). The mean duration of diabetes was 8.8 ± 0.9 years and ethnicity included 8 White and 1 Asian individuals. All participants were on metformin, 5 were taking sulfonylureas, of whom 4 were also taking DPP4 inhibitors. Seven participants were taking statins. Seven participants completed the full exercise protocol. One participant had hypotension and therefore could not sit on the bike to exercise and the other had cannulation difficulties preventing blood sampling at some time points. All patients had no known atherosclerotic cardiovascular disease or heart failure. All participants had sedentary lifestyles.
Exercise Protocol
Before exercise there were no significant differences in metabolite and hormone measurements except for BOHB, which was significantly higher with DAPA (P = .036). Plasma metabolite and hormone data at rest, during exercise, and post exercise are shown in Figs. 2 and 3. Following DAPA treatment, glucose and lactate were lower (P < .0001 and P < .05, respectively) and BOHB and GH were higher (P < .0005 and P = .01) than after placebo. Although insulin was lower with DAPA, it did not achieve statistical significance (P = .142). NEFA, noradrenalin, and adrenalin were not different between treatments.
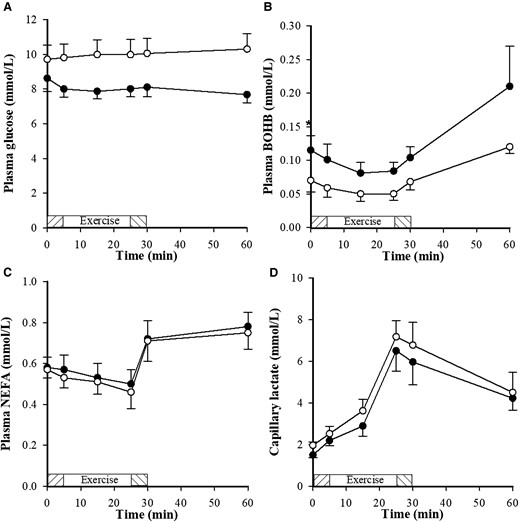
Metabolite concentrations (glucose, BOHB, NEFA and lactate), following dapagliflozin (•) and placebo (o), in response to a submaximal exercise protocol with a 5 minutes warm up at 50 W (shaded exercise bar), two consecutive 10 minutes stages at a constant load corresponding to 40% and 70% VO2max (open exercise bar) followed by 5 minutes warm down (shaded exercise bar); n=7. Between 0 and 60 minutes glucose and lactate were lower (P < .0001, P < 0.05, respectively) and BOHB was higher with dapagliflozin (P = 0.0005). *<0.05 significance.
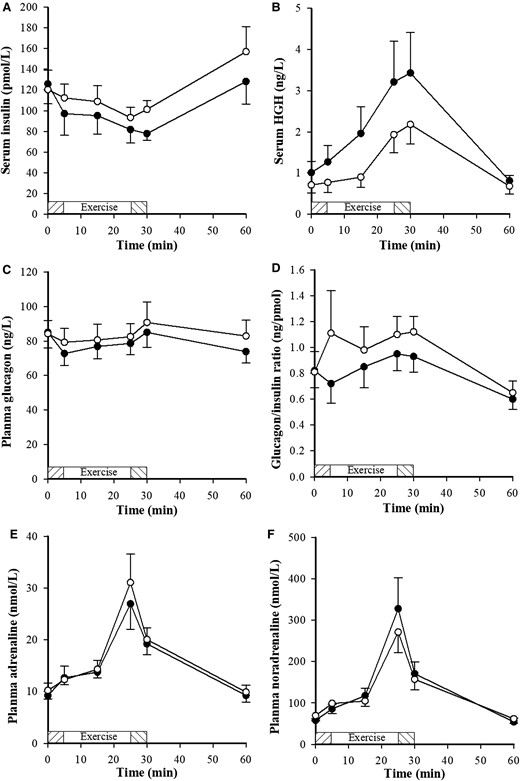
Hormone concentrations, following dapagliflozin (•) and placebo (o), in response to a submaximal exercise protocol with a 5-minute warm-up at 50 W (shaded exercise bar), 2 consecutive 10-minute stages at a constant load corresponding to 40% and 70% maximal oxygen consumption (VO2max) (open exercise bar) followed by 5 minutes warm down (shaded exercise bar); n = 7. Between 0 and 60 minutes, growth hormone (GH) was higher (P = .01) with dapagliflozin.
During the exercise protocol (0-60 minutes), there was no significant difference between treatments in HR, systolic blood pressure, respiratory rate, rate pressure product, respiratory exchange ratio, fat oxidation rate, or carbohydrate oxidation rate.
Echocardiography and Pulse Wave Velocity
Echocardiography results are shown in Table 1. During DAPA treatment, the participants demonstrated an increased mean peak diastolic mitral annular velocity (e’) (9 ± 0.3 m/s) in comparison to placebo (8 ± 0.3 m/s; P = 0.03) predominantly due to changes in the medial e” (P = .03). The indexed LA volume was significantly reduced following DAPA compared with placebo (P = .045). The RV e” was significantly reduced in the DAPA group (9 ± 0.7 cm/s) as compared to placebo (10 ± 1 cm/s, P = .006). RV s’, when corrected for HR, was also reduced in the DAPA group (P = .042). Due to some participants having poor subcoastal acoustic windows, inferior vena cava (IVC) paired data could be obtained for only 6 participants. There were large and very large ESs associated with an increased IVC diameter in the DAPA group both inspiration (IVCi) and expiration (IVCe) respectively in comparison to placebo. ESs were not significant between treatments (IVCe: P = .06), (IVCi; P = .11). A moderate ES was also seen with cardiac output which reduced in DAPA (5 ± 0.3 L/min) compared with placebo (6 ± 0.3 L/min) caused by a significant reduction in resting HR (DAPA 70 ± 2 bpm, placebo 77 ± 3 bpm; P = .03). No significant valvular abnormalities were detected. Tricuspid regurgitation was insufficient to consistently estimate right ventricular systolic pressure.
Echocardiographic parameters following 4 weeks of dapagliflozin and 4 weeks of placebo (n = 9)
. | Unit of measure . | Dapagliflozin . | Placebo . | Cohen D (95% CI) . |
---|---|---|---|---|
LV volumes, function, and mass | ||||
End diastolic volume | mL/m2 | 56 ± 2 | 57 ± 2 | −0.2 (−1.1 to − 0.7) |
End systolic volume | mL/m2 | 23 ± 1 | 23 ± 1 | 0 (−0.8 to 0.9) |
Stroke volume (EDV-ESV) | mL/m2 | 33 ± 1 | 34 ± 1 | −0.3 (−1.2 to 0.5) |
Stroke volume (LVOTa × VTI) | mL/m2 | 38 ± 2 | 39 ± 2 | −0.1 (−0.1 to 0.7) |
Cardiac output | L/min | 5 ± 0.3 | 6 ± 0.3 | −0.6 (−1.6 to 0.3) |
Ejection fraction | % | 59 ± 0.7 | 60 ± 1 | −0.3 (−1.2 to 0.6) |
LV mass index | g/m2 | 69 ± 6 | 73 ± 7 | 0.2 (−0.6 to 1.1) |
LV longitudinal function | ||||
MAPSE (mean) | cm | 1.4 ± 0.1 | 1.4 ± 0.3 | −0.3 (−1.2 to 0.6) |
LV S’ (mean) | cm/s | 10 ± 0.7 | 11 ± 1 | −0.5 (−1.4 to 0.4) |
RV diameter and longitudinal function | ||||
RV basal diameter | cm | 3.3 ± 0.2 | 3.4 ± 0.2 | −0.2 (−0.7 to 1.1) |
RV mid diameter | cm | 2.8 ± 0.1 | 2.7 ± 0.1 | 0.2 (−0.7 to 1.1) |
TAPSE | cm | 2.3 ± 0.1 | 2.1 ± 0.2 | 0.3 (−0.6 to 1.2) |
RV S’ | cm | 11 ± 1 | 13 ± 1 | −0.7 (−1.7 to 0.2)a |
LV Diastology | ||||
E | cm/s | 76 ± 6 | 72 ± 2 | 0.2 (−0.7 to 1.1) |
LV A | cm/s | 81 ± 7 | 85 ± 8 | −0.2 (−1.1 to 0.7) |
LV DT | ms | 232 ± 2 | 207 ± 1 | 0.6 (−0.3 to 1.5) |
E/A | 1.0 ± 1.3 | 0.9 ± 0.1 | 0.3 (−0.6 to 1.2) | |
LV e” (medial) | cm/s | 8.0 ± 0.7 | 7.0 ± 0.3 | 0.9 (0.0 to 1.9)a |
LV e” (lateral) | cm/s | 10 ± 0.7 | 9 ± 0.3 | 0.5 (−0.4 to 1.4) |
LV a” (mean) | cm/s | 12 ± 1.3 | 11 ± 0.3 | 0.2 (−0.7 to 1.1 |
E/e’ | 8.5 ± 0.9 | 9.3 ± 0.9 | −0.3 (−1.2 to 0.6) | |
Atrial volumes | ||||
LA volume | mL/m2 | 19 ± 2 | 23 ± 3 | −0.7 (1.6 to 0.2)a |
RA volume | mL/m2 | 17 ± 2 | 17 ± 2 | 0 (−0.9 to 0.9) |
Inferior vena cava | ||||
IVC diameter expiration | cm | 2.0 ± 0.4 | 1.3 ± 0.1 | 1.6 (0.5 to 2.7) |
IVC diameter inspiration | cm | 1.2 ± 0.1 | 0.9 ± 0.1 | 1.2 (0.2 to 2.1) |
. | Unit of measure . | Dapagliflozin . | Placebo . | Cohen D (95% CI) . |
---|---|---|---|---|
LV volumes, function, and mass | ||||
End diastolic volume | mL/m2 | 56 ± 2 | 57 ± 2 | −0.2 (−1.1 to − 0.7) |
End systolic volume | mL/m2 | 23 ± 1 | 23 ± 1 | 0 (−0.8 to 0.9) |
Stroke volume (EDV-ESV) | mL/m2 | 33 ± 1 | 34 ± 1 | −0.3 (−1.2 to 0.5) |
Stroke volume (LVOTa × VTI) | mL/m2 | 38 ± 2 | 39 ± 2 | −0.1 (−0.1 to 0.7) |
Cardiac output | L/min | 5 ± 0.3 | 6 ± 0.3 | −0.6 (−1.6 to 0.3) |
Ejection fraction | % | 59 ± 0.7 | 60 ± 1 | −0.3 (−1.2 to 0.6) |
LV mass index | g/m2 | 69 ± 6 | 73 ± 7 | 0.2 (−0.6 to 1.1) |
LV longitudinal function | ||||
MAPSE (mean) | cm | 1.4 ± 0.1 | 1.4 ± 0.3 | −0.3 (−1.2 to 0.6) |
LV S’ (mean) | cm/s | 10 ± 0.7 | 11 ± 1 | −0.5 (−1.4 to 0.4) |
RV diameter and longitudinal function | ||||
RV basal diameter | cm | 3.3 ± 0.2 | 3.4 ± 0.2 | −0.2 (−0.7 to 1.1) |
RV mid diameter | cm | 2.8 ± 0.1 | 2.7 ± 0.1 | 0.2 (−0.7 to 1.1) |
TAPSE | cm | 2.3 ± 0.1 | 2.1 ± 0.2 | 0.3 (−0.6 to 1.2) |
RV S’ | cm | 11 ± 1 | 13 ± 1 | −0.7 (−1.7 to 0.2)a |
LV Diastology | ||||
E | cm/s | 76 ± 6 | 72 ± 2 | 0.2 (−0.7 to 1.1) |
LV A | cm/s | 81 ± 7 | 85 ± 8 | −0.2 (−1.1 to 0.7) |
LV DT | ms | 232 ± 2 | 207 ± 1 | 0.6 (−0.3 to 1.5) |
E/A | 1.0 ± 1.3 | 0.9 ± 0.1 | 0.3 (−0.6 to 1.2) | |
LV e” (medial) | cm/s | 8.0 ± 0.7 | 7.0 ± 0.3 | 0.9 (0.0 to 1.9)a |
LV e” (lateral) | cm/s | 10 ± 0.7 | 9 ± 0.3 | 0.5 (−0.4 to 1.4) |
LV a” (mean) | cm/s | 12 ± 1.3 | 11 ± 0.3 | 0.2 (−0.7 to 1.1 |
E/e’ | 8.5 ± 0.9 | 9.3 ± 0.9 | −0.3 (−1.2 to 0.6) | |
Atrial volumes | ||||
LA volume | mL/m2 | 19 ± 2 | 23 ± 3 | −0.7 (1.6 to 0.2)a |
RA volume | mL/m2 | 17 ± 2 | 17 ± 2 | 0 (−0.9 to 0.9) |
Inferior vena cava | ||||
IVC diameter expiration | cm | 2.0 ± 0.4 | 1.3 ± 0.1 | 1.6 (0.5 to 2.7) |
IVC diameter inspiration | cm | 1.2 ± 0.1 | 0.9 ± 0.1 | 1.2 (0.2 to 2.1) |
Mean ± SEM.
Abbreviations: A, late mitral inflow velocity; a”, mitral annular late diastolic velocity; DT, deceleration time; e”, mitral annular early diastolic velocity; E, early mitral inflow velocity; EDV, end-diastolic volume; ESV, end-systolic volume; IVC, inferior vena cava; LA, left atrium; LV, left ventricle; LVOT, left ventricular outflow tract; MAPSE, mitral annular plane systolic excursion; RA, right atrium; RV, right ventricle; S”, peak systolic annular velocity; TAPSE, tricuspid annular plane systolic excursion; VTI, velocity time integral.
P less than .05.
Echocardiographic parameters following 4 weeks of dapagliflozin and 4 weeks of placebo (n = 9)
. | Unit of measure . | Dapagliflozin . | Placebo . | Cohen D (95% CI) . |
---|---|---|---|---|
LV volumes, function, and mass | ||||
End diastolic volume | mL/m2 | 56 ± 2 | 57 ± 2 | −0.2 (−1.1 to − 0.7) |
End systolic volume | mL/m2 | 23 ± 1 | 23 ± 1 | 0 (−0.8 to 0.9) |
Stroke volume (EDV-ESV) | mL/m2 | 33 ± 1 | 34 ± 1 | −0.3 (−1.2 to 0.5) |
Stroke volume (LVOTa × VTI) | mL/m2 | 38 ± 2 | 39 ± 2 | −0.1 (−0.1 to 0.7) |
Cardiac output | L/min | 5 ± 0.3 | 6 ± 0.3 | −0.6 (−1.6 to 0.3) |
Ejection fraction | % | 59 ± 0.7 | 60 ± 1 | −0.3 (−1.2 to 0.6) |
LV mass index | g/m2 | 69 ± 6 | 73 ± 7 | 0.2 (−0.6 to 1.1) |
LV longitudinal function | ||||
MAPSE (mean) | cm | 1.4 ± 0.1 | 1.4 ± 0.3 | −0.3 (−1.2 to 0.6) |
LV S’ (mean) | cm/s | 10 ± 0.7 | 11 ± 1 | −0.5 (−1.4 to 0.4) |
RV diameter and longitudinal function | ||||
RV basal diameter | cm | 3.3 ± 0.2 | 3.4 ± 0.2 | −0.2 (−0.7 to 1.1) |
RV mid diameter | cm | 2.8 ± 0.1 | 2.7 ± 0.1 | 0.2 (−0.7 to 1.1) |
TAPSE | cm | 2.3 ± 0.1 | 2.1 ± 0.2 | 0.3 (−0.6 to 1.2) |
RV S’ | cm | 11 ± 1 | 13 ± 1 | −0.7 (−1.7 to 0.2)a |
LV Diastology | ||||
E | cm/s | 76 ± 6 | 72 ± 2 | 0.2 (−0.7 to 1.1) |
LV A | cm/s | 81 ± 7 | 85 ± 8 | −0.2 (−1.1 to 0.7) |
LV DT | ms | 232 ± 2 | 207 ± 1 | 0.6 (−0.3 to 1.5) |
E/A | 1.0 ± 1.3 | 0.9 ± 0.1 | 0.3 (−0.6 to 1.2) | |
LV e” (medial) | cm/s | 8.0 ± 0.7 | 7.0 ± 0.3 | 0.9 (0.0 to 1.9)a |
LV e” (lateral) | cm/s | 10 ± 0.7 | 9 ± 0.3 | 0.5 (−0.4 to 1.4) |
LV a” (mean) | cm/s | 12 ± 1.3 | 11 ± 0.3 | 0.2 (−0.7 to 1.1 |
E/e’ | 8.5 ± 0.9 | 9.3 ± 0.9 | −0.3 (−1.2 to 0.6) | |
Atrial volumes | ||||
LA volume | mL/m2 | 19 ± 2 | 23 ± 3 | −0.7 (1.6 to 0.2)a |
RA volume | mL/m2 | 17 ± 2 | 17 ± 2 | 0 (−0.9 to 0.9) |
Inferior vena cava | ||||
IVC diameter expiration | cm | 2.0 ± 0.4 | 1.3 ± 0.1 | 1.6 (0.5 to 2.7) |
IVC diameter inspiration | cm | 1.2 ± 0.1 | 0.9 ± 0.1 | 1.2 (0.2 to 2.1) |
. | Unit of measure . | Dapagliflozin . | Placebo . | Cohen D (95% CI) . |
---|---|---|---|---|
LV volumes, function, and mass | ||||
End diastolic volume | mL/m2 | 56 ± 2 | 57 ± 2 | −0.2 (−1.1 to − 0.7) |
End systolic volume | mL/m2 | 23 ± 1 | 23 ± 1 | 0 (−0.8 to 0.9) |
Stroke volume (EDV-ESV) | mL/m2 | 33 ± 1 | 34 ± 1 | −0.3 (−1.2 to 0.5) |
Stroke volume (LVOTa × VTI) | mL/m2 | 38 ± 2 | 39 ± 2 | −0.1 (−0.1 to 0.7) |
Cardiac output | L/min | 5 ± 0.3 | 6 ± 0.3 | −0.6 (−1.6 to 0.3) |
Ejection fraction | % | 59 ± 0.7 | 60 ± 1 | −0.3 (−1.2 to 0.6) |
LV mass index | g/m2 | 69 ± 6 | 73 ± 7 | 0.2 (−0.6 to 1.1) |
LV longitudinal function | ||||
MAPSE (mean) | cm | 1.4 ± 0.1 | 1.4 ± 0.3 | −0.3 (−1.2 to 0.6) |
LV S’ (mean) | cm/s | 10 ± 0.7 | 11 ± 1 | −0.5 (−1.4 to 0.4) |
RV diameter and longitudinal function | ||||
RV basal diameter | cm | 3.3 ± 0.2 | 3.4 ± 0.2 | −0.2 (−0.7 to 1.1) |
RV mid diameter | cm | 2.8 ± 0.1 | 2.7 ± 0.1 | 0.2 (−0.7 to 1.1) |
TAPSE | cm | 2.3 ± 0.1 | 2.1 ± 0.2 | 0.3 (−0.6 to 1.2) |
RV S’ | cm | 11 ± 1 | 13 ± 1 | −0.7 (−1.7 to 0.2)a |
LV Diastology | ||||
E | cm/s | 76 ± 6 | 72 ± 2 | 0.2 (−0.7 to 1.1) |
LV A | cm/s | 81 ± 7 | 85 ± 8 | −0.2 (−1.1 to 0.7) |
LV DT | ms | 232 ± 2 | 207 ± 1 | 0.6 (−0.3 to 1.5) |
E/A | 1.0 ± 1.3 | 0.9 ± 0.1 | 0.3 (−0.6 to 1.2) | |
LV e” (medial) | cm/s | 8.0 ± 0.7 | 7.0 ± 0.3 | 0.9 (0.0 to 1.9)a |
LV e” (lateral) | cm/s | 10 ± 0.7 | 9 ± 0.3 | 0.5 (−0.4 to 1.4) |
LV a” (mean) | cm/s | 12 ± 1.3 | 11 ± 0.3 | 0.2 (−0.7 to 1.1 |
E/e’ | 8.5 ± 0.9 | 9.3 ± 0.9 | −0.3 (−1.2 to 0.6) | |
Atrial volumes | ||||
LA volume | mL/m2 | 19 ± 2 | 23 ± 3 | −0.7 (1.6 to 0.2)a |
RA volume | mL/m2 | 17 ± 2 | 17 ± 2 | 0 (−0.9 to 0.9) |
Inferior vena cava | ||||
IVC diameter expiration | cm | 2.0 ± 0.4 | 1.3 ± 0.1 | 1.6 (0.5 to 2.7) |
IVC diameter inspiration | cm | 1.2 ± 0.1 | 0.9 ± 0.1 | 1.2 (0.2 to 2.1) |
Mean ± SEM.
Abbreviations: A, late mitral inflow velocity; a”, mitral annular late diastolic velocity; DT, deceleration time; e”, mitral annular early diastolic velocity; E, early mitral inflow velocity; EDV, end-diastolic volume; ESV, end-systolic volume; IVC, inferior vena cava; LA, left atrium; LV, left ventricle; LVOT, left ventricular outflow tract; MAPSE, mitral annular plane systolic excursion; RA, right atrium; RV, right ventricle; S”, peak systolic annular velocity; TAPSE, tricuspid annular plane systolic excursion; VTI, velocity time integral.
P less than .05.
Arterial stiffness was not different between treatments (DAPA 9.35 ± 0.60 m/s; placebo 9.07 ± 0.72 m/s).
Discussion
The aim of this study was to ascertain if an exertional stimulus would further elucidate mechanisms of the beneficial cardiovascular effects of SGLT2 inhibition. During exercise there was a significant increase in BOHB and GH and a significant decrease in lactate and glucose following 4 weeks of DAPA in comparison to placebo. We were also able to demonstrate differences in the participants’ resting echocardiography, principally a reduction in LA volume and an increase in e” following DAPA. Importantly, the 4-week treatment period and the crossover design of our study demonstrate that these hormonal, metabolic, and hemodynamic changes can be considered rapid in onset as well as reversible.
To our knowledge, no study has investigated the effect of SGLT2 inhibitors on GH. Current mechanistic thinking of SGLT2 inhibitors may therefore be missing the key role that GH plays in regulating glucose, lipid, and protein metabolism homeostasis. DAPA has been shown to reduce hyperinsulinemia and restore pulsatile GH secretion in obese mice resulting in decreased fat mass, improved insulin sensitivity, improved glucose tolerance along with preserved lean mass and delayed type 2 diabetes progression, which is thought to be due to a reduction in the secretion pressure on β cells (22). The mechanisms underlying GH secretion in obesity are not well understood but it is known that hyperinsulinemia inhibits GH secretion from the pituitary gland, regardless of systemic insulin resistance (23), although this is not thought to be centrally influenced by SGLT2 inhibitors (22). While our study showed a trend toward reduced insulin following DAPA, due to the wide SD this was not significant. Similarly, although it is well documented that SGLT2 inhibitors raise plasma glucagon levels in most human studies (24-26), we did not observe changes in circulating levels of glucagon. This may be due to the small number of participants in our study. The increase in human GH detected in the present study suggests it may have an important role in the effect of SGLT2 inhibitors to shift substrate utilization from glucose to lipid demonstrated by the increase in BOHB and decrease in glucose and lactate concentrations. We reported previously that urinary glucose excretion was significantly increased by DAPA in these individuals, lowering glucose concentration (21). We also reported that glucose uptake into peripheral tissues was reduced with DAPA, and this is a likely mechanism for the drive in ketogenesis. Although we were unable to demonstrate a difference in carbohydrate and lipid oxidation between treatments in the present study, in a placebo-controlled study of DAPA in 24 people with type 2 diabetes, Op Den Kamp et al (27) showed a shift from carbohydrate to lipid oxidation both during fasting and over 24 hours, measured using whole-room calorimetry.
Sympathetic hyperactivity is a characteristic of type 2 diabetes (28). SGLT2 inhibitors have been shown to lower blood pressure (29) without increasing HR, which suggests suppression of sympathetic nervous system (SNS) activity. In a hypertensive mouse model DAPA has been shown to reduce sympathetic innervation, which may underlie the observed reduction in blood pressure and improvement in endothelial function with DAPA in these mice (30). However, in response to our exercise protocol, plasma concentrations of adrenaline and noradrenaline were not different with DAPA, providing no evidence for suppression of SNS activity. We cannot, however, rule out differences in SNS activity at the tissue level that would not be detected in the study.
SGLT2 inhibitors are known to increase levels of BOHB, which has been implicated as an alternative but adaptive fuel source in heart failure both in diabetic and nondiabetic patients (15). BOHB has also been shown to decrease the production of reactive oxygen species and activate autophagy as well as potentially playing a role in transcriptional or posttranscriptional regulation of gene expression such as the upregulation of genes involved in gluconeogenesis (31, 32). We have previously shown that meal-derived fatty acids contribute to the increase in ketones during treatment with DAPA, which we considered was due to increased ketogenesis within the liver (21). Ketogenesis is sensitive to multiple hormonal stimuli including insulin, which reduces lipolysis and ketogenesis in the liver (33, 34), while catecholamines and GH stimulate lipolysis and subsequently ketogenesis (35). The myocardium is the largest consumer of ketone bodies per unit mass (36). In HF, ketone body concentrations are increased in the circulation of patients with acute and chronic HF, both with reduced and preserved ejection fraction (37)and also in animal models (38, 39), which is thought to be an autonomous but adaptive response to cardiac stress (40-42) by providing an additional, oxygen-efficient fuel source.
We also found a novel reduction in lactate following DAPA. A study of adipose tissue biopsies from 49 patients who underwent open heart surgery, some treated with DAPA and some without, showed that DAPA reduced the released lactate and acidosis in epicardial fat (P < .05) without changes in lipid storage–involved genes (43). This led the authors to conclude that DAPA improved glucose metabolism without lipogenesis-involved gene regulation or lactate production. A murine study demonstrated that empagliflozin, in isolated diabetic hearts, decreased lactate generation from labeled glucose and increased α-ketoglutarate synthesis from labeled palmitate (44). The decreased lactate generation seemed to be mediated through inhibition of the cardiac sodium/hydrogen exchanger (NHE-1), which is thought to be a novel target to prevent cardiac disease (45).
Our study demonstrated a rise in the speed of early LV relaxation (e’) during diastole reflecting increased ventricular compliance or reduced LV stiffness. The increases in e” were not due to physical training or the transient decrease in e” seen acutely after intense high-volume exercise (46). Furthermore e” is a relatively, but not absolutely, load- (preload and afterload) independent measure of LV relaxation (47). Beneficial alterations in diastolic function have been demonstrated in several echocardiographic studies with SGLT2 inhibitors (48). Overall this would indicate that the heart is showing intrinsic improvements in diastolic function due to increased compliance and reduced stiffness. We were also able to demonstrate a reduction in LA volume that again would be commensurate with lower LA pressure due to improved LV compliance, which has been demonstrated in other studies (49). The significant reduction in RV s’ in the dapagliflozin arm was an unexpected finding. While evidence is accumulating for the beneficial aspects of SGLT2 inhibitors in pulmonary hypertension secondary to left heart disease, studies in rodent models have also shown a potential effect on pulmonary arterial hypertension with hemodynamic, pulmonary vascular and myocardial architectural alterations (50). This may be associated with a reduction in pulmonary hypertension; however, it is difficult to interpret a reduction in RV S’ in the context of this as elevated pulmonary arterial systolic pressure is usually accompanied by a reduction in RV longitudinal function (51, 52).
The strength of this study is the crossover design, which demonstrates that the cardiac and metabolic changes from SGLT2 inhibition are reversible, at least following a short course of treatment. A further strength is the novel exercise component, which allows the effect of SGLT2 inhibition to be assessed with a stressed cardiovascular system. The weakness of this study is the small patient numbers due to having to stop recruitment with the onset of the COVID-19 pandemic. The findings are therefore of interest but further confirmatory studies are required.
Conclusion
Our study suggests that during exercise GH may be more important than catecholamines in driving a shift in fuel consumption from glucose to fat oxidation leading to reduced lactate and increased ketogenesis with DAPA treatment. This appears to be associated with improvements in diastolic function, which is likely to be a consequence of the metabolic adaptations secondary to SGLT2 inhibition. The cardiac and metabolic changes due to SGLT2 inhibition are rapid in onset and reversible.
Acknowledgments
We thank Rachael Gribbin for her laboratory assistance; Eleanor Drury and Abby Barton, who assisted on breath analysis; and Dr Benita Middleton for the metabolomics analysis.
Financial Support
Astra Zeneca Ltd funded the study and provided the investigational medicinal product.
Author Contributions
R.H., D.L.R.J., A.M.U., B.F., and M.D. designed the study; M.S. and F.S.M. carried out the metabolic studies; I.P. conducted TTE measurements; F.S.M. carried out sample analysis and N.J. assisted; A.M.U., B.F., R.H., I.P., and F.S.M, drafted the manuscript. All authors reviewed and edited the manuscript.
Disclosures
M.D. has acted as consultant, advisory board member, and speaker for Novo Nordisk, Sanofi-Aventis, Lilly, Merck Sharp & Dohme, Boehringer Ingelheim, AstraZeneca, and Janssen; an advisory board member for Servier and Gilead Sciences Ltd; as a speaker for NAPP, Mitsubishi Tanabe Pharma Corporation, and Takeda Pharmaceuticals International Inc; and received grants in support of research trials from Novo Nordisk, Sanofi-Aventis, Lilly, Boehringer Ingelheim, AstraZeneca, and Janssen. D.L.R.J. and R.H. have received research funding or advisory board or lecture fee honoraria from Astra Zeneca. The remaining authors have nothing to disclose.
Data Availability
All data sets generated and analyzed during the present study are not publicly available but are available from the corresponding author on reasonable request.
Clinical Trial Information
ClinicalTrials.gov number NCT04219124 (registered January 6, 2020).
References
Abbreviations
- BOHB
β-hydroxybutyrate
- DAPA
dapagliflozin
- EDV
end-diastolic volume
- ELISA
enzyme-linked immunosorbent assay
- ES
effect size
- ESV
end-systolic volume
- GH
growth hormone
- HR
heart rate
- IVC
inferior vena cava
- LA
left atrium
- LV
left ventricle
- LVOT
left ventricular outflow tract
- NEFA
nonesterified fatty acid concentrations
- PWV
pulse wave velocity
- RA
right atrium
- RV
right ventricle
- SNS
sympathetic nervous system
- TTE
transthoracic echocardiogram
- VO2max
maximal oxygen consumption