-
PDF
- Split View
-
Views
-
Cite
Cite
Klas Ekström, Mari-Anne Pulkkinen, Christine Carlsson-Skwirut, Anna-Lena Brorsson, Zhulin Ma, Jan Frystyk, Peter Bang, Tissue IGF-I Measured by Microdialysis Reflects Body Glucose Utilization After rhIGF-I Injection in Type 1 Diabetes, The Journal of Clinical Endocrinology & Metabolism, Volume 100, Issue 11, 1 November 2015, Pages 4299–4306, https://doi.org/10.1210/jc.2015-2070
- Share Icon Share
Type 1 diabetes is associated with portal insulin deficiency and disturbances in the GH-IGF axis including low circulating IGF-I and GH hypersecretion. Whether peripheral hyperinsulinemia and GH hypersecretion, which are relevant to the development of vascular complications, result in elevated tissue IGF-I remains unknown.
The purpose of this study was to determine the relationship between whole-body glucose uptake and tissue IGF-I measured by microdialysis.
This was a single-blind placebo-controlled crossover study.
The setting was a tertiary pediatric endocrine referral center.
The participants were seven young male adults with type 1 diabetes.
After an overnight fast, a 6-h lasting euglycemic clamp was performed (constant insulin infusion at 0.5 mU/kg × minute and variable glucose infusion rate [GIR]) and a subcutaneous injection of recombinant human (rh) IGF-I (120 μg/kg) or saline was given after 2 hours. In parallel, tissue IGF-I levels were determined by microdialysis (md-IGF-I).
md-IGF-I levels in muscle and subcutaneous fat, and GIR were determined.
md-IGF-I levels were detectable but unchanged after saline. After rhIGF-I, muscle and subcutaneous fat md-IGF-I increased during the second and third hour and then reached a plateau up to 10-fold higher than baseline (P < .001). GIR was unchanged after saline, whereas it increased 2.5-fold concomitantly with the increase in md-IGF-I (P < .0001). In contrast, serum IGF-I was increased already at 30 minutes after rhIGF-I and reached a plateau 2-fold above baseline (P < .0001).
We demonstrate that md-IGF-I measurements are valid and physiologically relevant by reflecting rhIGF-I–induced glucose uptake. Future studies should be conducted to elucidate the role of local tissue IGF-I in diabetic vascular complications.
Insulin resistance and impaired metabolic control are common problems in adolescents with type 1 diabetes mellitus. Endocrine disturbances in the GH-IGF axis are considered to be important in this regard (1, 2). However, little is known about the paracrine/autocrine changes in the GH-IGF axis in type 1 diabetes and studies on tissue levels of IGF-I are lacking.
The liver is the main site for the production of circulating IGF-I, and animal studies indicate that there is only a minor contribution from extrahepatic tissues (here called “tissues”) (3). IGF-I expression is regulated by GH, and the GH receptor function and signaling are dependent on insulin (4, 5). In type 1 diabetes, subcutaneous injections of insulin result in portal insulin deficiency and low circulating IGF-I levels, particularly in adolescents (6). The use of continuous subcutaneous insulin infusion (CSII) or long-acting insulin analogs only partly restores serum IGF-I levels and GH hypersecretion persists (7, 8). Only experimental intraportal or intraperitoneal insulin delivery has the potential to completely restore IGF-I levels (9–11) and to eliminate GH hypersecretion (9).
Low circulating IGF-I levels increase GH secretion by an attenuated negative feedback (2, 3, 12), and an elevated GH concentration is thought to be the main factor causing insulin resistance in type 1 diabetes. Direct blocking of GH production (13, 14) improves insulin sensitivity as does administration of recombinant human (rh) IGF-I per se (15). In overnight studies, rhIGF-I also reduced GH hypersecretion and insulin requirements in adolescents and young adults with type 1 diabetes (16, 17). The beneficial effects of adjunctive rhIGF-I therapy on metabolic control and insulin requirements have been demonstrated in 4- to 24-week studies in adolescents with type 1 diabetes (18, 19). The rhIGF-I dose required for these effects (20–80 μg/kg once daily) are markedly lower than the approved rhIGF-I dose (120 μg/kg twice daily) for treatment of short stature in severe primary IGF-I deficiency (SPIGFD) (20).
During adolescence, increased insulin doses are required to maintain good metabolic control, which leads to further hyperinsulinemia in the tissues. The effects of GH hypersecretion and tissue hyperinsulinemia and their relation to local paracrine/autocrine tissue production of IGF-I have not been studied in type 1 diabetes. However, the normal linear growth in children with type 1 diabetes suggests that tissue IGF-I levels are normal or high despite the low circulating levels of IGF-I (21).
Compensatory up-regulation of tissue IGF-I may affect the progression of diabetic vascular complications (22). Interestingly, early treatment with rhIGF-I is currently being assessed in a phase 2 trial (ClinicalTrials.gov identifier: NCT01096784) to prevent retinopathy of the premature associated with circulating IGF-I deficiency, hyperglycemia, and insulin resistance similar to the diabetic conditions. In retinopathy of the premature, there is evidence that after the first vascular insult associated with IGF-I deficiency, a second phase of proliferative changes is driven by increased IGF-I in recovering premature infants (23).
In humans, intravenously injected rhIGF-I induces glucose uptake and oxidation in skeletal muscle, although with a 13.5-fold lower potency than insulin (24, 25). On the other hand, rhIGF-I and insulin are equipotent in stimulating glucose uptake in human muscle strips ex vivo (26). IGF binding proteins (IGFBPs) modulate IGF-I access to the peripheral tissues, as well as local tissue IGF-I bioavailability, and are thought to account for this potency difference. The IGF-I receptor (IGF-1R), as well as hybrids of the insulin receptor (IR) and IGF-1R are highly abundant in skeletal muscle, whereas the expression of IGF-1Rs in liver and fat tissue is low (27, 28). In mice, double knockout of the IR and IGF-1R is needed to generate a diabetic phenotype, again pointing to muscle as the major site of IGF-I actions on glucose metabolism (29). There is one report suggesting direct hepatic IGF-I effects in a human rhIGF-I clamp study (30). However, that study did not address whether the experimental conditions allowed IGF-I cross-reactivity on the IR or whether hepatic IGF-1R were present.
Microdialysis as a technique to measure tissue IGF-I (md-IGF-I) levels in human muscle was first reported by Desvigne et al (31) and later validated in our reports of increased md-IGF-I levels in exercising muscle (32, 33). The microdialysis technique has a potential for the assessment of paracrine/autocrine IGF-I abnormalities in type 1 diabetes. However, it remains to be demonstrated that md-IGF-I levels in the tissues are linked to local actions of IGF-I on glucose metabolism.
In this study, we evaluated whether changes in tissue md-IGF-I and in circulating IGF-I induced by a subcutaneous injection of rhIGF-I or saline were associated with changes in glucose utilization in young adults with type 1 diabetes during a euglycemic clamp procedure. The impact of rhIGF-I on the glucoregulatory hormones GH, cortisol, and IGFBPs was also assessed.
Subjects and Methods
Subjects
Eight young male adults with type 1 diabetes were enrolled in the study. One subject discontinued the study at his own request after the first half of the study (after receiving saline), and his data were not included in the analysis. Inclusion criteria for the study were type 1 diabetes of ≥2 years' duration, age between 16 and 25 years, full maturation (Tanner stage 5), treatment with either CSII or multiple dose injection therapy. Exclusion criteria were untreated hypothyroidism or celiac disease with poor control (increase in transglutaminase titers). The baseline characteristics of the subjects were as follows: mean age 19.2 years (range, 18.3–20.9 years), mean diabetes duration, 9.0 years (range, 6.4–11.8 years), mean body mass index SD score, 0.41 (range, −0.49 to 2.34), and mean hemoglobin A1c (HbA1c), 64 mmol/mol (range, 50–90 mmol/mol).
The Central Ethical Review Board, Stockholm, and the Medical Products Agency, Sweden, approved the study protocol. All subjects gave their written informed consent.
Study protocol
This was a randomized, single-blind, placebo-controlled crossover study of the effects of subcutaneous rhIGF-I administration on the glucose infusion rate (GIR), a measure of whole-body glucose utilization and interstitial muscle and adipose tissue IGF-I concentrations under euglycemic clamp conditions. Each subject was studied twice and randomized to receive a subcutaneous injection of rhIGF-I (120 μg/kg [10 mg/mL], Increlex; Ipsen) or saline (0.1 ml, 0.9% NaCl), as outlined in Figure 1. The subcutaneous injection was given in the abdominal fat tissue, approximately 10 cm lateral to the umbilicus at the contralateral site of the abdominal microdialysis catheter. Strenuous exercise was avoided, and glucose control was optimized by CSII for at least 48 hours before each study day. The 2 study periods were separated by a median interval of 12 days (range, 7–43 days). Subjects were admitted to the hospital at 8:00 am after an overnight fast (from 10 pm). After the application of a local anesthetic cream (EMLA; AstraZeneca), 2 indwelling cannulae were inserted, 1 into the distal forearm vein for blood sampling, and 1 into the antecubital fossa vein for continuous insulin and glucose infusion. Three microdialysis catheters were inserted as outlined below. CSII was stopped, and a normoinsulinemic euglycemic clamp was started and monitored by frequent bedside glucose measurements (detailed below). A 1-hour run-in period was allowed to reach euglycemia (target plasma glucose, 5.0 mmol/L) and calibrate the microdialysis catheters. Collection of the microdialysate was then commenced, using 1-hour fractions, whereas blood was sampled every 30 minutes. Microdialysate and serum samples were stored at −40°C until the biochemical analysis. After completing the study day, subjects started their usual insulin therapy and received a meal. They were observed for at least 1 hour poststudy and instructed to perform frequent self-monitoring of blood glucose (see Safety issues below).
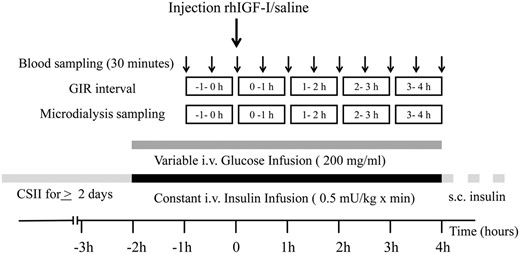
Microdialysis
Tissue IGF-I concentrations were determined by microdialysis in the left (LMVL) and right musculus vastus lateralis (RMVL) and in abdominal subcutaneous fat (ASF), as described by Berg et al (32, 33). Probes, perfusion pumps, and perfusion fluid were all purchased from CMA Microdialysis AB. In brief, after induction of local anesthesia (10 mg/mL mepivacaine hydrochloride [Carbocain]; AstraZeneca), 3 microdialysis catheters (CMA63 MD 40/30, 30-mm membrane length, and cutoff of 20 kDa) were inserted into the LMVL and RMVL (directed cranially in the sagittal plane at an angle of 45°) and into the ASF. The distal end of the outlet tubing was cut off and inserted into a polypropylene tube to collect the microdialysate for determinations of IGF-I (7.5 kDa). The microdialysis catheters were perfused (2 μl/min) with a nonbuffered water solution (CMA perfusion fluid T1) with an addition of 0.05% human serum albumin (50 g/l albumin; Baxter). After a 1-hour run-in period, microdialysate collections in 1-hour fractions of 120 μl were started (Figure 1). We have previously demonstrated that only unbound IGF-I crosses the membrane of the microdialysis catheter and that neither IGFBPs nor their fragments are detectable in the microdialysate (32). Furthermore, we have demonstrated that IGF-I recovery in the microdialysate is 16 ± 6% in vitro and 11 ± 1% in vivo under resting conditions (33).
Normoinsulinemic euglycemic clamp
After overnight fasting, a normoinsulinemic euglycemic clamp, modified from Ekström et al (34), was performed. A primed infusion of insulin (0.5 mU/kg × minute, 1 IU/mL, Actrapid; Novo Nordisk A/S) was given. The rate of insulin infusion (0.72 IU/kg × day) was chosen to slightly exceed the normal insulin needs in postpubertal individuals with well-controlled type 1 diabetes, thus allowing euglycemia (5 mmol/L) to be maintained by a variable 200 mg/mL glucose infusion. The forearm was kept in a heated box to arterialize the venous blood. Plasma glucose was checked every 5 to 10 minutes. The GIR was calculated during 1-hour intervals from the amount of glucose infused per minute divided by body weight (milligrams per kilogram × minute).
Biochemical analyses
Plasma glucose was determined by the glucose dehydrogenase method (Hemocue AB). The 30-minute mean plasma glucose values presented here were calculated as the average of all measurements from −15 minutes to +15 minutes of each 30-minute period. Serum IGF-I levels were determined every 30 minutes by a validated in-house time-resolved immunofluorometric assay (TR-IFMA), as described earlier (35) with some modifications. In brief, serum samples were diluted 1:200 with acetic acid, incubated for 2 hours at 5°C, and subsequently neutralized with equal volumes of Tris base containing an excess of IGF-II in microtiter plate wells precoated with monoclonal capturing anti-IGF-I antibody (MAB 41; generously provided by Novo Nordisk). After overnight incubation at 5°C and washing, a biotinylated polyclonal detection anti-IGF-I antibody (I-8773; Sigma-Aldrich) was added and incubated for 2 hours at room temperature, whereupon all wells were washed. Europium-labeled streptavidin was then added and incubated for 1 hour at room temperature. After 6 washes, enhancement solution (PerkinElmer Life Sciences) was added to all wells to dissociate protein-bound Eu3+. Time-resolved fluorescence was measured after 10 minutes using a PerkinElmer Victor multilabel plate reader. The intraassay and interassay coefficients of variation (CVs) averaged 5% and 10%, respectively. The md-IGF-I levels were determined by the same TR-IFMA, but the microdialysate was only diluted 1:1 with a mixture of equal volumes of acetic acid and Tris buffer containing IGF-II. Standards of the TR-IFMA were rhIGF-I (World Health Organization International Standard NIBSC 02/254) in a range from 0.156 to 5 μg/L for serum samples and from 0.039 to 1.25 μg/L for microdialysis samples. If md-IGF-I was undetectable, the value was set to 0.
Serum levels of insulin and GH were analyzed every 30 minutes using commercial TR-IFMAs (PerkinElmer Life Science). The intraassay and interassay CVs were 2.1% to 3.7% and 3.3% to 3.8% for insulin and 2.1% to 5.0% and 3.7% to 6.3% for GH. Serum levels of IGFBP-1 were analyzed hourly using a commercial ELISA (Mediagnost). The intraassay and interassay CVs were less than 6.8% and 7.4%, respectively. Hourly serum levels of cortisol were analyzed by a commercial RIA (Siemens Healthcare Diagnostics AB). The intraassay and interassay CVs were less than 5.1% and 6.4%, respectively. HbA1c was analyzed by ion-exchange HPLC on a Variant II Turbo system (Bio-Rad Laboratories). The intraassay and interassay CVs were 1.9% and 2.4%, respectively, at 5.6% (38 mmol/mol); the normal value was 4.6% to 6.0% (Diabetes Control and Complications Trial [DCCT] standard) or 27–42 mmol/mol, <50 years (International Federation of Clinical Chemistry and Laboratory Medicine [IFCC] standard).
Safety issues
The most common side effect of rhIGF-I in short children with SPIGFD is hypoglycemia (36). However, no clinical signs of hypoglycemia were recorded during the study; the lowest individual plasma glucose level was 3.6 mmol/L at 30 minutes before the rhIGF-I injection. Evening and night self-monitoring of blood glucose did not detect any hypoglycemia after rhIGF-I injection, and the subjects reported back the following morning. No other adverse events were noted except slight bleeding and/or pain at catheter/cannula injection sites.
Statistical analysis
Power calculations based on previous md-IGF-I studies (32, 33) demonstrated sufficient power with 6 patients (paired t test: power, 0.88; difference in means, 0.04; SD, 0.025; group size, 6; α, .05). The statistical data analysis was performed using Sigma Plot 11.0 (SYSTAT Software, Inc). Data are given as mean values and SEM when normally distributed and otherwise as the median and 25th to 75th percentiles. Follow-up data were analyzed by two-way repeated-measures ANOVA (one-factor repetition) with all pairwise multiple comparison procedures (Holm-Sidak method). P values of <.05 were considered significant.
Results
Tissue IGF-I in muscle and subcutaneous fat (md-IGF-I)
Median levels of md-IGF-I in LMVL, RMVL, and ASF increased significantly and in parallel in all compartments during the second and third hour after rhIGF-I and then remained elevated (Figure 2, A–C): in LMVL, from 0.058 μg/L (0.003–0.111 μg/L) to 0.343 μg/L (0.256–0.551 μg/L); in RMVL, from 0.037 μg/L (0.026–0.068) μg/L to 0.350 μg/L (0.335–0.559 μg/L); and in ASF from 0.082 μg/L (0.025–0.126 μg/L) to 0.655 μg/L (0.455–1.004 μg/L) (all P ≤ .001). In contrast, there was no change in md-IGF-I after saline. In all 3 compartments, the median levels of md-IGF-I were significantly higher after rhIGF-I vs saline, starting from the second hour after injection. md-IGF-I levels in fat and muscle did not differ over time and were undetectable in 17.1% of the measurements in muscle and in 11.4% in subcutaneous fat, but only after saline.
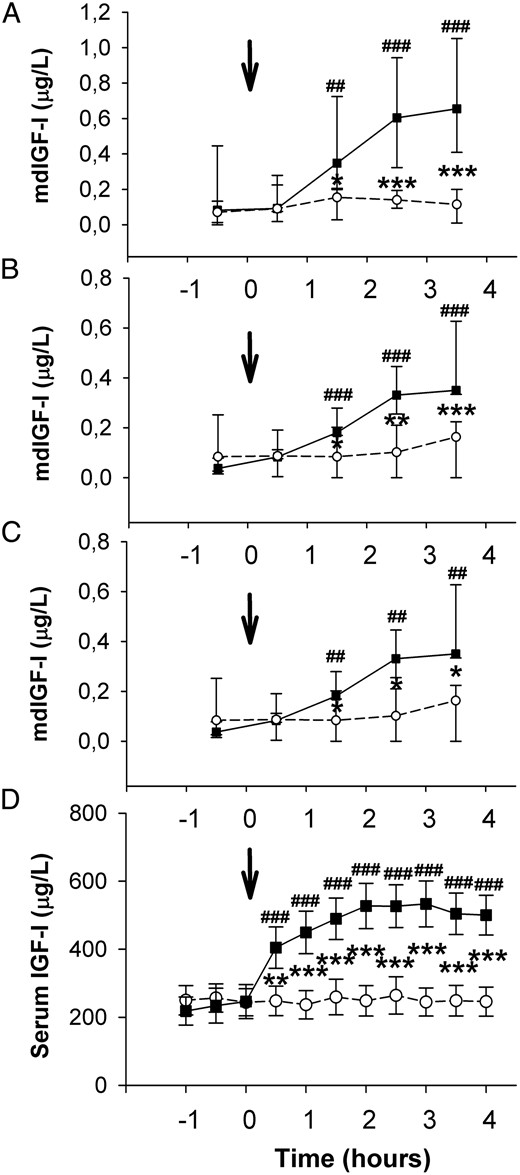
A–D, md-IGF-I in ASF (A), RMVL (B), and LMVL (C) collected in 1-hour fractions.
D, Serum IGF-I concentrations were determined every 30 minutes. The arrows indicate the time for subcutaneous rhIGF-I/saline injection. rhIGF-I, solid line with black boxes; saline, dashed line with open circles. In A–C, data are given as the median and range (25th to 75th percentile) and in D as mean values and SEM. Differences between groups: *, P < .05; **, P < .01; ***, P < .001. Differences between time points: ##, P < .01, ###, P < .001.
Circulating IGF-I
Serum IGF-I increased significantly during the first 30 minutes after rhIGF-I injection from 246 ± 50 to 405 ± 61 μg/L (P < .001), reached a maximum level 3 hours postinjection (533 ± 67 μg/L), and remained increased throughout the study. There was no change in serum IGF-I after saline (Figure 2D).
GIR
Normoinsulinemic euglycemic clamps were associated with stable insulin and glucose levels from −1 to 0 hours before rhIGF-I/saline injection until the end of the study (Figure 3, A and B). The baseline (−1 to 0 hours) mean GIR was 2.22 ± 0.54 mg/kg × minute and did not change during the first hour after the rhIGF-I injection. The mean GIR then increased significantly during the second hour (1–2 hours) and third hour (2–3 hours) and reached a maximum of 5.11 ± 0.92 mg/kg × minute (P < .001) during the fourth hour (3–4 hours) as shown in Figure 3C. There was no change in the mean GIR after saline injection. The mean GIR was significantly elevated after rhIGF-I, compared with that for saline, at the second, third, and fourth hours.
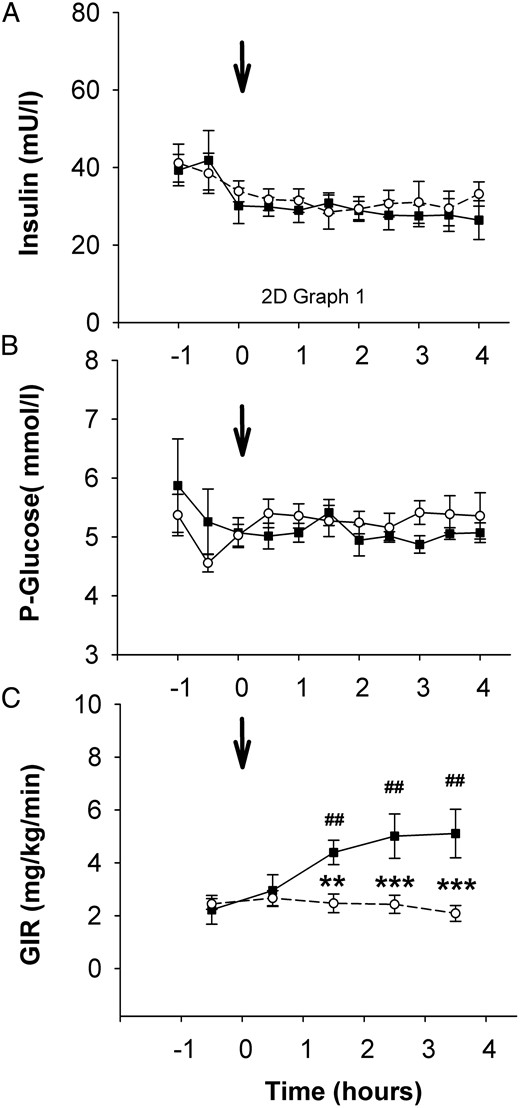
A–C, Serum insulin (A) and plasma glucose (B) concentrations and mean GIR calculated in 1-hour fractions (C).
The arrow indicates the time for subcutaneous rhIGF-I/saline injection. rhIGF-I, solid line with black boxes; saline, dashed line with open circles. Data are given as mean values and SEM. Differences between groups: **, P < .01. Differences between time points: ##, P < .01.
Glucoregulatory hormones
The mean GH levels did not change over time and did not differ between the rhIGF-I and saline groups (Figure 4A). IGFBP-1 decreased steadily in response to the insulin infusion but was not affected by the injection of rhIGF-I compared with saline (Figure 4B). The initially higher morning cortisol levels decreased steadily during both study days (Figure 4C) without any difference between saline and rhIGF-I.
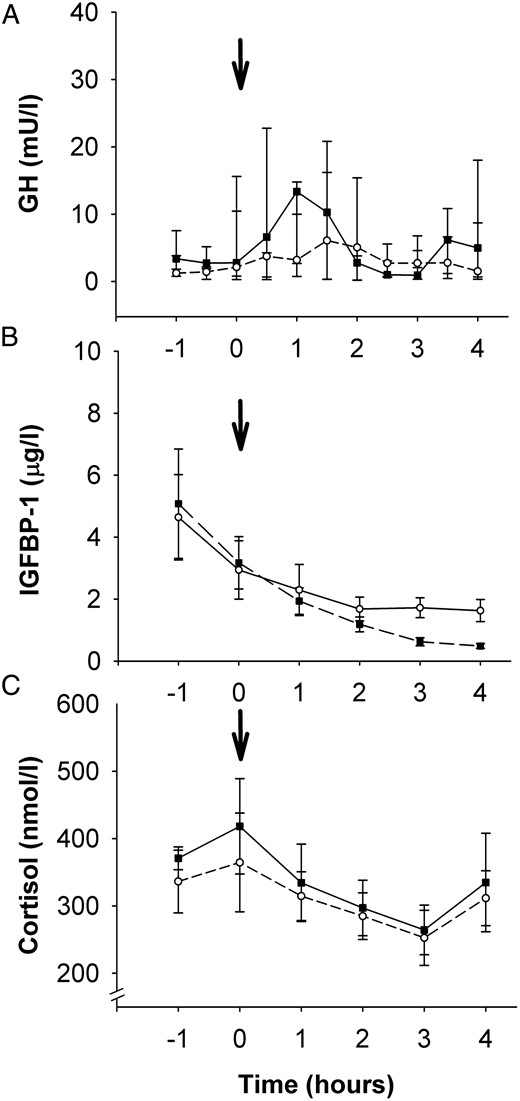
A–C, Serum GH (A), IGFBP-1 (B), and cortisol (C) concentrations.
The arrow indicates the time for the subcutaneous rhIGF-I/saline injection. rhIGF-I, solid line with black boxes; saline, dashed line with open circles. Data are given as mean values and SEM.
Discussion
We show for the first time that muscle and subcutaneous fat IGF-I levels determined by microdialysis directly reflect the action of IGF-I on glucose metabolism and thus is a valid method for detection of tissue IGF-I levels in type 1 diabetic subjects. We also demonstrate that subcutaneous injection of rhIGF-I induces a sustained increase in glucose utilization with a simultaneous increase in tissue md-IGF-I levels for at least 4 hours.
Circulating IGF-I was increased already after 30 minutes demonstrating that time is required before subcutaneously injected IGF-I is taken up by the circulation. In contrast, after an intravenous injection, IGF-I is momentarily increased in the circulation, whereas it takes 30 minutes before any glucose-lowering effect is detectable (24), indicating that injected IGF-I is retained in the circulation by the IGFBPs. This is in line with the present observation that the increase in md-IGF-I levels was detected in muscle and subcutaneous fat during the second hour after the subcutaneous injection of rhIGF-I. It is noteworthy that the GIR increased with the same delay, indicating that glucose uptake and utilization correlate with local tissue rather than circulating changes in IGF-I levels.
The present data support the fact that rhIGF-I has profound effects on glucose uptake and the most likely target is muscle. Although this was not directly addressed by tracer studies, the distribution of IGF-1R and IGF-1R/IR hybrid receptors suggests that the actions of IGF-I are mainly in skeletal muscles and less in liver or adipose tissue (26–30). Circulating IGF-I also has important indirect effects on glucose metabolism via its negative feedback on the pituitary secretion of GH (1, 2, 13) and by affecting other glucoregulatory hormones. However, neither GH nor cortisol changed after rhIGF-I injection, suggesting that during the present study conditions, the glucose-lowering effects of rhIGF-I are direct and not mediated via suppression of GH or cortisol.
Administration of rhIGF-I to healthy subjects causes a marked suppression of insulin (24, 25) and does not allow a clear interpretation of the glucose-lowering potential of IGF-I. Studying type 1 diabetic patients who are lacking endogenous insulin production under euglycemic clamp conditions circumvented these issues, and the clamp provided a continuous insulin infusion that stabilized insulin at a level where the IR may be saturated and, therefore, less likely to cross-react with IGF-I.
Local muscle IGF-I levels remained elevated and appeared to plateau toward the end of this study (4 hours). Our study does not determine whether there is a slow release to the tissues of circulating stores of IGF-I bound in the ternary complex with IGFBP-3 and the acid-labile subunit or whether IGF-I is stored locally bound to IGFBPs and the extracellular matrix. However, the finding of a sustained increase in circulating IGF-I for up to 4 hours supports the former explanation. In addition, a more prolonged increase in glucose utilization was seen in type 1 diabetic patients after injecting this high dose of rhIGF-I (120 μg/kg), compared with a peak approximately 2 hours after injection reported in SPIGFD subjects, who are also deficient in IGFBP-3 and acid-labile subunit and therefore have compromised ternary complex formation (37).
The md-IGF-I levels did not differ between muscle compartments and subcutaneous fat at baseline, but they increased to slightly higher levels in subcutaneous fat. Whether this observation is explained by a higher access of circulating IGF-I to subcutaneous fat that could result from a higher blood flow, by low expression of the IGF-IR in subcutaneous fat and thereby a decreased internalization and clearance, or by other factors, was not investigated.
We have previously evaluated the 20-kDa cutoff microdialysis probe and demonstrated that the in vitro recovery of IGF-I was approximately 10%, whereas neither intact nor fragmented IGFBPs crossed the probe (32, 33). Although the IGF-I assay was slightly modified in the current study, we found that baseline values are comparable with those of our previous studies in healthy subjects. The same is true of the peak md-IGF-I levels in interstitial muscle tissue after rhIGF-I injection, which were comparable to peak md-IGF-I levels in muscle after exercise (32). Thus, we believe we have obtained physiologically relevant tissue IGF-I levels. Desvigne et al (31) used a 60-kDa cutoff probe and reported free IGF-I concentrations in the muscle tissue that were 10-fold higher than the levels reported by us and also 10-fold higher than serum-free IGF-I levels in their own study. The existence of an IGF-I gradient from muscle (high) to circulation (low) implies that endocrine IGF-I does not significantly contribute to muscle glucose utilization, and this notion contrasts with our current findings.
The main goal for adjuvant rhIGF-I treatment in type 1 diabetes is to provide a reduction of the GH hypersecretion (38) and thereby to improve insulin sensitivity and metabolic control. In addition, rhIGF-I therapy reduces insulin requirements (17, 39). In future trials, it will be of interest to explore whether adolescents with type 1 diabetes show similar or even higher tissue md-IGF-I levels than healthy adolescents. If low-dose rhIGF-I therapy in type 1 diabetes suppresses GH hypersecretion and decreases tissue hyperinsulinemia, it may suppress, rather than increase, tissue md-IGF-I. In a lifelong perspective, these considerations may help to understand the involvement of tissue IGF-I in the development of diabetic microvascular and macrovascular complications (40).
We used the highest recommended dose of rhIGF-I (120 μg/kg) approved in SPIGFD (23) to increase the likelihood of obtaining detectable levels of md-IGF-I in this first physiological validation of the microdialysis technique. However, previous studies exploring the potential role of rhIGF-I as an adjuvant to intensive insulin treatment in children and adolescents with type 1 diabetes have shown that lower doses (40 μg/kg daily) are safe and efficient for lowering GH secretion, HbA1c, and insulin requirements over 4 to 12 weeks (18).
In conclusion, this study demonstrates that it is possible to measure tissue md-IGF-I after a single subcutaneous injection of rhIGF-I. Furthermore, our study demonstrates that md-IGF-I closely reflects a sustained increase in GIR by augmenting peripheral glucose utilization. Our data support the notion that measurement of local tissue IGF-I is physiologically relevant, and we suggest that future studies should focus on local tissue levels of IGF-I and its relation to metabolic control, endogenous insulin secretion, insulin sensitivity, and diabetic vascular complications.
Acknowledgments
We thank Eva Örtqvist and Jenny Salemyr who helped in recruiting subjects and Eric Rullman who gave practical assistance in planning the study.
Author contributions: K.E. and M.-A.P. contributed to the design and planned the study, performed the investigations, and wrote the manuscript. A.-L.B. assisted in the clamp studies. C.C.-S. did the assay measurements and, in collaboration with J.F. and Z.M., the IGF-I analysis. P.B. initiated, designed, and supervised the study. All authors revised/edited the manuscript. K.E., M.-A.P., and P.B. are the guarantors of this work and, as such, had full access to all the data in the study and take responsibility for the integrity of the data and the accuracy of the data analysis.
Results from this work were presented orally in abstract form at the Annual Meeting of the European Society for Pediatric Endocrinology, Leipzig, Germany, 2012, and at the Sixth International Congress of the GRS and IGF Society, Munich, Germany, 2012.
This study has been registered at ClinicalTrials.gov and with the European Clinical Trials Database (EudraCT number 2011-000233-37).
This work was supported by the Jalmari and Rauha Ahokas Foundation, the Samariten Foundation, the Swedish Child Diabetes Foundation (Barndiabetesfonden), the Foundation Frimurare Barnhuset in Stockholm, the Society for Child Care (Sällskapet Barnavård), and the HRH Crown Princess Lovisa Society for Medicine. K.E. received ALF project funding from the Stockholm County Council. M.A.P. received financial support from ISPAD, the Biomedicum Helsinki Foundation, and the Finnish Foundation for Pediatric Research. Z.M. is the recipient of an unrestricted grant for research from NovoNordisk (Bagsvaerd, Denmark). Ipsen (Kista, Sweden) gave an unconditional grant and provided rhIGF-I (Increlex).
Disclosure Summary: P.B. is a member of the advisory board for Ipsen and has received research grants from Ipsen. The other authors have nothing to disclose.
K.E. and M.-A.P. contributed equally to the study and are listed in alphabetic order.
Abbreviations
- ASF
abdominal subcutaneous fat
- CSII
continuous subcutaneous insulin infusion
- CV
coefficient of variation
- GIR
glucose infusion rate
- HbA1c
hemoglobin A1c
- IGFBP
IGF binding proteins
- IGF-1R
IGF-I receptor
- IR
insulin receptor
- LMVL
left musculus vastus lateralis
- md-IGF-I
microdialysis IGF-I
- rh
recombinant human
- RMVL
right musculus vastus lateralis
- SPIGF
severe primary IGF-I deficiency
- TR-IFMA
time-resolved immunofluorometric assay.