-
PDF
- Split View
-
Views
-
Cite
Cite
Eda Marie Barsalote-Wei, David Nichols, Robert Steven Tegg, Alieta Eyles, Annabel Jun-Chn Wilson, Calum Rae Wilson, Rhizosphere bacteria degrade a key root exudate metabolite critical for pathogen germination and root infection, Journal of Applied Microbiology, Volume 136, Issue 5, May 2025, lxaf090, https://doi.org/10.1093/jambio/lxaf090
- Share Icon Share
Abstract
Glutamine (Gln), present within potato root exudates, stimulates germination of resting spores and chemotactic attraction of zoospores of the plasmodiophorid pathogen, Spongospora subterranea. We hypothesized that rhizosphere bacteria could alter the rhizosphere metabolome by diminishing the occurrence of Gln with the eventual aim of reducing pathogen activation, attraction and infection. This study aimed to isolate and characterize bacteria capable of substantially degrading Gln within the potato rhizosphere.
Eleven bacteria were isolated from potato rhizosphere samples using Gln as a sole carbon source. Of these, Pantoea sp. (RR15) and Rhodococcus sp. (RR09) showed superior Gln degradation potential. Both isolates established within the potato rhizosphere and reduced Gln concentrations in situ. Further analysis of the rhizosphere metabolome showed significant treatment effects for a range of other organic compounds, including some known to stimulate or inhibit Spongospora subterranea germination and/or taxis.
We demonstrate that establishing selected bacteria in the rhizosphere of potatoes can successfully modify the root rhizosphere metabolome.
Plant-microbe rhizosphere interactions are facilitated by root exudate chemistry. The use of rhizosphere bacteria to modify the exudate/rhizosphere metabolome has the potential to interfere with soil-pathogen signalling and provide a novel approach to root disease management.
Introduction
The rhizosphere is the zone of soil that surrounds the plant root, supporting an extremely complex ecosystem that is essential for plant growth and defence against phytopathogen invasions (Lynch and Whipps 1990, Lodewyckx et al. 2002, Badri et al. 2009, Raaijmakers et al. 2009). Plants secrete a variety of low molecular weight organic compounds from their roots into the surrounding soil environment (Vives-Peris et al. 2020) to attract and support rhizosphere microbial populations (Bais et al. 2006, Leach et al. 2017, Zhalnina et al. 2018). These root exudates can function as chemoattractants, inhibitors, or stimulants of the soil microbiota, and are often important in pathogen propagule germination and host recognition by root-infecting pathogens (Nelson 1990, Steinkellner et al. 2005, Bais et al. 2006, Doornbos et al. 2011, Balendres et al. 2016a, Amponsah et al. 2021).
The plasmodiophorid plant pathogen, Spongospora subterranea f. sp. subterranea is responsible for root and tuber (powdery scab) disease in potatoes (Merz and Falloon 2009). Root infection has been associated with significant yield declines and powdery scab of tubers decreases tuber quality, both impacting the economic viability of the potato industries (Wilson 2016). The pathogenicity cycle of S. subterranea has been well characterized (Balendres et al. 2016b, Strydom et al. 2024). In brief, long-lived resting spores (present as aggregates within a sporosorus) present in cropping soils germinate in the presence of soil water, stimulated by root exudate compounds (Fornier et al. 1996, Balendres et al. 2016a). Released zoospores migrate to the host roots via chemotactic attraction to these same root exudates (Amponsah et al. 2023), to which they encyst and penetrate, facilitating infection. Infected cells produce a uninucleate plasmodium that develops into a multinucleate zoosporangium. This zoosporangium typically contains 2–4 zoospores, which can release secondary zoospores into the soil environment that can initiate further root and stolon infections (Merz 1997).
Germination of S. subterranea resting spores occurs much earlier and releases greater numbers of zoospores in the presence of root exudate stimulant compounds compared to water alone (Balendres et al. 2016a). The amino acid glutamine (Gln) has been showed to have one of the greatest stimulatory effects on S. subterranea resting spore germination (Balendres et al. 2016a) and zoospore taxis (Amponsah et al. 2023). These experiments (and other unpublished data) have confirmed Gln stimulatory activity with concentrations varying from 0.001 M to 0.68 M; however, a definitive study to determine the minimum Gln concentration required for germination and taxis stimulation is yet to be done.
The objective of this study was to identify, screen, and select rhizobacteria that demonstrated the potential to reduce concentrations of Gln within the potato root rhizosphere and thus indirectly inhibit root infection by decreasing pathogen resting spore germination and chemotactic signals. Enrichment cultures and ultra-performance liquid chromatography-tandem mass spectrometry (UPLC-MS/MS) techniques were used to identify bacterial strains capable of utilizing Gln as their sole carbon source. To the best of our knowledge, this is the first study to describe a selection of rhizosphere bacteria capable of degrading a key potato root exudate compound and thus altering the rhizosphere metabolome in situ as a target against germination and attraction of a plant pathogen. We propose that manipulation of the rhizosphere metabolome in this manner offers a novel and sustainable approach to plant pathogen biological control.
Materials and methods
Bacterial isolation from the potato rhizosphere
Rhizosphere samples were collected from five randomly selected plants within a commercial crop of Solanum tuberosum cv. ‘Ranger Russet’ growing in NW Tasmania (-41.17°S, 146.33°E). Potato plants were sampled at 75 days after planting. Plant roots were shaken to remove loosely adhering soil, the roots were rinsed four times in distilled water for 30 s each. The washed roots were then transferred into 50 mL Falcon tubes containing 30 mL phosphate-buffered saline solution (0.1 mol L-1), and remaining soil adhering to the roots was separated by agitation in a rotary shaker (180 rpm, 30 min) and centrifuged for 15 min at 25°C at 12 000 g. The supernatant was discarded, and the resultant rhizosphere soil pellet was collected and stored at 4°C until use (Barillot et al. 2013, Buermans and den Dunnen 2014).
To isolate bacteria, 0.2 g of the rhizosphere soil pellet was resuspended in 50 mL sterile minimal salt media (MSM) broth and then the mixture was centrifuged at 3000 g for 5 min. The supernatant was serially diluted with MSM from 10–1 to 10–8 CFU mL-1. An aliquot of 150 µL was spread onto 2.5% (w/v) MSM agar and amended with 1 mL of a filter sterilized 200 mmol L-1 stock of Gln. Plates were inverted and incubated in darkness at 18–23°C with colony growth monitored. Bacteria with morphologically distinct colonies and pigmentation were chosen for sub-culturing, purification, and re-streaking onto fresh agar plates.
A standard microbiological evaluation of each selected individual bacteria was done, which involved streaking on nutrient agar, macroscopic (Leica MZ12 stereomicroscope; Leica Microsystems GmbH, Wetzlar, Germany) and microscopic observation (Leica DMLB compound microscope) of colony and cell morphology via wet-mounting and Gram staining reaction (Krieg et al. 2010). Pure cultures were maintained in 50% glycerol and kept at −80°C.
Molecular genotyping of isolates
One colony of each bacterium was suspended in 10 mL Luria Bertani broth in a sterilized McCartney bottle and grown overnight with shaking. A 250 µL volume of broth was collected and genomic DNA extracted using the DNeasy ultra-clean microbial kit (Qiagen, MD, USA). DNA extracts were then used for 16S rRNA gene amplification with primers 515F: 5′-GTGCCAGCMGCGCGGTAA-3′ and 806R: 5′-GGACTACHVGGGTWTCTAAT-3′. The reactions were carried out using a thermocycling profile of 95°C (5 min) followed by 30 cycles of 95°C (1 min), 65°C (1 min), and 72°C (1.5 min); with a final extension of 72°C (10 min).
PCR products were visualized under blue light following electrophoresis in a 1% agarose gel containing and 1X SYBR™ Safe DNA gel stain (Invitrogen, MA, USA) and purified using a QIAquick PCR Purification Kit (Qiagen, MD, USA) following the manufacturer’s guidelines. The quality and concentration of purified PCR products were determined using the Qubit® 2.0 Fluorometer (Invitrogen, MA, USA) before submission to the Australian Genome Research Facility for DNA sequencing in both directions (http://www.agrf.org.au/).
Forward and reverse sequences were aligned, primer regions removed, and homology searches conducted of the GenBank DNA database (http://www.ncbi.nlm.nih.gov) using BLAST. Closest sequences were determined and tentative genera identified.
Screening bacterial isolates for growth on and degradation of Gln
Seven bacterial isolates from distinct genera that grew well with Gln as a carbon source were enriched separately by inoculating them into 100 mL of LB broth (10 g peptone, 5 g yeast, 5 g NaCl in 1 L of medium, pH 5.8) in 250 mL flasks in an orbit shaker set at 150 rpm and 23°C until OD600 was equal to 1 (Lami et al. 2020). Thereafter, bacterial cells were harvested by centrifugation at 6000 g for 5 min at 4°C. The procedure was then repeated three times with 1 mL of culture solution withdrawn and reconstituted in a fresh 100 mL MSM media, this was then utilized as the starting culture for further studies (Krishnan et al. 2018).
Relative growth and degradation potential of isolates was investigated by initially diluting inoculum of each isolate in MSM to 0.11 CFU mL-1. Inoculum (100 µL) of each isolate was then added to individual flasks containing 100 mL of MSM and 20 mmol L-1 Gln. Three replicate flasks per isolate were prepared. All flasks were incubated at 18–23°C on an orbital shaker set to 150 rpm. After 24, 48, 72, and 96 h incubation, a 1 mL aliquot of broth was removed from each flask to determine relative growth measured as turbidity via OD on a spectrophotometer (λ = 600 nm), and a further 10 mL sample of each culture solution was transferred to 15 mL Falcon tubes, centrifuged for 5 min at 12 000 g, and the supernatant tested for Gln content by reverse-phase ultra-pressure liquid chromatography-tandem mass spectrometry (UPLC-MS/MS) analysis.
Estimating potato root colonizing ability of isolates RR15 and RR09
The two bacterial isolates showing the greatest Gln degradation potential (RR15 and RR09) were further examined for their ability to colonize potato roots in an in vitro system as has been used previously to estimate colonization capacity (Achouak et al. 2004). The roots of 36 three-week-old axenically grown tissue-cultured potato plants of cv. Ranger Russet were suspended in 20 mL liquid potato multiplication (PM) medium (composed of Murashige and Skoog [MS] salts, 4.43 g L-1; sucrose, 30 g L-1; casein hydrolysate, 0.5 g L-1; ascorbic acid, pH 5.8) containing 108 CFU mL-1 of either bacterial isolate (18 plants for each isolate). Roots from three plants treated with each isolate were then sampled 1, 3, 6, 9, 12 and 15 days post inoculation.
External (root surface) bacterial colonization of the entire root was evaluated both microscopically and using CFU counts. The roots of each plant were cut and separated from the stem and fresh weight measured. Subsamples were examined microscopically by mounting roots segments in water and observation at 1000× magnification (Leica DMLB compound microscope), with presence of bacteria surrounding the root hairs noted. The remaining root samples were placed into 50 mL Falcon tubes containing 25 mL of sterile phosphate-buffered saline solution (pH 7.4). After vortexing the tubes for 5 min at 5000 g, the suspension was collected, and successive dilutions of the suspension were plated on LB agar (7.5 g agar, 5 g tryptone, 5 g NaCl, and 2.5 g yeast extract) and incubated at 18–25°C for 24–48 h before obtaining a CFU g-1 root tissue count.
Internal (endophytic) bacterial populations were assessed from the same roots. Roots were surface disinfected in three steps: a 60 s ethanol wash, a 6 min 1% w/v sodium hypochlorite wash, a 30 s ethanol wash and a final rinse in sterile distilled water. The roots were then macerated using a mortar and pestle, the homogenates transferred to a microcentrifuge tube, and total DNA extracted using the DNeasy Power Plant Pro kit (QIAGEN, MD, USA) following the manufacturer’s guidelines. To ensure standardized tissues extraction efficiency, an internal positive control was included in each sample prior to DNA extraction through the addition of 50 µL of 1.9 × 109 CFU mL-1 of the marine bacterium Pseudomonas prydzensis (Bowman 1998), not naturally present in terrestrial soils, that had been maintained and cultured on marine agar (peptone 5.0 g L-1, yeast extract 2.0 g L-1, NaCl 35.0 g L-1, agar 15.0 g L-1, pH 5.8).
Quantification of isolates RR09, RR15 and P. prydzensis was performed by TaqMan qPCR amplification of 250–300 bp products with primers and TaqMan probes designed from the 16S region of each isolate using the Primer QuestTM tool (https://sg.idtdna.com/PrimerQuest/Home/Index; Supplementary data). Primer and probe combinations of RR15-2f (ACGGCCGCAAGGTTAAA) and RR15-2r (GCTGGATGTCAAGAGTAGGTAAG) and probe RR15-2prb (ACAAGCGGTGGAGCATGTGGTTTA) were used to quantify isolate RR15; primers RR09-1f (CGGAATCACTGGGCGTAAA) and RR09-1r (GTTCCACCAACCTCTACCATAC), and probe RR09-1prb (CGGAATCACTGGGCGTAAA) were used to quantify isolate RR09; and forward primer (TCGGAATTACTGGGCGTAAAG), reverse primer (GAGTGTGATAGAGGGTGGTAGA) and probe (AGGCGGTTTGTTAAGCGAGATGTGA) were used to quantify P. prydzensis. qPCR was performed with 5 ng of bacterial DNA using a Rotor-Gene Q thermocycler (QIAGEN, Hilden, Germany). Three technical replicates per sample were run.
Absolute DNA quantification for each bacterium was achieved using a standard curve generated by qPCR with 10-fold serial dilutions from 108–102 copies per reaction of a gBlock fragment (Supplementary data) containing the targeted DNA fragment of the 16S rRNA gene of each bacterium. The cycle threshold (Ct) values of obtained from the standard curve were used to calculate the number of target DNA using the Rotor-Gene Q software package (Qiagen, Hilden, Germany).
Assessment of metabolite degradation by bacterial isolates in-situ
Two pot experiments were conducted to assess the capacity of bacterial isolates to diminish Gln within the potato root zone and surrounding soil in situ.
The first experiment tested the four bacterial isolates that showed the greatest capacity for Gln degradation (RR08, RR09, RR15, and RR17) and compared against an uninoculated control. Tissue-cultured potato plantlets (3 cm height with 3–4 fully expanded leaves) of cv. Ranger Russet were transferred into pots (17 cm × 19 cm) filled with an equal mix of 2.5 L of field soil (red Ferrosol, Australian Soil Classification; Isbell 2002) and potting mix (1:1:8 mix of peat, coarse sand, and composted pine bark). The trial consisted of five treatments (four bacterial treatments and the uninoculated control) with two collection times (8 and 16 weeks) and three replicates. The roots of bacterium-treated plants were dipped in inoculum suspensions (108 CFU mL-1 in liquid PM medium) of the individual bacteria for 5 h, while control seedlings’ roots were dipped in liquid PM medium only before transplanting. Pots were grown in a glasshouse maintained at 20 ± 2°C for 16 weeks. Three pots from each treatment were randomly selected for exudate collection at 8 and 16 weeks after planting. The day before root exudate collection, potted plants were watered until the soil was completely saturated. The next day, 300 mL of distilled water was poured into each pot and left to sit for 2 h. Leachate draining through the filtered bottom hole of the pot was collected (Fig 1) and 100 mL from each pot transferred into two 50 mL conical centrifuge tubes, agitated in a rotary shaker at 100 rpm for 10 min, and then centrifuged for 10 min at 5000 g. The supernatant containing water-soluble components was filter sterilized using a Merck Millipore™ membrane filter, 0.22 µm pore size (Merck KGaA, Darmstadt, Germany), then frozen and stored at −20°C before UPLC-MS/MS analysis of Gln content.
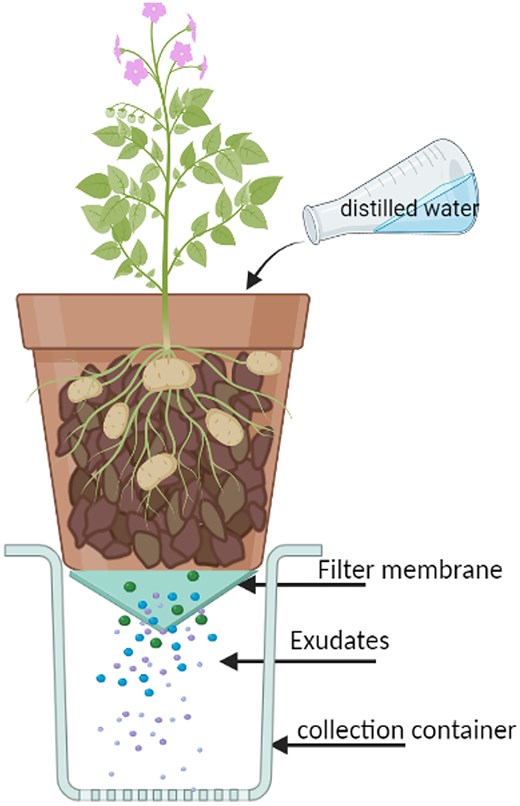
The second experiment retested the two isolates that showed the greatest Gln degradation in vitro and in situ (RR09 and RR15). In this trial, isolates were applied both individually and as a combined inoculum. Tissue culture plant material and potting mix were as in the prior experiment. This trial had three collection times with three replicates per sampling. Roots of treated plants were again dipped for 5 h in inoculum suspensions at 108 CFU mL-1 in liquid PM medium of the individual bacteria (RR15, RR09) or a mixture of both isolates (RR15 + RR09 each at 108 CFU mL-1) with control plants dipped in liquid PM medium only before transplanting. Pots were grown in a glasshouse maintained at 20 ± 2°C. Exudate collection and storage were as in the previous experiment with collections made at 4, 6, and 8 weeks after planting. Analysis was as before except UPLC-MS/MS analysis targeted an additional 15 amino acids and 23 other organic compounds previously described from potato root exudates (Balendres et al. 2016a) in addition to Gln.
In this second in situ experiment, bacterial establishment and persistence within the potato root rhizosphere and surrounding bulk soil following amendment was also assessed. Rhizosphere and bulk soil samples were taken at 2, 4, 6, and 8 weeks from three randomly selected pots of each treatment following inoculation and transplanting. Rhizosphere samples were prepared by removing loosely adhering soil and placing roots in 30 mL of distilled water in a Falcon tube and vortexing for 30 s. The roots were removed and the soil suspension centrifuged for 15 min at 25°C at 12 000 g. Bulk soil samples (5 g) were collected from a region of the pot without plant roots. The sample was sieved through a 2 mm mesh and 30 mL of sterile distilled water added followed by vortexing and centrifugation as for the rhizosphere sample. DNA was extracted from the resulting soil pellets using the DNeasy PowerSoil Pro kit (QIAGEN) following the manufacturer’s guidelines, and TaqMan qPCR with absolute quantification of isolates RR09 and RR15 based on gblock standards as described before, with three technical replicates per sample. Amplified bacterial DNA content was determined per 5 ng template DNA.
UPLC-MS/MS analysis
Detection of Gln and other primary metabolites directly from the runoff test solutions was achieved by using a HILIC UPLC-MS procedure derived from Gika et al. (2012). The UPLC was performed by using a Waters Acquity UPLC H-class system (Massachusetts, USA) and MS analysis using a Waters Xevo triple quadrupole MS system (Massachusetts, USA), respectively. The HILIC separation was performed on a 2.1 mm × 150 mm Acquity 1.7 µm BEH amide VanGuard column maintained at 60°C and eluted with a two-step gradient at 500 µL/min flow rate for 30 min. The mobile phases were composed of A (acetonitrile–water, 95–5 (v/v), 0.1% formic acid and 0.075% NH4OH), and B (acetonitrile–water, 2–98 (v/v), 0.2% formic acid and 0.1% NH4OH). LiChrosolv-grade acetonitrile was purchased from Merck (Germany), while formic acid (>95%) and NH4OH were purchased from Sigma–Aldrich (USA). The gradient started with a 4 min isocratic step at 100% mobile phase A, then rose to 28% mobile phase B over the next 21 min and finally to 60% B over 5 min 17. The column was then equilibrated for 12 min in the initial conditions. Two cycles of weak and strong solvent washing of the injection system were carried out between injections. The injection volume was 10 µL and the column eluent was directed to the mass spectrometer. Metabolite (compound) detection was performed by using selected ion monitoring (SIM) and an electrospray ionization (ESI) source was applied operating in both positive and negative ion modes. The parameters in the electrospray were set as follows: capillary voltage, −2.5 kV or 3 kV; cone and desolvation temperatures, 150 and 400°C, respectively, with a desolvation gas flow of 950 L/h and cone flow of 100 L/h. The cone voltage was optimized for each individual analyte. The analysis was operated using the MassLynxTM XS software 17. The LOD and LOQ of the analytes were determined at signal-to-noise ratios of 3 and 10, respectively. The repeatability of the HILIC UPLC-MS method was confirmed by the analysis of standard chemicals, root exudates and root exudates spiked with standard chemicals.
Statistical analyses
The mean differences between treatments were examined using repeated measures ANOVA with post-hoc pairwise Bonferroni tests using the R statistical language framework (Core Team; 2023) or 1-way and 2-way ANOVA using Genstat for Windows 23rd Edition (VSN International, Hemel Hempsted, UK) with Fisher’s Protected Least Significant Difference calculated for metabolite and bacterial colonization analyses. The values of the metabolite data were log10 transformed to meet assumptions of normality and homogeneity of variance in ANOVA using the R package MetaboAnalystR (https://www.metaboanalyst.ca/).
Results
Characterization of bacterial isolates
Eleven distinct bacterial isolates (RR01, RR05, RR08, RR09, RR10, RR12, RR13, RR15, RR16, and RR17) were obtained from the potato rhizosphere on minimal media amended with Gln as a sole carbon source.
The colony morphology of the bacterial isolates was generally round, raised, flat or convex, with a smooth or cottony colony surface and a spectrum of white, yellow and red pigments. Cellular morphology included cocci, rods and filamentous cell types, with cells occurring individually in chains, branched or a network or filaments. RR15 was Gram-negative with all others being Gram-positive. The actinomycete isolate RR12 was the only one that produced spores. Isolates cells varied in size from 0.3 to 0.9 µm in length and 0.5 to 1.2 µm in diameter (Supplementary Table 1).
Comparisons of 16S rRNA gene sequences to the GenBank database revealed closest homology to Staphylococcus sp. (isolates RR01, RR05, RR10), Bacillus sp. (RR08), Rhodococcus sp. (RR09), Streptomyces sp. (RR12), Pantoea sp. (RR13, RR15), Glutamicibacter sp. (RR14, RR16), and Brevibacterium sp. (RR17).
Isolate growth and ability to degrade target compounds in vitro
Bacteria in minimal media supplemented with 20 mmol L-1 Gln exhibited highly significant variation in their rate of growth over time (isolate: F6,12 = 4246.0, P < 0.001; time: F3,6 = 5791.4, P < 0.001; isolate x time interaction: F18,36 = 635.5, P < 0.001; Fig 2A), and in their capacity for Gln degradation over time (isolate: F6,12 = 7005.3, P < 0.001; time: F3,6 = 9328.8, P < 0.001; isolate x time interaction: F18,36 = 420.1, P < 0.001; Fig 2B). Pairwise comparisons showed significant differences in growth between all isolate pairs from 48 h incubation onwards except for the RR01 and RR12, RR08 and RR17, and RR12 and RR14 pairs. Similarly, pairwise analysis of times after 48 h incubation showed significant differences in Gln degradation rate between all isolates pairs except RR08 and RR12 (48–96 h), RR08 and RR14, RR12 and RR14 (48–72 h), RR1 and RR14 (72–96 h), and RR09 and RR15 (96 h only). Isolates RR09 and RR15 clearly demonstrated both the greatest growth and Gln degradation rate compared to other isolates, with isolates RR08 and RR17 also showing greater growth and isolate RR17 greater Gln degradation to the other three isolates tested (Fig. 2A and B). Gln levels were undetectable in the culture medium inoculated with RR15 after 48 h, or after 96 h with RR09. No other isolate reached this level of Gln degradation within the experimental period (Fig. 2B).
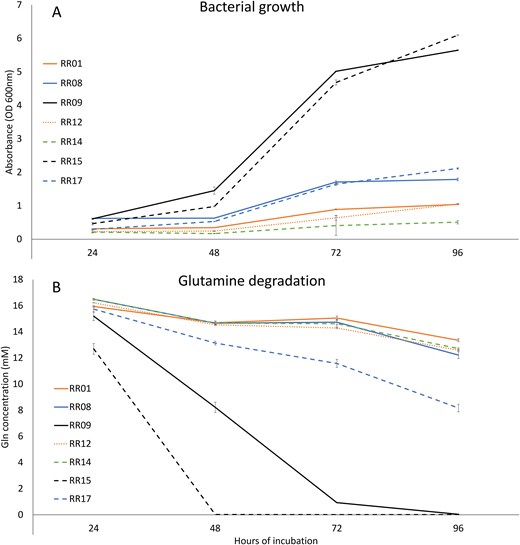
Relative bacterial growth on medium with Gln as the sole carbon source (A) as measured by culture turbidity, and relative Gln degradation (B) as measured by UPLC-MS/MS. Error bars are ± standard deviation.
Estimating root colonizing ability of RR15 and RR09 in in-vitro grown plants
Both bacteria successfully established populations within the potato root rhizosphere (Fig. 3A) and appeared to endophytically colonize root tissues (Fig. 3B). There were highly significant differences between isolates, time, and the interaction between isolate and time for both external rhizosphere bacterial populations (F2,4 = 75562.6, P < 0.001; F5,10 = 1882.2, P < 0.001; F10,20 = 789.0, P < 0.001) and internal endophyte populations (F2,4 = 6266.6, P < 0.001; F5,10 = 436.1, P < 0.001; F10,20 = 84.7, P < 0.001). Pairwise comparisons showed significant differences between all isolate pairs at all time points with isolate RR15 developing more abundant populations both in the rhizosphere and within the potato roots than RR09. After 15 days the bacterial populations were still increasing within the rhizosphere, but endophytic populations largely plateaued after 6 days for both isolates (Fig. 3A and B). Microscopic observation of roots confirmed presence of bacterial colonies on the surface of root hairs (Fig. 3C, D, and E). RR15 often colonized root hair tips, while RR09 was generally found only at the base of root hairs.
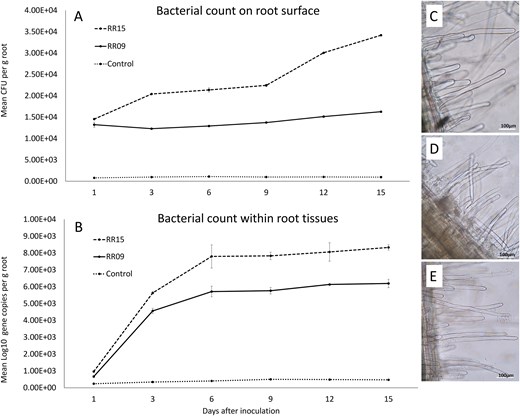
Bacterial colonization efficiency of isolates RR09 and RR15 on potato root surface (A) and within root tissues (B) following in vitro inoculation. Error bars are ± standard deviation. Microscopic images (1000× magnification) of potato roots showing bacterial colonization 8 weeks after inoculations: (C) uninoculated control (D) RR09 inoculated, and (E) RR15 inoculated.
Inoculant capacity to diminish Gln content in root zone in situ
In the first in situ experiment, analysis of water runoff from plants treated with all four (RR08, RR09, RR15, and RR17) bacteria showed less average Gln content (11.2–16.9 ng mL-1) than the control pot (26.7 ng mL-1) with a significant isolate effect (F4,4 = 11.0, P = 0.020; Fig 4A). Individual pairwise comparisons show significant differences between the uninoculated control and RR09 and the control and RR15 only. Time of collection (F1,1 = 10.3, P = 0.193) and interactions between isolate and collection time (F4,4 = 0.21, P = 0.921) were not significant. Of the tested bacteria, isolates RR09 and RR15 showed the greatest Gln degradation capacity with a 4–5-fold reduction in Gln content compared to the control at 16 weeks.
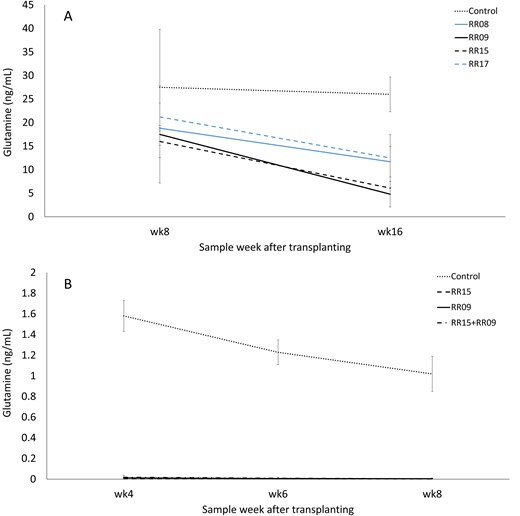
Gln content in runoff water from pot-grown potato plants treated with bacterial inoculants. Trial 1 (A) with four isolates and trial 2 (B) with two isolates applied individually or as a combined inocula. Error bars are ± standard deviation.
In the second experiment, isolate (F3,6 = 528.3, P < 0.001) and the interactions between isolates and collection time (F6,12 = 4.9, P = 0.009) showed significant differences while the collection time component was non-significant (F2,4 = 5.5, P = 0.07; Fig 4B). Of note, the average amount of Gln detected from control pots was ∼16-fold lower than in the previous experiment (1.7 ng mL-1). Pairwise comparisons showed both isolates either individually or as a combined inoculum, were significantly different from the control at all three collection times. All bacterial treatments (RR09, RR15 and the combination treatment) reduced Gln to near undetectable levels (0.0–0.01 ng mL-1, a 100-fold decrease from the control; Fig 4B).
The RR15 and RR09 individual and combination inoculant treatments had additional, and varied impact on other amino acids and organic compounds measured from the collected pot leachates (Table 1). Several amino acids (threonine, serine, alanine, arginine, histidine, methionine, and lysine), organic compounds (piperidine, tartaric acid, malic acid, syringic acid, and gluconic acid), and sugars (ribose C5, sucrose disaccharide, and glucose C6) tested for, were not detected in either the control or treated groups in this assay (Table 1).
Metabolite content of runoff water from pot-grown potato plants treated with bacterial inoculants.
Compounda . | . | . | Bacterial inoculant treatmentd . | . | . | |||
---|---|---|---|---|---|---|---|---|
Amino acids . | Resting spore germinationb . | Zoospore chemotaxisc . | Control . | RR15 . | RR09 . | RR15 + RR09 . | P . | l.s.d . |
Alanine | (-)e | (-) | (-) | (-) | n/ae | |||
Arginine | (-) | (-) | (-) | (-) | n/a | |||
Aspartic acid | + | (-) | 3.23 | (-) | (-) | 0.436 | ||
Glutamine | + | + | 1.7 | 0.0 | 0.01 | 0.01 | <0.001 | 0.649 |
Glycine | 3.1 | 5.8 | (-) | 4.3 | 0.464 | |||
Histidine | (-) | (-) | (-) | (-) | n/a | |||
Isoleucine | N | N | 1.0 | 0.6 | (-) | 0.2 | 0.013 | 0.559 |
Leucine | 0.7 | 0.7 | (-) | 0.2 | 0.029 | 0.498 | ||
Lysine | (-) | (-) | (-) | (-) | n/a | |||
Methionine | (-) | (-) | (-) | (-) | n/a | |||
Phenylalanine | 8.8 | 8.0 | 7.8 | 7.7 | <0.001 | 0.243 | ||
Proline | N | + | 0.8 | 0.7 | 0.3 | 0.2 | 0.039 | 0.486 |
Serine | + | + | (-) | (-) | (-) | (-) | n/a | |
Threonine | (-) | (-) | (-) | (-) | n/a | |||
Tyramine | + | + | 2.9 | (-) | 0.9 | (-) | 0.106 | |
Tyrosine | 0.8 | 0.6 | 1.5 | (-) | 0.014 | 0.811 | ||
Valine | 1.0 | (-) | 0.3 | 0.1 | <0.001 | 0.399 | ||
Other organic compounds | ||||||||
3,4-dihydroxybenzoic acid | 21.1 | 2.8 | 7.2 | (-) | 0.141 | |||
Caffeic acid | 99.0 | 27.8 | 23.8 | 7.5 | 0.036 | 61.70 | ||
Chlorogenic acid | 422.0 | 43.0 | 99.0 | 20.0 | 0.018 | 250.8 | ||
Choline | - | 9.4 | 13.9 | 10.7 | 4.3 | 0.334 | ||
Citric acid | + | 14.9 | (-) | (-) | (-) | 0.012 | 9.25 | |
Citrulline | + | 1.31 | 0.63 | 0.88 | (-) | 0.753 | ||
Gallic acid | 38.2 | 3.4 | 8.1 | (-) | 0.002 | 16.84 | ||
Gluconic acid | (-) | (-) | (-) | (-) | n/a | |||
Glucose C6 | (-) | (-) | (-) | (-) | n/a | |||
Guanidine | (-) | 31.8 | 17.4 | 7.7 | 0.097 | |||
Lactic acid | N | (-) | 396.0 | 178.0 | 137.0 | 0.022 | 227.2 | |
Malic acid | (-) | (-) | (-) | (-) | n/a | |||
Nicotinamide | + | 13.4 | 8.6 | 7.7 | 5.4 | 0.759 | ||
Piperidine | (-) | (-) | (-) | (-) | n/a | |||
Putrescine | 10.8 | 36.9 | 30.9 | 15.8 | <0.001 | 10.12 | ||
Pyridoxal | 10.3 | 4.0 | 3.4 | 2.2 | 0.112 | |||
Ribose C5 | (-) | (-) | (-) | (-) | n/a | |||
Spermidine | N | 58.0 | 33.5 | 17.1 | 4.5 | 0.171 | ||
Spermine | N | - | 63.3 | 25.1 | 21.3 | 19.7 | <0.001 | 17.31 |
Succinic acid | + | (-) | 396.0 | 365.0 | 390.0 | 0.174 | ||
Sucrose disaccharide | (-) | (-) | (-) | (-) | n/a | |||
Syringic acid | (-) | (-) | (-) | (-) | n/a | |||
Tartaric acid | (-) | (-) | (-) | (-) | n/a | |||
Thiamine | 13.2 | 8.5 | (-) | (-) | 0.263 |
Compounda . | . | . | Bacterial inoculant treatmentd . | . | . | |||
---|---|---|---|---|---|---|---|---|
Amino acids . | Resting spore germinationb . | Zoospore chemotaxisc . | Control . | RR15 . | RR09 . | RR15 + RR09 . | P . | l.s.d . |
Alanine | (-)e | (-) | (-) | (-) | n/ae | |||
Arginine | (-) | (-) | (-) | (-) | n/a | |||
Aspartic acid | + | (-) | 3.23 | (-) | (-) | 0.436 | ||
Glutamine | + | + | 1.7 | 0.0 | 0.01 | 0.01 | <0.001 | 0.649 |
Glycine | 3.1 | 5.8 | (-) | 4.3 | 0.464 | |||
Histidine | (-) | (-) | (-) | (-) | n/a | |||
Isoleucine | N | N | 1.0 | 0.6 | (-) | 0.2 | 0.013 | 0.559 |
Leucine | 0.7 | 0.7 | (-) | 0.2 | 0.029 | 0.498 | ||
Lysine | (-) | (-) | (-) | (-) | n/a | |||
Methionine | (-) | (-) | (-) | (-) | n/a | |||
Phenylalanine | 8.8 | 8.0 | 7.8 | 7.7 | <0.001 | 0.243 | ||
Proline | N | + | 0.8 | 0.7 | 0.3 | 0.2 | 0.039 | 0.486 |
Serine | + | + | (-) | (-) | (-) | (-) | n/a | |
Threonine | (-) | (-) | (-) | (-) | n/a | |||
Tyramine | + | + | 2.9 | (-) | 0.9 | (-) | 0.106 | |
Tyrosine | 0.8 | 0.6 | 1.5 | (-) | 0.014 | 0.811 | ||
Valine | 1.0 | (-) | 0.3 | 0.1 | <0.001 | 0.399 | ||
Other organic compounds | ||||||||
3,4-dihydroxybenzoic acid | 21.1 | 2.8 | 7.2 | (-) | 0.141 | |||
Caffeic acid | 99.0 | 27.8 | 23.8 | 7.5 | 0.036 | 61.70 | ||
Chlorogenic acid | 422.0 | 43.0 | 99.0 | 20.0 | 0.018 | 250.8 | ||
Choline | - | 9.4 | 13.9 | 10.7 | 4.3 | 0.334 | ||
Citric acid | + | 14.9 | (-) | (-) | (-) | 0.012 | 9.25 | |
Citrulline | + | 1.31 | 0.63 | 0.88 | (-) | 0.753 | ||
Gallic acid | 38.2 | 3.4 | 8.1 | (-) | 0.002 | 16.84 | ||
Gluconic acid | (-) | (-) | (-) | (-) | n/a | |||
Glucose C6 | (-) | (-) | (-) | (-) | n/a | |||
Guanidine | (-) | 31.8 | 17.4 | 7.7 | 0.097 | |||
Lactic acid | N | (-) | 396.0 | 178.0 | 137.0 | 0.022 | 227.2 | |
Malic acid | (-) | (-) | (-) | (-) | n/a | |||
Nicotinamide | + | 13.4 | 8.6 | 7.7 | 5.4 | 0.759 | ||
Piperidine | (-) | (-) | (-) | (-) | n/a | |||
Putrescine | 10.8 | 36.9 | 30.9 | 15.8 | <0.001 | 10.12 | ||
Pyridoxal | 10.3 | 4.0 | 3.4 | 2.2 | 0.112 | |||
Ribose C5 | (-) | (-) | (-) | (-) | n/a | |||
Spermidine | N | 58.0 | 33.5 | 17.1 | 4.5 | 0.171 | ||
Spermine | N | - | 63.3 | 25.1 | 21.3 | 19.7 | <0.001 | 17.31 |
Succinic acid | + | (-) | 396.0 | 365.0 | 390.0 | 0.174 | ||
Sucrose disaccharide | (-) | (-) | (-) | (-) | n/a | |||
Syringic acid | (-) | (-) | (-) | (-) | n/a | |||
Tartaric acid | (-) | (-) | (-) | (-) | n/a | |||
Thiamine | 13.2 | 8.5 | (-) | (-) | 0.263 |
Compounds tested by UPLC-MS/MS analyses from runoff water collected from pots with potato plants (cv. Russet Ranger) with or without bacterial inoculant treatments.
Compound has demonstrated ability to stimulate S. subterranea resting spore germination (+) or not (N), blank means not as yet tested (Balendres et al. 2016a).
Compound has demonstrated ability to attract (+), inhibit (-) or not influence S. subterranea zoospore chemotaxis, blank means not as yet tested (Amponsah et al. 2023).
Control = no inoculant; RR15 = Pantoea sp. inoculant; RR09 = Rhodococcus sp inoculant; RR15 + RR09 = combination of both bacterial isolates.
Data are mean compound content within runoff collection water across all sample times (ng mL-1); (-) = below limit of detection; n/a = no analyses conducted
Metabolite content of runoff water from pot-grown potato plants treated with bacterial inoculants.
Compounda . | . | . | Bacterial inoculant treatmentd . | . | . | |||
---|---|---|---|---|---|---|---|---|
Amino acids . | Resting spore germinationb . | Zoospore chemotaxisc . | Control . | RR15 . | RR09 . | RR15 + RR09 . | P . | l.s.d . |
Alanine | (-)e | (-) | (-) | (-) | n/ae | |||
Arginine | (-) | (-) | (-) | (-) | n/a | |||
Aspartic acid | + | (-) | 3.23 | (-) | (-) | 0.436 | ||
Glutamine | + | + | 1.7 | 0.0 | 0.01 | 0.01 | <0.001 | 0.649 |
Glycine | 3.1 | 5.8 | (-) | 4.3 | 0.464 | |||
Histidine | (-) | (-) | (-) | (-) | n/a | |||
Isoleucine | N | N | 1.0 | 0.6 | (-) | 0.2 | 0.013 | 0.559 |
Leucine | 0.7 | 0.7 | (-) | 0.2 | 0.029 | 0.498 | ||
Lysine | (-) | (-) | (-) | (-) | n/a | |||
Methionine | (-) | (-) | (-) | (-) | n/a | |||
Phenylalanine | 8.8 | 8.0 | 7.8 | 7.7 | <0.001 | 0.243 | ||
Proline | N | + | 0.8 | 0.7 | 0.3 | 0.2 | 0.039 | 0.486 |
Serine | + | + | (-) | (-) | (-) | (-) | n/a | |
Threonine | (-) | (-) | (-) | (-) | n/a | |||
Tyramine | + | + | 2.9 | (-) | 0.9 | (-) | 0.106 | |
Tyrosine | 0.8 | 0.6 | 1.5 | (-) | 0.014 | 0.811 | ||
Valine | 1.0 | (-) | 0.3 | 0.1 | <0.001 | 0.399 | ||
Other organic compounds | ||||||||
3,4-dihydroxybenzoic acid | 21.1 | 2.8 | 7.2 | (-) | 0.141 | |||
Caffeic acid | 99.0 | 27.8 | 23.8 | 7.5 | 0.036 | 61.70 | ||
Chlorogenic acid | 422.0 | 43.0 | 99.0 | 20.0 | 0.018 | 250.8 | ||
Choline | - | 9.4 | 13.9 | 10.7 | 4.3 | 0.334 | ||
Citric acid | + | 14.9 | (-) | (-) | (-) | 0.012 | 9.25 | |
Citrulline | + | 1.31 | 0.63 | 0.88 | (-) | 0.753 | ||
Gallic acid | 38.2 | 3.4 | 8.1 | (-) | 0.002 | 16.84 | ||
Gluconic acid | (-) | (-) | (-) | (-) | n/a | |||
Glucose C6 | (-) | (-) | (-) | (-) | n/a | |||
Guanidine | (-) | 31.8 | 17.4 | 7.7 | 0.097 | |||
Lactic acid | N | (-) | 396.0 | 178.0 | 137.0 | 0.022 | 227.2 | |
Malic acid | (-) | (-) | (-) | (-) | n/a | |||
Nicotinamide | + | 13.4 | 8.6 | 7.7 | 5.4 | 0.759 | ||
Piperidine | (-) | (-) | (-) | (-) | n/a | |||
Putrescine | 10.8 | 36.9 | 30.9 | 15.8 | <0.001 | 10.12 | ||
Pyridoxal | 10.3 | 4.0 | 3.4 | 2.2 | 0.112 | |||
Ribose C5 | (-) | (-) | (-) | (-) | n/a | |||
Spermidine | N | 58.0 | 33.5 | 17.1 | 4.5 | 0.171 | ||
Spermine | N | - | 63.3 | 25.1 | 21.3 | 19.7 | <0.001 | 17.31 |
Succinic acid | + | (-) | 396.0 | 365.0 | 390.0 | 0.174 | ||
Sucrose disaccharide | (-) | (-) | (-) | (-) | n/a | |||
Syringic acid | (-) | (-) | (-) | (-) | n/a | |||
Tartaric acid | (-) | (-) | (-) | (-) | n/a | |||
Thiamine | 13.2 | 8.5 | (-) | (-) | 0.263 |
Compounda . | . | . | Bacterial inoculant treatmentd . | . | . | |||
---|---|---|---|---|---|---|---|---|
Amino acids . | Resting spore germinationb . | Zoospore chemotaxisc . | Control . | RR15 . | RR09 . | RR15 + RR09 . | P . | l.s.d . |
Alanine | (-)e | (-) | (-) | (-) | n/ae | |||
Arginine | (-) | (-) | (-) | (-) | n/a | |||
Aspartic acid | + | (-) | 3.23 | (-) | (-) | 0.436 | ||
Glutamine | + | + | 1.7 | 0.0 | 0.01 | 0.01 | <0.001 | 0.649 |
Glycine | 3.1 | 5.8 | (-) | 4.3 | 0.464 | |||
Histidine | (-) | (-) | (-) | (-) | n/a | |||
Isoleucine | N | N | 1.0 | 0.6 | (-) | 0.2 | 0.013 | 0.559 |
Leucine | 0.7 | 0.7 | (-) | 0.2 | 0.029 | 0.498 | ||
Lysine | (-) | (-) | (-) | (-) | n/a | |||
Methionine | (-) | (-) | (-) | (-) | n/a | |||
Phenylalanine | 8.8 | 8.0 | 7.8 | 7.7 | <0.001 | 0.243 | ||
Proline | N | + | 0.8 | 0.7 | 0.3 | 0.2 | 0.039 | 0.486 |
Serine | + | + | (-) | (-) | (-) | (-) | n/a | |
Threonine | (-) | (-) | (-) | (-) | n/a | |||
Tyramine | + | + | 2.9 | (-) | 0.9 | (-) | 0.106 | |
Tyrosine | 0.8 | 0.6 | 1.5 | (-) | 0.014 | 0.811 | ||
Valine | 1.0 | (-) | 0.3 | 0.1 | <0.001 | 0.399 | ||
Other organic compounds | ||||||||
3,4-dihydroxybenzoic acid | 21.1 | 2.8 | 7.2 | (-) | 0.141 | |||
Caffeic acid | 99.0 | 27.8 | 23.8 | 7.5 | 0.036 | 61.70 | ||
Chlorogenic acid | 422.0 | 43.0 | 99.0 | 20.0 | 0.018 | 250.8 | ||
Choline | - | 9.4 | 13.9 | 10.7 | 4.3 | 0.334 | ||
Citric acid | + | 14.9 | (-) | (-) | (-) | 0.012 | 9.25 | |
Citrulline | + | 1.31 | 0.63 | 0.88 | (-) | 0.753 | ||
Gallic acid | 38.2 | 3.4 | 8.1 | (-) | 0.002 | 16.84 | ||
Gluconic acid | (-) | (-) | (-) | (-) | n/a | |||
Glucose C6 | (-) | (-) | (-) | (-) | n/a | |||
Guanidine | (-) | 31.8 | 17.4 | 7.7 | 0.097 | |||
Lactic acid | N | (-) | 396.0 | 178.0 | 137.0 | 0.022 | 227.2 | |
Malic acid | (-) | (-) | (-) | (-) | n/a | |||
Nicotinamide | + | 13.4 | 8.6 | 7.7 | 5.4 | 0.759 | ||
Piperidine | (-) | (-) | (-) | (-) | n/a | |||
Putrescine | 10.8 | 36.9 | 30.9 | 15.8 | <0.001 | 10.12 | ||
Pyridoxal | 10.3 | 4.0 | 3.4 | 2.2 | 0.112 | |||
Ribose C5 | (-) | (-) | (-) | (-) | n/a | |||
Spermidine | N | 58.0 | 33.5 | 17.1 | 4.5 | 0.171 | ||
Spermine | N | - | 63.3 | 25.1 | 21.3 | 19.7 | <0.001 | 17.31 |
Succinic acid | + | (-) | 396.0 | 365.0 | 390.0 | 0.174 | ||
Sucrose disaccharide | (-) | (-) | (-) | (-) | n/a | |||
Syringic acid | (-) | (-) | (-) | (-) | n/a | |||
Tartaric acid | (-) | (-) | (-) | (-) | n/a | |||
Thiamine | 13.2 | 8.5 | (-) | (-) | 0.263 |
Compounds tested by UPLC-MS/MS analyses from runoff water collected from pots with potato plants (cv. Russet Ranger) with or without bacterial inoculant treatments.
Compound has demonstrated ability to stimulate S. subterranea resting spore germination (+) or not (N), blank means not as yet tested (Balendres et al. 2016a).
Compound has demonstrated ability to attract (+), inhibit (-) or not influence S. subterranea zoospore chemotaxis, blank means not as yet tested (Amponsah et al. 2023).
Control = no inoculant; RR15 = Pantoea sp. inoculant; RR09 = Rhodococcus sp inoculant; RR15 + RR09 = combination of both bacterial isolates.
Data are mean compound content within runoff collection water across all sample times (ng mL-1); (-) = below limit of detection; n/a = no analyses conducted
Compound content in the leachates was significantly decreased by both individual and combination inoculant treatments for Glu, phenylalanine, valine, caffeic acid, chlorogenic acid, citric acid, gallic acid and spermine. Significant decreases in isoleucine, leucine and proline content were also seen with the RR09 and combination treatments only, and tyrosine with the combination treatment only. Lactic acid content was significantly increased by all inoculant treatments, as was putrescine content in both individual but not the combination treatments (Table 1).
Bacterial colonization by isolates RR09 and RR15 of the potato root rhizosphere and surrounding bulk soil was demonstrated (Fig 5A–F). Isolate RR15, when applied as a sole inoculant to roots prior to planting showed substantial rhizosphere colonization (2.9 × 105–5.5 × 105 ng amplified bacterial DNA/reaction), which significantly increased over the period of study (F3,6 = 60.55, P < 0.001; Fig 5A). RR15 also established within the bulk soil with a peak population detected at 2 weeks followed by a modest but significant decline over time (F3,6 = 29.15, P < 0.001; Fig 5B). RR09 also appeared to establish populations within the rhizosphere, but these were much lower than RR15 (0.013–0.78 ng DNA/reaction), which peaked at the first measurement (2 weeks) and then significantly declined (F3,12 = 11.03, P < 0.001; Fig 5C). A similar pattern of a relatively small population significantly declining over time was seen in the bulk soil samples (F3,11 = 8.52, P = 0.014; Fig 5D). In the co-inoculation treatment, RR15 established greater populations within the rhizosphere than RR09 (F1,14 = 9.52, P = 0.008) although titres were less than in the single inoculation treatments (Fig 5E). In the bulk soil in co-inoculated pots, RR09 was undetectable, while RR15 had significantly greater populations (F1,14 = 39.17, P < 0.001) similar to that observed in the single inoculation treatment (Fig 5F).
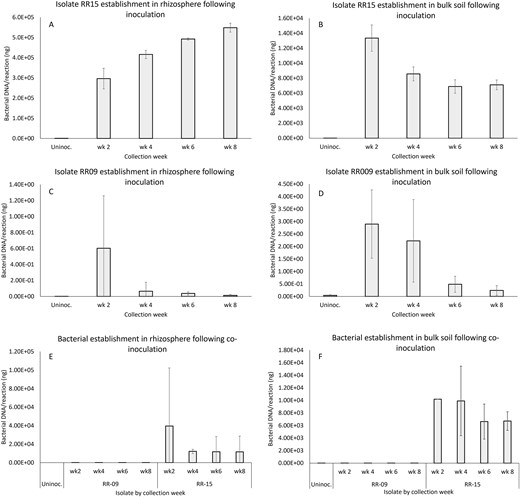
Establishment and persistence of bacterial populations of isolates RR09 and RR15 in rhizosphere and bulk soil samples in pot culture measured by TaqMan qPCR following pretreatment of transplanted plantlet roots with a single or combined inoculum of each bacterium. Error bars are ± standard deviation.
Discussion
Exudates from plant roots attract and support beneficial soil bacteria that aid plants either directly by biosynthesizing antimicrobial compounds or indirectly by inducing systemic resistance in the plants (Haichar et al. 2008, 2014). Root exudates can also stimulate and attract soil-borne plant pathogens encouraging subsequent pathogenesis. For example, Balendres et al. (2016) found that Gln and certain other root exudate compounds from potatoes, were important in stimulating the germination of S. subterranea resting spores, and Amponsah et al. (2023) further showed Gln exudates will attract S. subterranea zoospores to potato roots. Here, we sought to identify rhizosphere bacteria capable of efficiently degrading Gln as a critical pathogen stimulant with the eventual aim of interference of pathogen activation, attraction and infection.
In the present study, we isolated 11 bacteria from the potato rhizosphere capable of growth on minimal media supplemented with Gln as the sole carbon source. Auto-degradation of Gln in sterile solutions can occur with 0.2%–0.8% loss per day at 22–24°C noted depending on the medium (Khan and Elia 1991). This level of loss is much less than observed for any of the bacterial isolates tested in our trial. Of the bacteria tested, two (RR15 and RR09) showed the greatest capacity for rapid and complete degradation of Gln. Morphological and molecular identification revealed that isolates RR15 and RR09 were members of the genera Pantoea and Rhodococcus, respectively.
Pantoea species are Gram-negative, yellow-pigmented bacteria that belong to the Enterobacteriaceae family and include a broad range of species that have been isolated from diverse environments (Walterson and Stavrinides 2015). Rhodococcus species are aerobic non-sporulating, non-motile, Gram-positive members of the Nocardiaceae family (Johnson and Stockwell 1998). Both Pantoea and Rhodococcus species are predominant members of the soil community within the Proteobacteria and Actinobacteria, respectively, which have been documented to possess high capabilities for degradation of enzymes and antifungal metabolites (Blaszack et al. 2011, Zeng et al. 2016). Several Pantoea isolates have been developed into commercial biocontrol agents, with activity against pathogens by competitive niche exclusion, antibiotic production, and an ability to induce plant systemic resistance (Johnson and Stockwell 1998, Stockwell et al. 2002, Smits et al. 2019, Zheng et al. 2021). Pantoea species also exhibit remarkable environmental versatility and adaptation, as well as a wide range of biosynthetic and biodegradative capacities (Walterson and Stavrinides 2015). Some Rhodococcus isolates have also been investigated for commercial purposes due to their unique capacity to degrade highly stable and persistent environmental contaminants, making them ideal for biocatalytic and bioremediation processes (Kuyukina and Ivshina 2010, Tyagi et al. 2011).
We identified several Gram-positive degrading bacteria in our study; however, the Gram-negative Pantoea isolate demonstrated the overall greatest efficacy at targeted metabolite degradation. Bacterial degradation pathways often involve enzymes coded by conserved gene clusters (e.g. Ghosal et al. 2016, Malhotra et al. 2021), which are mostly found in Gram-negative bacteria.
Root colonization is one of the most crucial stages in the interaction between bacteria and their hosts. In this study, it was observed that within 24 h, bacterial cell colonization was apparent on root surfaces at the elongation zone, which has been similarly found in bacteria from the genera Bacillus sp. and Rhizobium sp. (Massalha et al. 2017, Pini et al. 2017). Results showed that isolate RR15 was capable of rapid and efficient root colonization as early as 24 h after inoculation and achieved a maximum of 3.4 × 104 CFU g-1 root by day 15. This finding is comparable to that of Soluch et al. (2021), who found that a Pantoea sp. colonized wheat roots rapidly across individual plants 5 h after inoculation and increased considerably in population size over the course of the experiment, eventually reaching a stable density of 5.1 × 107 CFU g-1 root between 8 and 15 days after inoculation. Wheatley and Poole (2018) suggest that the Pantoea populations are capable of rapid, consistent, and robust colonization, most likely mediated by pili and attachment proteins in combination with or in response to plant chemical signals. The successful establishment of rhizosphere and bulk soil populations of our Pantoea sp. (RR15) in pot culture, following inoculation of transplants prior to planting, further supports the capacity of this isolate to efficiently associate and persist with potato roots and the surrounding soil environment.
The in-situ pot trial demonstrated clear changes in the chemical composition of root exudates following bacterial inoculation by week 8. This finding is consistent with previous studies that also demonstrated changes in metabolite composition of the whole plant by specific soil microbes, indicating a bi-directional interaction between root and rhizosphere microbial populations (Curzi et al. 2008, Fernandez et al. 2012).
Where significant changes in metabolite content between treatments were found, these mostly reflected depletion of metabolites in the presence of bacterial inoculants. Of those compounds, Gln, proline and citric acid are known stimulants of S. subterranea resting spore germination and/or chemoattractants of zoospores, and thus their significant reduction may aid inhibition of pathogen activation and infection (Balendres et al. 2016a, Amponsah et al. 2021). On the other hand, spermine, a known S. subterranea zoospore chemotaxis inhibitor (Amponsah et al. 2021), content was also reduced compared to the control and thus could negatively impact disease mitigation. Several known stimulatory materials were either undetectable (serine), not significantly changed (aspartic acid, nicotinamide and succinic acid), or not tested in this analysis (e.g. asparagine, pinitol, raffinose, trehalose, rhamnose, cellobiose, N-acetyl-cysteine, piperazine, and glucuronic acid). The impact of significantly reducing isoleucine, leucine, phenylalanine, tyrosine, valine, caffeic acid, chlorogenic acid, and gallic acid and increasing lactic acid and putrescine on pathogen biology by one or both bacterial inoculants is currently unknown but worthy of future testing. In particular, the increasing content of putrescine is interesting, as this is a precursor molecule for polyamines which are involved in plant immunity against disease but can also be produced by pathogens to manipulate defence (Gerlin et al. 2021).
While this work has targeted chemical signals that exacerbate S. subterranea infection, we note that alteration of the rhizosphere metabolome may also affect other beneficial and pathogenic organisms either positively or negatively, and this would be worthy of further investigation. Also, in addition to any direct effect of the inoculants on these compounds, there could be indirect effects resulting from a close interaction of RR15 and/or RR09 with other competing microorganisms in the soil, which could affect the production of secondary metabolites, as has been previously shown with Streptomyces spp. (Tyc et al. 2017).
In conclusion, this study demonstrated the successful isolation and characterization of two rhizosphere bacteria (Pantoea sp. RR15 and Rhodococcus sp. RR09) that showed an affinity for the potato root rhizosphere and demonstrated a strong capacity to degrade Gln, an important pathogen-stimulating compound within potato root exudates. The targeted degradation of Gln that both stimulates pathogen resting spore germination and directs zoospore chemotaxis offers a novel approach to biological control of soil-borne disease through manipulation of soil chemical ecology. Evaluation of the impact of these rhizosphere bacteria on plant growth and disease expression will be examined in future studies.
Author contributions
Eda Marie Barsalote-Wei (Data curation, Formal analysis, Investigation, Methodology, Writing – original draft, Writing – review & editing), David Nichols (Conceptualization, Data curation, Formal analysis, Funding acquisition, Methodology, Supervision, Validation, Writing – review & editing), Robert Steven Tegg (Supervision, Writing – review & editing), Alieta Eyles (Supervision, Writing – review & editing), Annabel Jun-Chn Wilson (Methodology, Supervision), and Calum Rae Wilson (Conceptualization, Data curation, Formal analysis, Funding acquisition, Project administration, Supervision, Validation, Writing – review & editing)
Conflict of interest
None declared.
Funding
This research was supported by the Australian Research Council, Discovery Grant program (DP180103337).
Data availability
The raw data supporting the conclusions of this article will be made available by the authors on reasonable request.