-
PDF
- Split View
-
Views
-
Cite
Cite
Nathan van Wyk, Dorte Fischer, Derik Wilbers, Susan T L Harrison, Athanasios Kotsiopoulos, Mark Dopson, Toward the bioleaching of bauxite residue by Gluconobacter oxydans, Journal of Applied Microbiology, Volume 135, Issue 11, November 2024, lxae279, https://doi.org/10.1093/jambio/lxae279
- Share Icon Share
Abstract
This project evaluated a biologically mediated strategy to solubilize several rare earth elements and critical raw materials, including scandium, from bauxite residue. This work seeks to expand on previous research on contact leaching with bauxite.
In this study, Gluconobacter oxydans was shown to secrete mixed organic acids, including gluconic acid, which was superior to pure gluconic acid in the dissolution of bauxite residue, even at low molarities. In situ contact leaching with G. oxydans significantly promoted the dissolution yield (recovery of metal present in the ore) of yttrium, aluminum, calcium, and titanium (41.18%, 67.79%, 80.16%, and 59.41%, respectively) but allowed for only marginal dissolution yield of scandium, lanthanum, cerium, and neodymium (13.40%, 14.74%, 24.41%, and 10.67%, respectively) at relatively low pulp densities. In addition, the dissolution yields of rare earth elements were reduced further with time, presumably as the oxides of these elements fell out of solution.
This work builds on previous research that seeks to extract rare earth elements and critical raw materials from bauxite residue through contact leaching with organic acids. Some elements such as yttrium, aluminum, calcium, and titanium could be effectively solubilized; however some elements showed reduced solubility, possibly due to tight association with the iron phase of the residue. However, the relative ease and speed of leaching, and improved solubilization, suggest that this could be a viable method for securing critical raw material supplies.
The biogenic acid-mediated dissolution of economically valuable metals from metal-containing wastes and ores presents an attractive, yet currently unrealized technology. Gluconobacter oxydans rapidly produces gluconic acid, which is effective in the dissolution of several metals from bauxite residue. Despite low molarities of biogenic gluconic acid proving more effective than other common organic and mineral acids in the dissolution of bauxite residue, relatively low yields of economically valuable metals remain low.
Introduction
With rapidly expanding global electrification through low-carbon technologies, a growing population, and industrialization practices moving toward net zero comes a concomitant increase in demand for critical raw materials (CRMs) and rare earth elements (REEs) (Jowitt 2018, Jowitt et al. 2018, Kalt et al. 2022, Grohol et al. 2023). As defined and updated by the European Commission, CRMs and REEs are industry-critical materials at risk of supply disruption and/or of high economic importance (Grohol et al. 2023).
Uneven global distribution of CRMs and REEs as ores or recyclable materials contributes to supply uncertainty whereby ores are predominantly mined and processed in developing countries, while developed countries consume the bulk of these manufactured goods (Jowitt 2018, Abhilash et al. 2021). The implication is that energy consumption and pollution resulting from mineral processing are restricted to the regions of origin and the valuable end-materials to the regions of consumption. An aversion to health hazards, pollution, and strong environmental laws in many developed countries has allowed geopolitical entities to have near-monopolies on REE supply (Barnett et al. 2020). Only an estimated 1% of REEs are recycled from end-of-life products currently, with the remainder considered as waste, and so both contributing to the ongoing pollution and thus being removed from the materials cycle altogether (Jowitt et al. 2018). Currently, the growing global demand for REEs must predominantly be supplied through the processing of primary sources.
Bauxite is the primary source of alumina (Barnett et al. 2020). During the Bayer process, milled bauxite ore is digested in a pressure vessel with a concentrated sodium hydroxide solution at up to 270°C. The resultant sodium aluminate is filtered from the solid fraction and further refined to alumina (Hind et al. 1999). An estimated 2–4 tonnes of bauxite is required for every tonne of alumina produced, with the generation of 1–2.5 tonnes of residue, depending on the grade and quality of the bauxite ore (Hind et al. 1999, Liu and Naidu 2014, Panda et al. 2021, Tanvar and Mishra 2022). The solid fraction—often referred to as bauxite residue, or red mud—is highly caustic with a pH of 10–12 and may contain elevated concentrations of radioactive elements (Borra et al. 2015, Onghena et al. 2017). The global bauxite residue stockpile was estimated at 4 billion tonnes in 2018, with an annual contribution of >140 million tonnes. This presents a hazard to the environment and human health (Borra et al. 2015, 2017, Rivera et al. 2018, Kiskira et al. 2020, Tanvar and Mishra 2022). Bauxite residue is primarily disposed of in clay-lined dams into which the bauxite residue slurry is pumped, or dewatered and dried to decrease environmental risks and lower disposal costs (Hind et al. 1999, Qu and Lian 2013, Ujaczki et al. 2018). The disposal and stockpiling of bauxite residue constitutes a significant proportion of the overall production cost of alumina—disposal requires large tracts of land, ∼1 km2 every 5 years for a 1 Mt year−1 alumina plant (Hind et al. 1999, Tanvar and Mishra 2022).
Less than 4% of bauxite residue is reported to be utilized in downstream processes, predominantly as an aggregate or additive in the construction industry or in the production of ceramics (Ujaczki et al. 2018, Tanvar and Mishra 2022). However, the presence of empirically valuable elements, including iron (Fe), titanium (Ti), aluminium (Al), vanadium (V), and scandium (Sc) plus other REEs and CRMs in bauxite residue makes it a potentially lucrative target for metal recovery (Tanvar and Mishra 2022), prior to repurposing the resultant residual waste for the bulk uses proposed. Several studies have investigated the extraction of valuable elements from bauxite through processes mediated abiotically and biotically by subsequent valorization of the bauxite residue. Abiotic extraction processes usually rely on pyro- and hydrometallurgical processes for the extraction of metals (Borra et al. 2015, Davris et al. 2016, Borra et al. 2016b, 2017, Onghena et al. 2017, Ujaczki et al. 2017, Rivera et al. 2018, Tanvar and Mishra 2022). However, bioleaching technology using microorganisms for the extraction of valuable metals from iron oxide ores is gaining attention since it has the potential to be, comparatively, greener and more energy-efficient process (Liu and Li 2015, Pathak et al. 2021). Several biotic methods for the solubilization of REEs and CRMs from bauxite residue have been proposed, relying on the production of organic acid/s with concomitant leaching of metals (Vachon et al. 1994, Qu and Lian 2013, Urík et al. 2015, Qu et al. 2019a,b, Barnett et al. 2020, Abhilash et al. 2021, Kiskira et al. 2023). The biotic production of acids for bioleaching is an attractive proposition depending on the acid and organism as (i) the cost of producing industrially feasible concentrations of acids may be significantly lower than purchasing them from vendors; (ii) the acid may be produced using a secondary waste stream as feed, thus reducing the cost of production; and (iii) the biotically produced blend of acids may be superior at leaching REEs and CRMs than a pure acid alone (Reed et al. 2016, Qu et al. 2019a, Kiskira et al. 2020). Leaching of bauxite residue has been demonstrated using a variety of acids with efficient leaching favoring strong mineral acids in the order HNO3 > HCl > H2SO3 > H2SO4 > H3PO4. However, it has also been shown that biotically produced organic acids show enhanced solubilization and leaching of metals from bauxite residue (Ujaczki et al. 2019, Abhilash et al. 2021, Panda et al. 2021, Kiskira et al. 2023).
REEs appear to be present in bauxite as mineral phases, or as ions that are absorbed to the surface of minerals or substituting similar ions in the lattice of the mineral matrix (Borra et al. 2015). Sc, a high value REE, accounts for >95% of the total value of the REEs present in bauxite residue (Borra et al. 2016a). Sc appears to be associated with the iron (III) oxide lattice that is insoluble in acid solutions unless the iron is completely dissolved (Borra et al. 2015, 2016a, 2017). Gluconic acid, an organic acid, effectively leaches REEs from complex, REE-containing, recycling materials as well as iron oxide-rich bauxite (Reed et al. 2016, Abhilash et al. 2021). This study seeks to explore the use of a biotic organic acid in the dissolution of bauxite waste for the release of valuable elements, while going on to explore the viability of the microorganisms used in this contact leaching system.
Gluconobacter oxydans is a Gram-negative gluconic acid-producing chemoorganotrophic bacterium (Gupta et al. 2001, Elfari et al. 2005). Gluconic acid has previously been shown to effectively leach REEs from bauxite, while presenting a lower hazard (Barnett et al. 2020, Abhilash et al. 2021). Here, the solubility of a Greek bauxite residue in various mineral and organic acids, including spent G. oxydans media, was investigated. Other parameters, including growth kinetics in the presence of bauxite residue, gluconic acid production, and leaching of REEs were investigated.
Materials and methods
Characterization of the bauxite residue
Bauxite residue was received courtesy of Mytilineos S.A., Greece. The residue was washed twice in 3× volumes of distilled water and dried in an aluminum tray at 50°C for ∼2 days, or until no sign of moisture shown. The bauxite residue was broken into smaller lumps by hand, pulverized with a ceramic pestle, and passed through a 1-mm test sieve. The residue was then milled for 2 min at 100 rpm in a planetary ball milling machine (Retsch® S1).
Inductively coupled plasma-optical emission spectrometry (ICP-OES) chemical analysis of samples was carried out using an Agilent 5800. Briefly, the sample was acid digested (65%, HNO3), passed through a 0.2-µm filter, and appropriately diluted using 2% HNO3 (m/m) before analysis. X-ray diffraction (XRD) data were collected using a Bruker D8 ADVANCE diffractometer equipped with a position-sensitive detector and a cobalt (Co) anode as an X-ray source (λ Kα1 = 1.78897 Å; 35 kV and 40 mA). XRD patterns were recorded in the 2θ angle range of 5°–70° with a step size of 0.01805° and 1.5 s per step. Data processing was done using the TOPAS software suite.
Microbial strains and culture conditions
Gluconobacter oxydans (DSMZ 46616, ATCC 9844) was purchased from the Leibniz Institute DSMZ (German Collection of Microorganisms and Cell Cultures, GmbH). G. oxydans was cultured and maintained on a solid medium comprising no. 5 yeast extract medium (Krajewski et al. 2010), pH 6.8, and 20 g l−1 precipitated CaCO3 with 15 g l−1 bacteriological agar. G. oxydans was cultured in modified medium no. 5 (Krajewski et al. 2010), with pH amended to 6.8, but excluding precipitated CaCO3. The cultures were grown in Erlenmeyer flasks sealed with foil caps, incubated at 30°C, and shaken at 120 rpm. The culture pH was monitored to estimate maximal gluconic acid production. Cell-free media were created by centrifuging and filtering (10 000 × g, 0.44 µm) the G. oxydans media when the pH attained a value of 2.5.
Determination of gluconic acid concentrations
A d-gluconate (d-gluconic acid) colorimetric assay kit (Sigma–Aldrich, MAK279) was used to determine the gluconic acid concentration in biotically generated spent media solutions. Samples were analyzed as per manufacturer’s instructions; briefly, samples were treated by filtration through a 10 kDa molecular weight cut-off spin filter (Piercetm Concentrator, PES, Thermo Scientific). The samples were then treated with polyvinylpolypyrrolidone [PVPP, 1% (w/v)] by mixing for 5 min at room temperature. The samples were centrifuged at 10 000 × g for 5 min, the supernatant collected, and neutralized with a 1:1 dilution with 0.5 mol l−1 Tris–HCl, pH 8.0. The absorbance of the samples was read on a plate reader (FLUOstar Omega, BMG Labtech) at 450 nm (A450) and the gluconic acid concentration determined against a standard curve concentration range as defined in the kit.
Abiotic and biotic acid dissolution
The performance of selected acids was compared by creating equinormal solutions (0.5 mol l−1) from commercial laboratory chemicals, except for the G. oxydans spent medium that was prepared as previously described (pH 2.7, 0.12 M). The dissolution of the bauxite residue [0.5% (m/v)] was tested against water (as a control), spent G. oxydans media, and a selection of mineral (HCl, HNO3, and H2SO4) and organic acids (acetic acid, citric acid, gluconic acid, and oxalic acid) at 30°C, 120× rpm for 7 days. The dissolution rate was tested gravimetrically by filtering the contents of the reaction flask, washing all residues with water, drying the pre-weighed filters at 65°C, and weighing after drying overnight. All samples were analyzed in triplicate and results presented as means ± SD.
Determination of solubilized elements
The dissolution of selected elements was measured by aspirating 50 ml of the reaction volume, filtering (0.22 µm; nitrocellulose), and analysing by inductively coupled plasma mass spectrometry (ICP-MS) as performed by SAI, University of A Coruña, Spain. The yield was reported as the mass of solubilized metal expressed as a percentage of total metal in the bauxite residue (equation 1)
Dissolution of increasing pulp densities of bauxite residue in fresh and spent G. oxydans media
Sterilized bauxite residue at increasing pulp densities (0.1%, 0.5%, and 1.0%) was incubated in cell-free spent G. oxydans media (0.1 mol l−1 gluconic acid) for 7 days to determine the dissolution as a result of the interaction of gluconic acid and increasing rates of extremely alkaline residue. In addition, two control samples with pulp densities at either side of the range investigated (0.1% and 2.5%) were incubated in fresh media. All samples were in triplicate, incubated at 30°C and 120 rpm, and contained the antibiotic cefoxitin (Thermo Scientific) at 50 µg ml−1, to which G. oxydans possesses natural resistance, to suppress bacterial growth. As bauxite residue is an extensively oxidized waste, sterilization by autoclaving at 121°C for 20 min was considered to not alter the mineralogy significantly. The unsolubilized bauxite residue was collected on pre-weighed filters, dried, and weighed. The extent of dissolution was determined as a percentage of the original added bauxite residue.
Contact batch leaching of bauxite residue to determine leaching of selected elements
Bauxite residue pulp (0.5%) was added to 450 ml fresh medium no. 5 (Krajewski et al. 2010) modified to exclude CaCO3 but included cefoxitin (Thermo Scientific) at 50 µg ml−1. To this, a 10% volume inoculum of a fresh G. oxydans culture was added, while the same volume of fresh media was added to control samples. The cultures were allowed to grow for a total of 14 days at 30°C and 120× rpm. Samples were taken at 0, 3, 7, and 14 days and centrifuged at 10 000 × g, and the supernatant filtered through a 0.22-µm filter. The filtrate was analyzed by ICP-MS and the dissolution yield of Sc, yttrium (Y), lanthanum (La), cerium (Ce), neodymium (Nd), Al, Ca, Fe, and Ti determined as the percentage of solubilized elements to the total determined in the bauxite residue.
Contact batch leaching of bauxite and use of adapted cells
Sterilized bauxite residue pulp (0.5%, 1%, 2%, and 5% pulp density in triplicate, with each condition including a cell-free control) was added to 450 ml fresh medium no. 5 (Krajewski et al. 2010) modified to exclude CaCO3, but including cefoxitin (Thermo Scientific) at 250 µg ml−1. To this a 10% volume inoculum of a fresh G. oxydans culture was added. A representative, well-mixed sample was drawn and analyzed on days 0, 1, 2, 3, 5, 7, and 9, and pH, redox potential (eH), total solubilized iron (Fe), and cell number were recorded. At the conclusion of the experiment, a 10% inoculum was used to initiate a new leach with identical parameters, but without controls. Leaching of elements of interest was not recorded due to transfer between the successive experiments; however, pH, redox potential and cell number were recorded. The experiment was terminated after 2 days.
Viability determination
Petri dishes with solid media [medium no. 5, including precipitated CaCO3 (20 g l−1), cefoxitin (50 µg ml−1), and bacteriological agar (15 g l−1)] were plated (in triplicate) with 100 µl (of a 1000× dilution) of media from a bauxite-containing (0.1% bauxite residue, n = 3) and a control (no added bauxite residue, n = 3) culture. The cultures consisted of medium no. 5 [without CaCO3 and bacteriological agar but included cefoxitin (50 µg ml−1)], 10% (v/v) of a fresh G. oxydans inoculum, and with or without the bauxite residue. The cultures were sampled, appropriately diluted, and plated immediately after bauxite addition, as well as on days 1, 2, 3, 5, and 7. The plates were incubated at 30°C until clearing halos from gluconic acid-producing colonies were observed. The colonies were counted and colony forming units (CFUs ml−1) calculated.
Results
Elemental and mineralogical analysis of the bauxite residue
The bauxite residue had a heterogenous nature, and analyses (Table 1) showed that the mineralogy was dominated by hematite and cancrinite with lesser contributions of diaspore, rhodonite, and kimzeyite. The main aluminum-contributing minerals found in bauxite ore (diaspore and gibbsite) were depleted during the Bayer process. However, significant concentrations of aluminum (Table 2) persisted in the residue. Analysis of REEs in the residue indicated that significant concentrations of Y, Sc, La, Ce, and Nd were present within the residue matrix (Table 3).
Mineral . | % Weight . | Mineralogical formulae . |
---|---|---|
Brucite | 1.36 | Mg(OH)2 |
Magnesite | 4.82 | MgCO3 |
Aragonite/calcite | 4.01 | CaCO3 |
Phlogopite | 2.99 | KMg3AlSi3O10(F,OH)2 |
Hematite | 34.57 | Fe2O3 |
Anatase/Rutile | 0.46 | TiO2 |
Ilmenite | 1.85 | (Fe,Ti)2O3 |
Kaolinite | 0.28 | Al2Si2O5(OH)4 |
Goethite | 4.15 | FeO(OH) |
Diaspore | 7.45 | AlO(OH) |
Gibbsite | 1.03 | Al(OH)3 |
Cancrinite | 15.55 | Na6Ca2[(CO3)2|Al6Si6O24]·2H2O |
Rhodonite | 7.71 | (Mn2+, Fe2+, Mg, Ca)SiO3 |
Kimzeyite | 7.78 | Ca3(Zr,Ti)2(Si,Al,Fe3+)3O12 |
Illite | 1.00 | (K,H3O) (Al,Mg,Fe)2(Si,Al)4O10[(OH)2,(H2O)] |
Quartz | 0.35 | SiO2 |
Dolomite | 0.69 | CaMg(CO3)2 |
Alunite | 0.29 | KAl3(SO4)2(OH)6 |
Corundum | 0.18 | Al2O3 |
Millerite | 0.17 | NiS |
Sillimanite | 0.01 | Al2SiO5 |
Montmorillonite | 0.02 | (Na,Ca)0.33(Al,Mg)2(Si4O10) (OH)2·nH2O |
Periclase | 0.28 | MgO |
Total | 97.00 |
Mineral . | % Weight . | Mineralogical formulae . |
---|---|---|
Brucite | 1.36 | Mg(OH)2 |
Magnesite | 4.82 | MgCO3 |
Aragonite/calcite | 4.01 | CaCO3 |
Phlogopite | 2.99 | KMg3AlSi3O10(F,OH)2 |
Hematite | 34.57 | Fe2O3 |
Anatase/Rutile | 0.46 | TiO2 |
Ilmenite | 1.85 | (Fe,Ti)2O3 |
Kaolinite | 0.28 | Al2Si2O5(OH)4 |
Goethite | 4.15 | FeO(OH) |
Diaspore | 7.45 | AlO(OH) |
Gibbsite | 1.03 | Al(OH)3 |
Cancrinite | 15.55 | Na6Ca2[(CO3)2|Al6Si6O24]·2H2O |
Rhodonite | 7.71 | (Mn2+, Fe2+, Mg, Ca)SiO3 |
Kimzeyite | 7.78 | Ca3(Zr,Ti)2(Si,Al,Fe3+)3O12 |
Illite | 1.00 | (K,H3O) (Al,Mg,Fe)2(Si,Al)4O10[(OH)2,(H2O)] |
Quartz | 0.35 | SiO2 |
Dolomite | 0.69 | CaMg(CO3)2 |
Alunite | 0.29 | KAl3(SO4)2(OH)6 |
Corundum | 0.18 | Al2O3 |
Millerite | 0.17 | NiS |
Sillimanite | 0.01 | Al2SiO5 |
Montmorillonite | 0.02 | (Na,Ca)0.33(Al,Mg)2(Si4O10) (OH)2·nH2O |
Periclase | 0.28 | MgO |
Total | 97.00 |
Mineral . | % Weight . | Mineralogical formulae . |
---|---|---|
Brucite | 1.36 | Mg(OH)2 |
Magnesite | 4.82 | MgCO3 |
Aragonite/calcite | 4.01 | CaCO3 |
Phlogopite | 2.99 | KMg3AlSi3O10(F,OH)2 |
Hematite | 34.57 | Fe2O3 |
Anatase/Rutile | 0.46 | TiO2 |
Ilmenite | 1.85 | (Fe,Ti)2O3 |
Kaolinite | 0.28 | Al2Si2O5(OH)4 |
Goethite | 4.15 | FeO(OH) |
Diaspore | 7.45 | AlO(OH) |
Gibbsite | 1.03 | Al(OH)3 |
Cancrinite | 15.55 | Na6Ca2[(CO3)2|Al6Si6O24]·2H2O |
Rhodonite | 7.71 | (Mn2+, Fe2+, Mg, Ca)SiO3 |
Kimzeyite | 7.78 | Ca3(Zr,Ti)2(Si,Al,Fe3+)3O12 |
Illite | 1.00 | (K,H3O) (Al,Mg,Fe)2(Si,Al)4O10[(OH)2,(H2O)] |
Quartz | 0.35 | SiO2 |
Dolomite | 0.69 | CaMg(CO3)2 |
Alunite | 0.29 | KAl3(SO4)2(OH)6 |
Corundum | 0.18 | Al2O3 |
Millerite | 0.17 | NiS |
Sillimanite | 0.01 | Al2SiO5 |
Montmorillonite | 0.02 | (Na,Ca)0.33(Al,Mg)2(Si4O10) (OH)2·nH2O |
Periclase | 0.28 | MgO |
Total | 97.00 |
Mineral . | % Weight . | Mineralogical formulae . |
---|---|---|
Brucite | 1.36 | Mg(OH)2 |
Magnesite | 4.82 | MgCO3 |
Aragonite/calcite | 4.01 | CaCO3 |
Phlogopite | 2.99 | KMg3AlSi3O10(F,OH)2 |
Hematite | 34.57 | Fe2O3 |
Anatase/Rutile | 0.46 | TiO2 |
Ilmenite | 1.85 | (Fe,Ti)2O3 |
Kaolinite | 0.28 | Al2Si2O5(OH)4 |
Goethite | 4.15 | FeO(OH) |
Diaspore | 7.45 | AlO(OH) |
Gibbsite | 1.03 | Al(OH)3 |
Cancrinite | 15.55 | Na6Ca2[(CO3)2|Al6Si6O24]·2H2O |
Rhodonite | 7.71 | (Mn2+, Fe2+, Mg, Ca)SiO3 |
Kimzeyite | 7.78 | Ca3(Zr,Ti)2(Si,Al,Fe3+)3O12 |
Illite | 1.00 | (K,H3O) (Al,Mg,Fe)2(Si,Al)4O10[(OH)2,(H2O)] |
Quartz | 0.35 | SiO2 |
Dolomite | 0.69 | CaMg(CO3)2 |
Alunite | 0.29 | KAl3(SO4)2(OH)6 |
Corundum | 0.18 | Al2O3 |
Millerite | 0.17 | NiS |
Sillimanite | 0.01 | Al2SiO5 |
Montmorillonite | 0.02 | (Na,Ca)0.33(Al,Mg)2(Si4O10) (OH)2·nH2O |
Periclase | 0.28 | MgO |
Total | 97.00 |
Element . | % Weight Bauxite residue . |
---|---|
Al | 8.77 |
Ca | 6.52 |
Co | 0.005 |
Fe | 24.75 |
Mg | 0.13 |
Si | 5.66 |
Ti | 2.83 |
Zn | 0.009 |
Total | 48.67 |
Element . | % Weight Bauxite residue . |
---|---|
Al | 8.77 |
Ca | 6.52 |
Co | 0.005 |
Fe | 24.75 |
Mg | 0.13 |
Si | 5.66 |
Ti | 2.83 |
Zn | 0.009 |
Total | 48.67 |
Element . | % Weight Bauxite residue . |
---|---|
Al | 8.77 |
Ca | 6.52 |
Co | 0.005 |
Fe | 24.75 |
Mg | 0.13 |
Si | 5.66 |
Ti | 2.83 |
Zn | 0.009 |
Total | 48.67 |
Element . | % Weight Bauxite residue . |
---|---|
Al | 8.77 |
Ca | 6.52 |
Co | 0.005 |
Fe | 24.75 |
Mg | 0.13 |
Si | 5.66 |
Ti | 2.83 |
Zn | 0.009 |
Total | 48.67 |
Element . | PPM (mg kg−1) Bauxite residue . |
---|---|
Y | 81.9 |
Sc | 99.0 |
La | 84.4 |
Ce | 306.9 |
Nd | 85.9 |
Element . | PPM (mg kg−1) Bauxite residue . |
---|---|
Y | 81.9 |
Sc | 99.0 |
La | 84.4 |
Ce | 306.9 |
Nd | 85.9 |
Element . | PPM (mg kg−1) Bauxite residue . |
---|---|
Y | 81.9 |
Sc | 99.0 |
La | 84.4 |
Ce | 306.9 |
Nd | 85.9 |
Element . | PPM (mg kg−1) Bauxite residue . |
---|---|
Y | 81.9 |
Sc | 99.0 |
La | 84.4 |
Ce | 306.9 |
Nd | 85.9 |
Abiotic acid and spent medium dissolution of bauxite residue
Dissolution of the bauxite residue with equinormal 0.5 mol l−1 solutions of a range of abiotic-origin mineral (HCl, HNO3, and H2SO4) and organic (acetic, citric, gluconic, and oxalic) acids showed with the exception of oxalic acid similar dissolutions resulting in ∼35%–43% dissolution of bauxite residue (Fig. 1). Oxalic acid performed better, solubilizing 57.37% (SD = 0.01) of the bauxite residue versus spent G. oxydans media with 71.22% (SD = 0.04) solubilization (paired, two-tailed student’s t-test, P = 0.06). The gluconic acid produced by G. oxydans (spent G. oxydans media) contained 0.12 N gluconic acid and resulted in significantly improved dissolution of bauxite residue over abiotic gluconic acid, with 71.22% (SD = 0.04) and 37.39% (SD = 0.02; P = 0.033) solubilization, respectively.
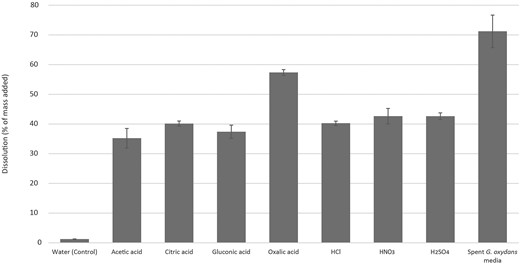
Dissolution of bauxite residue 0.5% (m/v) with abiotic and biotic acids. Dissolution was conducted using acetic acid, citric acid, gluconic acid, oxalic acid, hydrochloric acid, nitric acid, and sulfuric acid along with spent G. oxydans medium and water as control. All acids were an equinormal solution at 0.5 mol l−1 except the spent G. oxydans medium (0.12 mol l−1 gluconic acid). Data points are averages of replicates (n = 3) ± SE. SE for water (control), acetic acid, citric acid, gluconic acid, oxalic acid, hydrochloric acid, nitric acid, and sulfuric acid were 0.04, 3.29, 0.87, 2.21, 1.00, 0.72, 2.60, and 1.13, respectively. SE for the spent G. oxydans media was 5.49.
Gluconic acid generation by G. oxydans
Evolution of gluconic acid in a G. oxydans culture (technical replicate; n = 3) with glucose (0.28 mol l−1) as a feed resulted in variable gluconic acid synthesis, usually between 0.1 and 0.25 mol l−1; however, the profile was conserved.
After 10% (v/v) inoculation with a 24-h old culture on day 0, the concentration of gluconic acid rapidly increased over 24 h, followed by a plateau, and decreased from day 2. The decrease in gluconic acid concentration occurred at the same time as a browning of the media, most likely the oxidation of gluconic acid to 2-ketogluconic acid and 2,5-diketogluconic acid (Gupta et al. 2001). The addition of 0.1% (m/v) bauxite residue to a freshly inoculated G. oxydans culture (Fig. 2) resulted in a slowed evolution of gluconic acid and associated drop in pH when compared to the control. The P-values for gluconic acid concentration and pH in cultures containing 0.1% bauxite and no bauxite were only significantly different on day 1 (P = 0.006 and P = 0.01, respectively). The bauxite residue-containing samples attained numerically similar gluconic acid concentration and pH on days 2 and 3, respectively.
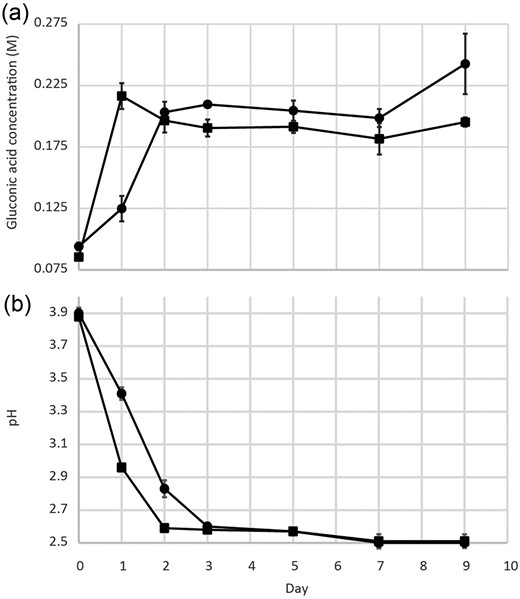
Gluconic acid production (a) and pH trace (b) of a G. oxydans culture with (circle) and without (square) contacting 0.1% pulp density bauxite residue. P-values for gluconic acid concentration and pH were only significant on day 1 (P = 0.006 and P = 0.01, respectively). Data points are averages of replicates (n = 3) ± SE.
Bioleaching of bauxite residue with G. oxydans spent media
The addition of increasing concentrations [0.1%, 0.5%, and 1.0% (m/v)] of bauxite residue to cell-free G. oxydans spent media solution (0.1 mol l−1 gluconic acid) was used to determine the solubilization capacity of a standard concentration of biotic gluconic acid (Fig. 3). Controls (fresh medium no. 5) at different pulp densities [0.1% and 2.5% (m/v)] were used to determine whether the media itself was able to drive solubilization of bauxite residue. Solubilization of bauxite residue in the control samples resulted in 22.77% (0.1% bauxite) and −0.5% (2.5% bauxite) dissolution. Bauxite residue at higher pulp densities leached with spent media [0.5% and 1.0% (m/v)] were not significantly different from each other (P = 0.25) and resulted in 40.79% and 39.63% dissolution, respectively. A bauxite residue pulp density of 0.1% resulted in 55.47% dissolution in spent media and was significantly different from the other conditions (0.5% and 1.0%; P = 0.015 and 0.022, respectively). In addition, the dissolution bauxite residue with spent medium was significantly greater than that of the cell-free control (P = 0.015). These data indicated that while medium alone was able to solubilize some fraction of the bauxite residue (Figs 1 and 3), the spent media resulted in a significantly higher dissolution.
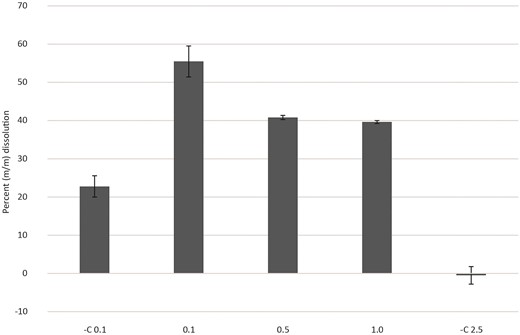
Increasing concentrations (0.1%, 0.5%, and 1.0%) of bauxite residue leached in a cell-free G. oxydans spent medium solution (0.1 mol l−1 gluconic acid). Controls (fresh medium, medium no. 5) were performed with pulp densities of 0.1% and 2.5%. The controls were not significantly different (P = 0.13) from each other. Pulp densities (0.5% and 1.0%) of bauxite residue were not significantly different (P = 0.25). Data points are averages of replicates (n = 3) ± SE.
G. oxydans contact bioleaching of bauxite residue
The extent to which an active G. oxydans culture leached bauxite residue [n = 1, 0.5% (m/v)] was determined by comparing metals leaching in cell-free spent media (Fig. 4a and b) and a culture inoculated with 10% (v/v) G. oxydans (Fig. 4c and d). Calcium was readily released from the bauxite residue in the cell-free control, attaining a concentration in solution of 134 mg l−1 on day 3, but largely plateauing after that, reaching a maximum concentration of 150 mg l−1. All other analyzed metals largely remained within the mineral matrix. The mobility of metals increased when the bauxite residue was incubated in contact with G. oxydans. Calcium reached a maximum of 289 mg l−1 on day 3, followed by a slow decrease over the remaining experimental period. Al and Ti were also solubilized to concentrations of 261 and 101 mg l−1, respectively, on day 14. Sc, Y, La, Ce, and Nd were leached into solution at concentrations of 271, 210, 164, 152, and 65 µg l−1, respectively (peak solubilizations in Table 4). All metals analyzed, other than Al and Ti, showed a peak dissolution on day 3, coinciding with peak gluconic acid concentrations. Also of note was that although Fe was the most abundant element within the bauxite residue matrix, little total Fe (2.27%) was leached into solution and was completely absent at the conclusion of the experiment.
![Bioleaching of bauxite residue pulp (0.5%) via contact with G. oxydans (c and d). Cell-free medium was used as a control (a and b). Samples taken on days 0, 3, 7, and 14 were analyzed by ICP-MS for selected elements (a and c: Al[♦], Ca[■], Fe[–], and Ti[●]; b and d: Sc[♦], Y[●], La[▲], Ce[■], and Nd[–]).](https://oup.silverchair-cdn.com/oup/backfile/Content_public/Journal/jambio/135/11/10.1093_jambio_lxae279/7/m_lxae279fig4.jpeg?Expires=1750240750&Signature=oVuEBqyMcrs2wlNk1BLwpj3NRrflhTb9pRk9R30jpYimyRf6KskQhW4oOZZkKyUTdNWqf9nsf3i81xycxc1qFN6mh6Fq0kAhagnDif9EClEhjaZeIxZ2ClCp6JUf5bO6R74v4m8iUeqS3LuPUjdADq6PFkFSJxrgD~xtxK8K9gyuRB1i-ELMygOs8QTPalO49WHHL9IYxbiU60Pewxq4AeYPNJN9ebi5cHZqOOHdd8dHUQ7qKSYlRje98zGKL7a905L8MEfmd3dckwuevKRH4-a9da5U39zqYEoRm59UG1toiS0fDgAPQ3WMzxPTCwe5M5Wu0edC-0UScnoxLPrGEw__&Key-Pair-Id=APKAIE5G5CRDK6RD3PGA)
Bioleaching of bauxite residue pulp (0.5%) via contact with G. oxydans (c and d). Cell-free medium was used as a control (a and b). Samples taken on days 0, 3, 7, and 14 were analyzed by ICP-MS for selected elements (a and c: Al[♦], Ca[■], Fe[–], and Ti[●]; b and d: Sc[♦], Y[●], La[▲], Ce[■], and Nd[–]).
Maximum yields (including the day when the maximum yields were attained) of selected elements solubilized from 0.5% pulp density bauxite residue when grown in contact with a G. oxydans culture.
. | Peak yield (day) . | Maximum yield (%) . |
---|---|---|
Sc | 3 | 13.40 |
Y | 3 | 41.18 |
La | 3 | 14.74 |
Ce | 3 | 24.41 |
Nd | 3 | 10.67 |
Al | 14 | 67.79 |
Ca | 3 | 80.16 |
Fe | 3 | 2.27 |
Ti | 14 | 59.41 |
. | Peak yield (day) . | Maximum yield (%) . |
---|---|---|
Sc | 3 | 13.40 |
Y | 3 | 41.18 |
La | 3 | 14.74 |
Ce | 3 | 24.41 |
Nd | 3 | 10.67 |
Al | 14 | 67.79 |
Ca | 3 | 80.16 |
Fe | 3 | 2.27 |
Ti | 14 | 59.41 |
Maximum yields (including the day when the maximum yields were attained) of selected elements solubilized from 0.5% pulp density bauxite residue when grown in contact with a G. oxydans culture.
. | Peak yield (day) . | Maximum yield (%) . |
---|---|---|
Sc | 3 | 13.40 |
Y | 3 | 41.18 |
La | 3 | 14.74 |
Ce | 3 | 24.41 |
Nd | 3 | 10.67 |
Al | 14 | 67.79 |
Ca | 3 | 80.16 |
Fe | 3 | 2.27 |
Ti | 14 | 59.41 |
. | Peak yield (day) . | Maximum yield (%) . |
---|---|---|
Sc | 3 | 13.40 |
Y | 3 | 41.18 |
La | 3 | 14.74 |
Ce | 3 | 24.41 |
Nd | 3 | 10.67 |
Al | 14 | 67.79 |
Ca | 3 | 80.16 |
Fe | 3 | 2.27 |
Ti | 14 | 59.41 |
Cell density, pH, and redox potential were recorded over 9 days in a G. oxydans culture with increasing bauxite residue concentrations [0.5%, 1.0%. 2.0%, and 5.0% (m/v)] and a cell-free medium control sample (Fig. 5). Metals concentrations were not analyzed as this had been recorded in a previous experiment (Fig. 4). Cell densities (Fig. 5a) at time zero were approximately proportional to pulp density—most likely due to indigenous microorganisms that could not be removed from the bauxite residue. In addition, pH values (Fig. 5c) were proportional to the relative densities of the caustic bauxite residue added to the condition, with the lowest pHs attained by conditions with the lowest bauxite additions. Additions of 0.5% and 1.0% bauxite residue resulted in a pH of 2.8 and 3.1 after 9 days, close to the lower growth limit of G. oxydans. The highest bauxite residue addition initially showed a drop in pH, followed by a transient rise, likely due to cytotoxicity of the leachate preventing gluconic acid production. As with pH, recorded redox potential (Fig. 5b) was proportional to the relative densities of bauxite residue added to the condition, with the highest pulp densities attaining a negative redox potential. The redox potential for all conditions initially decreased from day 0 to 3, followed by an increase until the conclusion of the experiment.
![Cell counts (a), redox potential (b), and pH (c) for a G. oxydans contact leach (n = 3) culture and a fresh medium control (n = 1) of bauxite residue at increasing pulp densities (control, 0.5% (♦), 0.5% (–), 1.0% [▲], 2.0% [■], and 5.0% [●]). Data points are averages of replicates (n = 3) ± SE.](https://oup.silverchair-cdn.com/oup/backfile/Content_public/Journal/jambio/135/11/10.1093_jambio_lxae279/7/m_lxae279fig5.jpeg?Expires=1750240750&Signature=P-f0Gw3LohObR4TReIlY8HpHBPEE3wDYA-1ojraudiM9GicTx11gyrz2npsT6ao5kMtc9gpd8ov-KJNVdt9qCHRiljZTVgcupXItadXKUFqidhfGVj6m3uC16Ji5HRCd7OKIeLC1uPscmEdJE07trgBLS5UHdmsOOLoYCzRUdrM0swN3tYG9t86buveKReZh85GcXY6MdC06Fj0ARJqZN8RYq5GboQYff~MhguQzWDMN~HrDLdmHZwnO2liiSaWNgFB3rQEyWfbIB9ElqxAmaXK8SIlTiFXYMPTjDUKcwE1Zd5TxVQviIB7G2QIxZsDZVOTiPlMAjPrQHnRCTO5rww__&Key-Pair-Id=APKAIE5G5CRDK6RD3PGA)
Cell counts (a), redox potential (b), and pH (c) for a G. oxydans contact leach (n = 3) culture and a fresh medium control (n = 1) of bauxite residue at increasing pulp densities (control, 0.5% (♦), 0.5% (–), 1.0% [▲], 2.0% [■], and 5.0% [●]). Data points are averages of replicates (n = 3) ± SE.
“Adapted” G. oxydans cells harvested from an inoculum cultured with 0.5% (m/v) bauxite residue were also used to inoculate cultures with 0.5%, 1.0%, 2.0%, and 5.0% (m/v) bauxite residue (Fig. 6). These adapted G. oxydans cells were unable to lower the pH or affect the redox potential of any condition as they were when using a fresh culture.
![Cell counts (a), redox potential (b), and pH (c) in an adapted G. oxydans contact leach (n = 3) culture and a fresh medium control (n = 1) of bauxite residue at increasing pulp densities [0.5% [■], 1.0% [▲], 2.0% (♦), and 5.0% [●]]. Data points are averages of replicates (n = 3) ± SE.](https://oup.silverchair-cdn.com/oup/backfile/Content_public/Journal/jambio/135/11/10.1093_jambio_lxae279/7/m_lxae279fig6.jpeg?Expires=1750240750&Signature=hauWGj3tYKYCQeO-wo-wE4CWqYX6zCzULsfB73-f0S7iTU9o8sjMjCRgnoAjt-mp4qLxPiozx-swl23~d8G8U-t2YFAi5vp~WhYsY1wHnGDpW7cqtIMtZjOaaB-~FyG5MGGf5XN19PtF1vlbsT7YsVxEOqGXTSVMYz69YbbdAhqArN13Dy13LfsItmz1kpl1L6qldKE~S~Jjw~jJ4vKGrGwFyBcb6TvEVBnphMPtc8QGpRMoD9FTOFA3C2slcoBIOqxLOE2tGzN~hwp~zQ9b5SXikzLOruhhXiLLPsOfWeZRKGWcXUaeLcS0AT2dyqMo1KWutLqrnxWC7h2nYpxQrA__&Key-Pair-Id=APKAIE5G5CRDK6RD3PGA)
Cell counts (a), redox potential (b), and pH (c) in an adapted G. oxydans contact leach (n = 3) culture and a fresh medium control (n = 1) of bauxite residue at increasing pulp densities [0.5% [■], 1.0% [▲], 2.0% (♦), and 5.0% [●]]. Data points are averages of replicates (n = 3) ± SE.
G. oxydans cell viability in the presence of bauxite residue
G. oxydans cells grown in contact with a 0.1% (m/v) bauxite residue and a control (without added bauxite residue) were plated onto solid media. CFUs were only significantly different on days 1 and 2 between the culture with G. oxydans in contact with bauxite residue and bauxite residue-free G. oxydans culture (P = 0.019 and 0.005, respectively). Up to 9000 CFUs ml−1 could be cultured on day 2 from the control condition, compared to 1000 CFUs ml−1 for the condition cultured in contact with bauxite residue. Culturable CFUs remained low (7 on day 3, 567 on day 5, and 3.33 on day 7) throughout the sampling period for G. oxydans cells cultured in contact with bauxite residue.
Discussion
This work is the second instance of contact leaching of bauxite with G. oxydans of which we are aware (Abhilash et al. 2021) and aimed to investigate the efficacy of organic acid leaching of Greek-origin bauxite residue. In this study, a maximum of 13.4% Sc and 59.41% Ti were solubilized after 3 days at a pulp density of 0.5%. The concentration of the solubilized Sc decreased until the completion of the experiment, while Ti gradually increased. Abhilash et al. recovered 83%–94% Sc after 18–20 days, Ti recovery rates were relatively low (<5%), and significant solubilization of metals were achieved at 20% pulp density, unlike this study where significant negative impacts to leaching efficiency at just 1%–2% pulp density were apparent. These differences were likely due to the specific mineralogy and pH of the different bauxite residues used in the two studies.
This study demonstrated good solubilization efficiency of some elements, semi-selective leaching against Fe, a suppressive effect of increased bauxite pulp density on the leaching capacity of gluconic acid, and a deleterious effect of increased bauxite pulp density on the cultivability of G. oxydans.
Leaching of bauxite residue with cell-free, biotic gluconic acid in spent G. oxydans media resulted in a significant dissolution advantage over other abiotic organic and mineral acids, even at a significantly lower molarity. It was likely that biotic gluconic acid contained several component organic acids that enhanced the leaching effect, despite a relatively high solution pH. This indicated that the conjugate base, and not necessarily increased hydronium ion availability, was an important determinant of leaching efficacy (Reed et al. 2016, Qu et al. 2019a, Kiskira et al. 2020, Schmitz et al. 2021). Rapid production (24 h) of gluconic acid by G. oxydans (Dai et al. 2022, 2023) also showed potential to overcome the problematic aspect of bioleaching that is slow acid generation rates. However, oxidation of gluconic acid to 2-ketogluconic acid and 2,5-diketogluconic acid, which is ineffective in leaching, presented a logistical challenge when utilizing biotic gluconic acid in the leaching of bauxite residue. This may be limited or avoided by utilizing fresh (≈24 h) spent media produced in a separate G. oxydans culture/reactor operating in a continuous manner, with the effluent feeding a bauxite residue leaching process.
Due to the present cost of reagents and the recent price of target metals, no industrial-scale heterotrophic bioleaching processes for the recovery of metals from wastes or ores have been implemented. However, future demand, coupled with increased recoveries/yield, could influence the balance of feasibility and profitability, and tip the scale toward the initiation of this or a similar industrialization process for the recovery of valuable metals. Present industrialized application of this work would likely necessitate biotic gluconic acid concentrations above those generated in biological systems (Rasoulnia et al. 2023). Increased concentrations of gluconic acid may be achieved through increasing the concentration of the evolved organic acids and application of bauxite residue, enhancing leaching. Alternatively, a two-step leaching system where biotic gluconic acid is synthesized and transferred to a separate leaching vessel, exposing the bauxite residue to a steady stream of fresh biotic gluconic acid, followed by extraction of the solubilized metals, is a method that may be explored. Finally, utilizing organic acid production by other microorganisms, such as Aspergillus niger, which is known to produce significant extracellular concentrations of oxalic acid (and which shows significant dissolution potential over gluconic acid), could increase overall yield.
Pulp density affected the efficacy of leaching with gluconic acid. Gluconic acid is converted to a gluconate anion at near-neutral pHs, requiring that leaching takes place at between pH 3 and 6 to preserve cell viability and gluconic acid leaching efficacy. High pulp densities were likely to exceed the narrow effective leaching pH range, and reasonable leaching densities are in the range of 0.1%–0.5% (m/v). The effect of pulp density in combination with metal toxicity has been encountered in other leaching studies with G. oxydans (Rasoulnia et al. 2023). A two-step process, where gluconic acid is continuously synthesized and then used to leach bauxite residue within a defined pH range, is likely a more effective strategy. In addition, a two-step process would allow higher pulp densities to be used—densities that would likely be toxic to G. oxydans, but not yet so alkaline as to form gluconate salts from gluconic acid.
Contact leaching of bauxite residue was effective at leaching Ca and Al, resulting in 80.16% and 67.79% yields, respectively. The semi-selective leaching of the bauxite residue allowed for the solubilization of only 2.27% of available Fe, all of which was removed from solution by the conclusion of the experiment by an unknown process. The 0.50%, 1.00%, and 2.0% pulp density conditions had a redox range of −14.3–69.3 mV on day 3 (day of peak dissolution for many elements) and a pH range of 3.22–3.91, which should result in solubilized Fe2+. This finding is in agreement with Abhilash et al. where low dissolution rates of Fe were recorded. Relatively low rates of REE solubilization and their removal from solution, most likely as oxides, were problematic but may be addressed in a continuous or semi-continuous system, where the pregnant leach solution is processed before the oxidation of the REEs. The low solubilization rates could not be explained using Pourbaix diagrams as all elements were theoretically soluble at the pH and redox potentials recorded in the solutions, especially at lower pulp densities [pH: 2.84–4.77; eH (mV): −101.3–218.7] (Brookins 1988). However, the previously reported high solubilization rates recorded for Sc (Abhilash et al. 2021) seemed to be not attainable, possibly due to the properties of bauxite residue used in the study, or due to Sc being associated with the Fe matrix in bauxite residue.
The “adaption” of G. oxydans cells appeared to be not a viable system for the effective leaching of bauxite residue. Relative to controls, cells exposed to bauxite residues appeared to have a limited ability to form colonies on solid media plates, indicating reduced viability, potentially from metal stress and cytotoxicity. G. oxydans sensitivity to heavy metals is documented (Rasoulnia et al. 2023, Yudina et al. 2023), but broader information on the effects of metals on G. oxydans viability is scarce.
This work builds on a previous study in the field and determines yields of various economically valuable elements within a closed system and with contact leaching of bauxite residue with G. oxydans. Refinements to the process, particularly using continuous or semi-continuous reactor systems, to reduce yield loss due to the oxidization of REEs by processing the solubilized metals before precipitation will shed light on the potential for the establishment of an industrial process for the solubilization of elements, and Sc in particular, from bauxite residue.
Acknowledgments
Mytilineos S.A. kindly provided the bauxite residue used in this study. This article does not contain any studies with human participants or animals performed by any of the authors.
Author contributions
Nathan van Wyk (Conceptualization, Data curation, Formal analysis, Investigation, Writing – original draft), Dorte Fischer (Data curation), Derik Wilbers (Data curation), Susan T. L. Harrison (Writing – review & editing), Athanasios Kotsiopoulos (Data curation, Writing – review & editing), and Mark Dopson (Conceptualization, Funding acquisition, Writing – review & editing)
Conflict of interest
None declared.
Funding
This project has received partial funding from the European Union’s Horizon 2020 research and innovation program under Grant agreement No. 821096.
Data availability
All data generated or analyzed during this study are included in this published article.